Open Access
REVIEW
Seismic Behavior of Squat Reinforced Concrete Shear Walls: A State-of-the-Art Review
1 Research Institute of Sciences and Engineering, University of Sharjah, Sharjah, P.O. Box 27272, United Arab Emirates
2 Department of Civil and Environmental Engineering, University of Sharjah, Sharjah, P.O. Box 27272, United Arab Emirates
3 Department of Civil Engineering, University of Ottawa, Ottawa, ON K1N 6N5, Canada
4 Department of Civil Engineering, Near East University, Nicosia, 99138, Cyprus
* Corresponding Author: Ahed Habib. Email:
Structural Durability & Health Monitoring 2025, 19(3), 417-439. https://doi.org/10.32604/sdhm.2025.059524
Received 10 October 2024; Accepted 18 December 2024; Issue published 03 April 2025
Abstract
Squat reinforced concrete (RC) shear walls are essential structural elements in low-rise buildings, valued for their high strength and stiffness. However, research on their seismic behavior remains limited, as most studies focus on tall, slender walls, which exhibit distinct failure mechanisms and deformation characteristics. This study addresses this gap by conducting an extensive review of existing research on the seismic performance of squat RC shear walls. Experimental studies, analytical models, and numerical simulations are examined to provide insights into key factors affecting wall behavior during seismic events, including material properties, wall geometry, reinforcement detailing, and loading conditions. The review aims to support safer design practices by identifying current knowledge gaps and offering guidance on areas needing further investigation. The findings are expected to aid researchers and practitioners in refining seismic design codes, ultimately contributing to the development of more resilient squat RC shear walls for earthquake-prone regions. This research underscores the importance of improving structural resilience to enhance the safety and durability of buildings.Keywords
Reinforced concrete (RC) shear walls play a fundamental role in the structural design of buildings, particularly in seismic regions [1,2]. These walls are critical for resisting lateral forces and enhancing the structural integrity and safety of buildings during seismic events [3,4]. Squat RC shear walls, with their low height-to-length ratio, are crucial in low-rise structures and buildings with limited vertical space. Their distinct structural characteristics necessitate a thorough understanding of their behavior under seismic loads to optimize their design and performance. The body of literature on the seismic behavior of squat RC shear walls is extensive, covering various aspects from experimental investigations to numerical simulations and analytical modeling. Choi [5] emphasized the significance of understanding the cyclic behavior of these walls to improve their seismic resilience. Li et al. [6,7] conducted cyclic tests on ultra-high-performance concrete (UHPC) squat shear walls, providing critical insights into their load-bearing capacity and deformation characteristics. Similarly, Chen et al. [8] explored enhancements in seismic behavior through innovative materials and construction techniques in one-sided concrete squat walls. In North America, El-Dakhakhni et al. [9] discussed the design of reinforced masonry and concrete walls, highlighting regional differences in design practices and their impact on wall behavior. Kim et al. [10] tested six squat walls under cyclic loading, contributing valuable data on failure mechanisms and deformation patterns. These findings align with those of Fathalla et al. [11], who also examined the seismic performance of squat walls under cyclic loads. The research by Akl et al. [12] further elaborate on collapse fragility functions for squat walls, while Arafa et al. [3] investigated the flexural and shear stiffness of these structures. Despite these efforts, existing literature lacks a review article that covers the experimental, analytical, and numerical studies of squat shear walls while also being systematic and bibliometrics. Accordingly, with the increasing frequency and intensity of earthquakes globally, there is an urgent need to enhance the understanding and design of these structural components. This study aims to conduct a comprehensive literature review on the seismic behavior of squat RC shear walls. By critical investigating findings from experimental, analytical, and numerical studies, the review will provide a detailed understanding of these walls’ performance during seismic events. The study will evaluate the effectiveness of current modeling techniques and propose recommendations for improving design practices and guidelines. The comprehensive scope of this study includes a detailed analysis of various aspects of wall behavior, modeling techniques, and design procedures. The review will also cover the general seismic performance of squat shear walls, discussing performance metrics and common failure mechanisms. Key experimental findings will be integrated to highlight the influence of structural and material parameters on wall behavior. Additionally, the study will examine analytical modeling approaches, numerical simulation methods, and the validation and comparison of these models against experimental data. The review will also provide a biometric assessment of the current state of the art and will go through the design strategies of squat shear walls. By addressing the existing fragmentation in the literature and providing a detailed perspective on wall behavior, this review aims to contribute to the development of more effective and reliable strategies for enhancing the seismic resilience of squat RC shear walls. The rest of the paper is organized as follows: Section 2 discusses the seismic behavior of squat shear walls; Section 3 reviews modeling techniques; Section 4 evaluates current design procedures; Section 5 summarizes key findings, identifies gaps, and suggests future research directions.
The bibliometric assessment of research on squat RC shear walls provides an insightful overview of scholarly activity in this specialized field. In order to perform the bibliometric assessment, a keyword search on the Scopus database identified about 110 directly related articles with squat shear walls mentioned in the title. These documents were then analyzed and reviewed in this study. Fig. 1 analyzes the publication trends over time and reveals a dynamic pattern in research output. The data shows periods of increased academic interest, particularly in recent years, which can be attributed to advancements in seismic design requirements and heightened awareness of structural resilience against natural disasters with respect to low-rise buildings. This trend underscores the growing importance of squat shear walls in the context of structural engineering and earthquake-resistant design.
Figure 1: Number of publications over time
A closer examination of the top journals, Fig. 2, reveals that the majority of influential studies are published in reputable sources such as Engineering Structures and ACI Structural Journal. The geographic distribution of research contributions, Fig. 3, highlights the global nature of scholarly work on squat shear walls. Leading countries, including the United States and China, are prominent due to their significant investments in infrastructure resilience and advanced research facilities. The presence of European nations like Germany and Italy also reflects their long-standing tradition in civil engineering research and innovation. This global distribution points to a collaborative international effort to enhance the understanding and performance of squat shear walls in seismic applications. The keyword analysis, Fig. 4, further elucidates the core themes and focal points within this body of research. The frequent occurrence of terms such as shear walls, RC, seismic behavior, and high-strength materials indicates a concentrated effort to understand the mechanical and seismic performance of squat shear walls. These keywords also suggest a strong emphasis on material innovations and the development of design methodologies that improve the resilience of structures in earthquake-prone areas.
Figure 2: Top 10 journals with the heights number of publications on squat shear walls
Figure 3: Countries with the highest number of publications on squat shear walls
Figure 4: Most used keywords in squat shear walls research
3 Seismic Behavior of Squat Shear Walls
3.1 General Seismic Performance
The seismic performance of squat RC shear walls is a vital area of study in structural engineering, particularly for buildings in earthquake-prone regions [12,13]. These walls are characterized by a low aspect ratio (height-to-length ratio less than two). As a result, they behave differently under seismic loading compared to their taller, slender counterparts [14,15]. Squat shear walls are less typically used in low-rise buildings and are less understood compared to taller ones, especially regarding their unique mechanisms of failure and deformation during earthquakes [16–18]. Unlike slender walls dominated by flexural deformations, squat walls are governed by shear deformations, making them more vulnerable to shear failures, which are often brittle and catastrophic if not properly addressed in the design [19,20]. The seismic performance of squat shear walls is influenced by several factors, including material properties, wall geometry, reinforcement detailing, and loading conditions [21,22]. Understanding these factors is crucial for enhancing the seismic resilience of buildings relying on squat shear walls for lateral load resistance [23,24]. Early research on squat shear walls, such as the study by Hidalgo et al. [14], emphasized the importance of reinforcement detailing in preventing shear failures and improving ductility under cyclic loading. Subsequent studies explored various reinforcement strategies to enhance seismic performance, including the addition of steel plates to improve energy dissipation and shear strength [8]. Recent research has expanded to include environmental factors affecting the seismic behavior of squat shear walls. For example, studies by Rong et al. [16] examined the impact of frost damage and exposure to offshore atmospheric environments on these walls, highlighting the need to consider environmental degradation in their seismic design. Similarly, Eid et al. [25] and Tong et al. [26] investigated the impact of construction materials, such as low-performance concrete (LPC) and UHPC, on the shear capacity and overall seismic performance of squat shear walls, finding that LPC can reduce seismic performance while UHPC can enhance it. Advanced analytical and numerical modeling techniques have also contributed to the understanding of squat shear wall behavior. Research by Sivaguru et al. [21] and Hosseini et al. [27] found that openings could significantly weaken the walls’ seismic resistance, emphasizing the need for careful reinforcement detailing around these openings. Environmental factors, such as chloride ion erosion and freeze-thaw cycles, also significantly affect the seismic performance of squat shear walls. Studies by Zheng et al. [23,28–30] and Yang et al. [31] demonstrated that environmental degradation could reduce the seismic capacity of these walls, underscoring the importance of incorporating such factors into the design and maintenance of squat shear walls. Similarly, chemical reactions within the concrete, such as alkali-silica reaction (ASR), can compromise the seismic resilience of these walls [32]. The role of boundary conditions and construction details in the seismic performance of squat shear walls has also been extensively studied. Research by Gulec et al. [15,33] provided insights into how boundary conditions influence shear strength and seismic performance. Different reinforcement materials have been explored for their impact on seismic performance as well. Studies by Yu et al. [18] and Lim et al. [34] found that steel fiber RC (SFRC) and strain-hardening cement composite (SHCC) can enhance shear strength and seismic resilience. The influence of wall geometry has also been a key area of research. Kim et al. [35] found that flanges can enhance seismic performance by providing additional lateral resistance. Yang et al. [36] emphasized the importance of considering geometric details in seismic design. Numerical simulations have become increasingly important in understanding the seismic behavior of squat shear walls. Tariq et al. [20] utilized gene expression programming to estimate the shear strength of RC squat walls, offering a novel approach to predictive modeling in seismic design. Akl et al. [12] conducted a seismic collapse risk assessment of low-aspect-ratio RC shear walls using FEMA P695 methodology, providing valuable insights into the probabilistic assessment of seismic performance. The integration of new materials, such as ultra-high-performance fiber-reinforced concrete (UHPFRC), has also been explored to enhance seismic performance. Nagib et al. [19,37] demonstrated that UHPFRC could significantly improve the seismic resistance and energy dissipation capacity of squat shear walls. Kang et al. [38] effect of cement matrix’s type on the shear performance of lightly reinforced squat shear walls subjected to cyclic loading. Finally, the role of the horizontal reinforcement ratio in improving the seismic performance of BFRP-RC squat shear walls was studied by Miao et al. [39]. Accordingly, the seismic performance of squat RC shear walls is influenced by various factors, Table 1, including material properties, wall geometry, reinforcement detailing, environmental conditions, and loading scenarios. While significant advancements have been made in understanding squat wall behavior, there remain gaps that require further research, particularly concerning the long-term effects of environmental degradation and performance under extreme loading conditions.
3.2 Failure Mechanisms and Modes
Over the past decades, experimental methods have been used as the main approach for investigating the behavior of civil structures [40,41]. In this regard, the failure mechanisms and modes of squat shear walls, which are critical aspects that significantly influence their seismic behavior, have been mainly investigated experimentally at the element level [10,24,31]. In general, squat shear walls, characterized by their relatively short height-to-length ratios, are prone to various failure modes, particularly under seismic loading conditions [42,43]. The failure mechanisms in these walls include shear failure, flexural-shear failure, shear sliding failure, flexural cracking, diagonal cracking, concrete crushing, brittle failure, and ductile failure [44,45]. Each of these mechanisms presents unique challenges that must be addressed to ensure the structural integrity and resilience of squat shear walls during seismic events. Shear failure is one of the most critical failure modes in squat shear walls, characterized by the formation of diagonal cracks and subsequent crushing of concrete, leading to sudden and brittle failure. This failure mode can be prevented by incorporating adequate shear reinforcement, which helps in distributing shear stresses and preventing the initiation and propagation of diagonal cracks [46,47]. As shown in Table 2, the implementation of appropriate shear reinforcement is essential to mitigate this type of failure and enhance the wall’s overall performance under seismic loads. Flexural-shear failure involves the simultaneous occurrence of flexural cracking at the wall’s base and shear cracking along its height, resulting in complex and unpredictable failure patterns. In order to prevent this failure mode, the use of high-strength concrete and well-detailed reinforcement is recommended.
These measures help control both flexural and shear cracks, thereby improving the wall’s resilience against seismic forces [31,43]. Shear sliding failure is another significant concern, where horizontal cracks develop along the plane of maximum shear stress, leading to substantial displacement and potential structural collapse. The incorporation of transverse reinforcement is a key preventive measure for this failure mode, as it helps prevent the propagation of horizontal cracks and subsequent shear sliding [48–50]. Flexural cracking, which primarily occurs at the base of the wall due to bending moments, can severely compromise the wall’s load-bearing capacity. Proper reinforcement detailing at the base is crucial to managing bending moments and preventing the onset of flexural cracking [31,50]. Additionally, diagonal cracking, caused by high shear stresses, poses a significant risk of shear failure if not adequately controlled. The strategic placement of reinforcement and optimization of concrete strength is necessary to control diagonal cracking and ensure the wall’s stability [4,51,52]. Concrete crushing is a localized failure mechanism that occurs at points of high compressive stress, compromising the structural integrity of the wall. Ensuring sufficient concrete cover and maintaining high concrete quality are essential preventive measures against this type of failure [49,53–56]. Therefore, understanding the various failure mechanisms and modes of squat shear walls is essential for improving their seismic performance. By implementing appropriate preventive measures, such as enhanced reinforcement detailing and the use of advanced materials, the resilience of these walls against seismic forces can be significantly improved, ultimately leading to safer and more durable structures.
3.3 Influence of Structural and Material Parameters
The seismic behavior of squat RC shear walls is a complex phenomenon influenced by various structural and material parameters. The influence of these parameters has been extensively studied to understand how they affect the overall performance of these walls during seismic events. Concrete strength is one of the most critical factors determining the seismic behavior of squat shear walls. Higher concrete strength increases the load-bearing capacity and reduces crack propagation during seismic events. Table 3 summarizes the influence of material properties on the seismic behavior of squat shear walls. It highlights that higher concrete strength, appropriate reinforcement types, and the addition of steel fibers contribute to improved seismic performance.
Moreover, reinforcement detailing plays a significant role in enhancing energy dissipation and controlling shear deformations, preventing brittle failures. Previously, Ganesan et al. [58] demonstrated that high-performance concrete with added steel fibers significantly enhances the first crack load, overall structural strength, and energy dissipation capacity under lateral cyclic loading. Similarly, UHPC squat shear walls exhibited superior performance in cyclic tests, with increased load-bearing capacity and reduced lateral deformations [6,7]. This aligns with findings from Chen et al. [59] and Chetchotisak et al. [60], who also highlighted the importance of concrete strength in seismic performance.
The application of high-strength concrete is further supported by the work of Liu et al. [43] where experimental and numerical investigations confirmed the enhanced seismic resilience of squat shear walls constructed with high-strength materials. The type of reinforcement used in squat shear walls plays a crucial role in influencing their seismic performance. Different types of reinforcement, such as steel, GFRP, and CFRP, affect the ductility and energy absorption capacity of the walls. Arafa et al. [3] emphasized that GFRP-reinforced squat walls demonstrated effective resistance to lateral loads, and their study highlighted the importance of considering concrete shear contribution and boundary element confinement for accurately predicting the ultimate flexural and shear strengths. Additionally, Woods et al. [46] found that CFRP sheets effectively restored the in-plane strength of earthquake-damaged RC shear walls, significantly enhancing their load-bearing capacity and overall seismic performance. These findings are consistent with those of Fathalla et al. [11], who observed that the type and detailing of reinforcement directly impact the energy dissipation and deformation characteristics of squat shear walls under seismic loads. Steel fibers, when added to concrete, further enhance the seismic performance of squat shear walls. Hosseini et al. [61] examined squat RC shear walls with steel and GFRP rebars. Testing six specimens, they found hybrid reinforcement improved seismic performance by modifying failure modes, enhancing energy dissipation, ductility, and load factors, and delivering superior hysteresis behavior compared to GFRP-only walls. Hybrid rebars proved effective in seismic applications.
Ganesan et al. [58] reported that steel fibers, at a volume fraction of 1% to 2%, increased the load-bearing capacity by approximately 25% and improved energy absorption by 30%. This improvement is attributed to the ability of steel fibers to delay the onset of shear cracking and enhance the ductility of the concrete, which is critical for preventing brittle failures during seismic events. Lim et al. [34] also supported these findings, noting that steel fibers contribute to the overall shear behavior of squat shear walls, particularly in configurations with vertical slits. Reinforcement detailing is another critical parameter that influences the seismic behavior of squat shear walls. Proper detailing, particularly the transverse reinforcement, is essential for controlling shear deformations and preventing brittle failures. Habibi et al. [32] found that a transverse reinforcement ratio of 0.5% to 1% was optimal for controlling shear deformations, thereby enhancing the energy dissipation capacity of the walls during seismic loading. This is consistent with the observations of Jin et al. [42] and Ma et al. [62], who emphasized the importance of reinforcement detailing in ensuring the structural integrity and seismic resilience of squat shear walls. The influence of structural parameters on the seismic performance of squat shear walls is further illustrated in Fig. 5, which shows variations in shear strength with design parameters such as aspect ratio, axial load ratio, boundary reinforcement ratio, and web reinforcement ratio [60]. This figure emphasizes the complex interplay between structural and material parameters in determining the overall seismic performance of squat shear walls, as also highlighted by Devine et al. [53] and Gondia et al. [63]. Additionally, the damage patterns of various squat shear wall materials, as depicted in Fig. 6, underscore the importance of material properties in influencing seismic behavior. Ultra-high-performance concrete and UHPFRC specimens exhibited distinct damage patterns under different shear stress demands, highlighting the role of material composition in determining the extent and nature of damage during seismic events [57]. These observations are corroborated by the experimental findings of Han et al. [4] and Hosseini et al. [27], which further reinforce the importance of understanding material behavior to optimize the seismic design of squat shear walls.
Figure 5: Variations in shear strength with design parameters: (a) aspect ratio; (b) axial load ratio; (c) boundary reinforcement ratio; (d) web reinforcement ratio (Reprinted with permission from Reference [60], Copyright 2024, Engineering Structures)
Figure 6: Damage patterns of various squat shear wall materials (Reprinted with permission from Reference [57], Copyright 2024, Engineering Structures)
4 Modeling Techniques for Squat Shear Walls
4.1 Analytical and Numerical Modeling Approaches
This section reviews the various modeling approaches employed in the literature, encompassing analytical models, numerical simulations, and machine learning techniques, all supported by extensive experimental validations, Tables 4 and 5. The strut-and-tie model is a widely adopted analytical approach for modeling the internal force distribution within squat shear walls. Chetchotisak et al. [60] developed a strut-and-tie model specifically tailored for predicting the shear strength of squat shear walls under earthquake loads, demonstrating its efficacy in capturing the complex force interactions as shown in Fig. 7. Similarly, Kassem [64] proposed a closed-form design formula based on the strut-and-tie model, enhancing the predictive capabilities for shear strength in squat walls. Massone et al. [65] further advanced this approach by modeling squat structural walls controlled by shear, providing a robust framework for shear response estimation. Massone [66] introduced a shear-flexure interaction model calibrated for squat structural walls, offering improved strength predictions by considering the interplay between shear and flexural forces. This approach was validated through experimental data, showcasing its reliability in practical applications. Chen et al. [59] developed an alternative shear strength equation for RC squat walls, emphasizing the importance of ensuring deformation capacity. This equation provides a simplified yet accurate method for estimating shear strength, facilitating easier design processes. Finite element modeling has been extensively utilized to simulate the behavior of structural components over the past [67–69]. In this context, Belletti et al. [70] employed a PARC-CL model to numerically predict the response of squat shear walls subjected to monotonic loading, achieving high accuracy in load-bearing capacity and deformation predictions. Damoni et al. [71,72] utilized nonlinear finite element analyses to simulate crack propagation and the transition from flexural to shear-dominated behavior, aligning closely with experimental observations. Gopalarathnam et al. [73] conducted nonlinear finite element dynamic analyses of squat shear walls with openings, highlighting the influence of openings on seismic performance. Similarly, Jin et al. [42] performed finite element modeling of squat shear walls under combined cyclic and high axial loads, providing insights into their complex loading responses. Liu et al. [43] investigated the seismic performance of RC squat shear walls with single post-openings reinforced by steel plates through both experimental and numerical methods, validating their finite element model results against empirical data. Kolozvari et al. [74] utilized OpenSees’ capabilities for modeling nonlinear behavior in RC walls and columns, with a focus on combined shear and flexural responses. The paper introduced the shear-flexure interaction MVLEM (SFI-MVLEM) and the fixed-strut-angle model (FSAM) to more accurately capture these interactions. Additionally, new material models, ConcreteCM and SteelMPF, improve the representation of cyclic degradation and prevent stress overshooting. Validated against experimental data, these models enhance load capacity and stiffness degradation predictions, particularly for structures with notable shear-flexure interaction. Petrone et al. [75] presented a versatile numerical model capable of nonlinear analysis for squat-to-tall reinforced-concrete shear walls, accommodating a range of loading conditions and wall geometries. This comprehensive framework allows for the simulation of various failure modes and seismic responses. Additionally, Rasoolinejad et al. [76] examined the size effect on squat shear walls using the microplane model M7, providing insights into how scaling influences seismic performance. This is crucial for ensuring that models remain accurate across different wall sizes and configurations.
Figure 7: Force transfer mechanisms for squat shear walls (Reprinted with permission from Reference [60], Copyright 2024, Engineering Structures)
4.2 Machine Learning-Based Modeling Techniques
Machine learning techniques have gained prominence in predicting the properties and behavior of materials and structures [77–81]. In this context, the behavior of various materials [82,83] and structural elements [84–87] has been estimated. Chen et al. [13] utilized a hybrid artificial neural network-particle swarm optimization model to predict shear strength, demonstrating superior accuracy compared to traditional methods. Goh et al. [88] applied multivariate adaptive regression splines (MARS) and neural network models to forecast shear strength, further validating the potential of these approaches in structural engineering. Gondia et al. [63] introduced mechanics-guided genetic programming expressions for shear strength prediction, integrating physical principles with data-driven methodologies. Nguyen et al. [89] used machine learning-based formulations to predict the shear capacity of squat flanged RC walls, offering a novel approach to seismic design. The presence of openings in squat shear walls introduces additional complexities in their seismic performance. Feng et al. [90] developed an interpretable XGBoost-SHAP machine learning model, enhancing the transparency and reliability of shear strength predictions for squat RC walls. Le Nguyen et al. [91] conducted a comparative study of various machine learning approaches for lateral strength estimation of squat shear walls, highlighting their practical implications and effectiveness. Sulaiman et al. [92] examined the efficiency of the XGBoost algorithm for predicting the shear strength of squat RC walls, performing comprehensive parametric analyses to optimize model performance. Nguyen et al. [93] further improved data-driven models for estimating shear capacity, emphasizing enhanced predictive accuracy and robustness, particularly in the case of random forest (RF) and gradient boosting regression tree (GBRT). Finally, Kazemi et al. [94] introduced an advanced ensemble approach for seismic risk and probability assessment by combining multiple machine learning models with optimization techniques. This stacked model integrates algorithms like decision trees, support vector machines, and gradient boosting and utilizes optimization methods, such as Bayesian and genetic algorithms, to refine model choice and parameters for peak performance. Focused specifically on RC shear walls, it analyzes structural and material factors affecting resilience to earthquakes, achieving 99.1% accuracy for incremental dynamic analysis (IDA) and 99.4% for seismic fragility curves. For user convenience, the study includes a graphical interface (GUI) that displays performance levels and seismic curves and calculates mean annual frequency for seismic hazards, providing a practical tool to support improved seismic safety decisions for concrete structures.
4.3 Material Innovations and Reinforcement Techniques
Shabana et al. [95] investigated the shear strength of GFRP-RC squat walls using the strut-and-tie model, demonstrating significant improvements in seismic performance. Their study underscores the benefits of advanced reinforcement materials in enhancing the ductility and strength of squat shear walls. In addition to that, Shabana et al. [96] investigated the stiffness characteristics of squat walls reinforced with glass FRP (GFRP) bars method to estimate the post-cracking shear stiffness of squat shear walls. Nagib et al. [19] explored the cyclic behavior of squat RC shear walls strengthened with UHPFRC, showcasing enhanced durability and seismic resilience. This approach highlights the potential of fiber-reinforced materials in modern structural design. Kim et al. [10,56] focused on the shear strength modeling of flanged squat walls, particularly in nuclear power plants, emphasizing the critical role of boundary elements. Their models account for high-strength reinforcing bars and boundary flanges, providing accurate shear strength predictions under seismic loads. Kim et al. [97] investigated flanged squat walls reinforced with 690 MPa bars, and found that Incorporating flanges significantly boost shear strength by 40%. Additionally, high-strength bars perform comparably to conventional ones, and shear strength surpasses ACI 318-19 limits by 200%. Woods et al. [46] utilized image analysis methods in the seismic rehabilitation of squat RC shear walls using CFRP sheets, demonstrating effective strengthening strategies. This work highlights the integration of advanced materials and diagnostic techniques in enhancing structural resilience. Weng et al. [98] focused on predicting the lateral load-displacement curves for RC squat walls failing in shear, providing critical data for understanding their deformation characteristics under seismic loading. Finally, Ocampo-Escobar et al. [99] compared the analytical findings with experimental data to identify important parameters impacting the effective stiffness of RC squat walls. Fig. 8a illustrates the reinforcement of the wall with truss elements, while Fig. 8b shows the wall with brick elements and steel reinforcement embedded into the wall.
Figure 8: (a) Reinforcement of the wall with truss elements; (b) wall with brick elements and steel reinforcement embedded into the wall (Reprinted from Reference [99])
Looi et al. [100] developed ultimate drift prediction models for rectangular squat RC shear walls, facilitating better seismic performance assessments. Seif Eldin et al. [101] studied the seismic performance parameters of fully grouted reinforced masonry squat shear walls, while Faraone et al. [51] analyzed damage patterns in both squat and flexural RC shear walls, contributing to a deeper understanding of failure mechanisms. Massone et al. [102] developed a single-panel model for estimating the shear response of squat RC walls, simplifying the analysis while maintaining high predictive accuracy. This approach is particularly useful for preliminary design and assessment purposes. Many studies integrate multiple modeling techniques to enhance prediction accuracy and reliability. For instance, Damoni et al. [71,72] combined finite element methods with discrete element models to capture complex behaviors such as cracking and shear-flexure interactions. Fig. 9 shows the squat RC wall configuration setup, while Fig. 10 demonstrates the verification of the prediction model compared to tested high axial load ratio ALR squat walls.
Figure 9: Squat RC wall configuration setup (Reprinted from Reference [100])
Figure 10: Verification of prediction model compared to tested high axial load ratio ALR squat walls (Reprinted from Reference [100])
5 Future Research Recommendations
Despite the extensive research on squat shear walls, several gaps and unresolved issues remain that warrant further investigation. One critical area is the need for comprehensive experimental studies that focus on the use of non-conventional materials and innovative strategies in squat shear walls. Existing studies have provided valuable insights, but there is a lack of large-scale experimental programs that can capture the full range of behaviors and failure modes under various seismic scenarios. These studies should include advanced materials such as UHPC and FRPs to fully understand their impact on seismic resilience. Another significant gap is the integration of environmental factors into the analysis and design of squat shear walls. While some studies have highlighted the impact of environmental degradation on seismic performance, more research is needed to evaluate the use of advanced materials like UHPC and HPC in terms of their environmental impact, particularly CO2 emissions and other gases. Developing models and design guidelines that account for long-term environmental exposure will be crucial for sustainable construction practices. The development of more accurate and realistic analytical models is also necessary. Current models often simplify the interactions between different types of reinforcement and concrete, which can lead to discrepancies between predicted and observed behaviors. Future research should focus on refining these models to improve design standards, incorporating the complexities of material behavior, and providing more reliable predictions of structural performance. Numerical simulations have proven to be invaluable tools, but there is a need to adopt advanced models such as artificial intelligence and machine learning to leverage the large amounts of accumulated data over time. These technologies can help develop more efficient algorithms and modeling approaches that provide accurate predictions with reduced computational effort. Additionally, validating these models against a broader range of experimental data is essential to ensure their reliability and applicability. Table 6 lists the future research recommendations on squat-RC shear walls.
This study aimed to address the significant gap in the literature regarding the seismic behavior of squat RC shear walls, particularly under extreme earthquake conditions. Unlike tall, slender walls, squat walls exhibit unique failure mechanisms and deformation characteristics that are not well understood. Through a comprehensive literature review, this study synthesized current research findings from experimental investigations, analytical models, and numerical simulations to provide a detailed understanding of squat shear walls’ performance during seismic events. The primary objective was to identify critical factors influencing their behavior and offer insights to guide the design and construction of more resilient structures in earthquake-prone regions. Based on the aforementioned statements, the following conclusions are drawn:
• Squat shear walls exhibit higher stiffness and lower deformability compared to slender walls, making them more suitable for low-rise buildings with space constraints. The inclusion of detailed reinforcement significantly improves energy dissipation and prevents brittle failures.
• The use of HPC and FRPs enhances the seismic resilience of squat shear walls. These advanced materials increase load-bearing capacity, improve ductility, and reduce deformations.
• Incorporating hybrid reinforcement techniques, seismic isolation systems, and the use of advanced materials like UHPC and steel fibers significantly improves the load-bearing capacity, energy dissipation, and overall seismic resilience of squat shear walls.
• Analytical models like the strut-and-tie model and frame analysis, along with numerical simulations such as the finite element method and discrete element method, have proven effective in predicting the seismic performance of squat shear walls. These models accurately simulate load-bearing capacity, deformation patterns, and failure mechanisms.
Despite the extensive research on this topic, several limitations and areas for future research were identified. There is a need for comprehensive experimental studies focusing on non-conventional materials and innovative strategies to capture the full range of behaviors and failure modes under various seismic scenarios. Additionally, integrating environmental factors into the analysis and design of squat shear walls is crucial for sustainable construction practices. Developing more accurate and realistic analytical models to predict the interaction between different reinforcement types and concrete will enhance design standards. Leveraging artificial intelligence and machine learning technologies can further refine numerical simulations, providing accurate predictions with reduced computational effort. Future research should also focus on validating these models against a broader range of experimental data to ensure their reliability and applicability.
Acknowledgement: None.
Funding Statement: This research received no specific grant from any funding agency in the public, commercial, or not-for-profit sectors.
Author Contributions: Ahed Habib, Zaid A. Al-Sadoon, Murat Saatcioglu, Ausamah Al Houri, Mohamed Maalej, Salah Al-Toubat, Mazen Shrif: contributed to the conception and design of the study. Ahed Habib, Zaid A. Al-Sadoon, Murat Saatcioglu, Ausamah Al Houri, Mohamed Maalej, Salah Al-Toubat, Mazen Shrif: conducted the literature review. Ahed Habib, Zaid A. Al-Sadoon, Murat Saatcioglu, Ausamah Al Houri, Mohamed Maalej, Salah Al-Toubat, Mazen Shrif: wrote the main manuscript text. Ahed Habib, Zaid A. Al-Sadoon, Murat Saatcioglu, Ausamah Al Houri, Mohamed Maalej, Salah Al-Toubat, Mazen Shrif: prepared figures and tables. All authors reviewed the results and approved the final version of the manuscript.
Availability of Data and Materials: The data that support the findings of this study are available from the corresponding author upon reasonable request.
Ethics Approval: Not applicable.
Conflicts of Interest: The authors declare no conflicts of interest to report regarding the present study.
References
1. Epackachi S, Sharma N, Whitaker A, Hortacsu A. A cyclic backbone curve for squat reinforced concrete shear walls. In: 11th National Conference on Earthquake Engineering 2018, 2018; Los Angeles, CA, USA. [Google Scholar]
2. Guan M, Sha M, Wang J, Hang X, Jin G. Cyclic behavior of self-slitting squat composite shear walls with concrete-filled steel tubes: experiment. J Constr Steel Res. 2023;210(160):108054. doi:10.1016/j.jcsr.2023.108054. [Google Scholar] [CrossRef]
3. Arafa A, Farghaly AS, Benmokrane B. Prediction of flexural and shear strength of concrete squat walls reinforced with GFRP bars. J Compos Constr. 2018;22(4):0000854. doi:10.1061/(ASCE)CC.1943-5614.000085. [Google Scholar] [CrossRef]
4. Han W, Zhao Z, Qian J, Zhang Y, Ma T. Experimental seismic behavior of squat shear walls with precast concrete hollow molds. Earthq Eng Eng Vib. 2019;18(4):540–7. doi:10.1007/s11803-019-0540-7. [Google Scholar] [CrossRef]
5. Choi CS. Improvement of earthquake-resistant performance of squat shear walls under reversed cyclic loads. Key Eng Mater. 2006;324–325:535–40. doi:10.4028/www.scientific.net/KEM.324-325.535. [Google Scholar] [CrossRef]
6. Li YY, Ding R, Nie JG. Experiment study on seismic behavior of squat UHPC shear walls subjected to tension-shear combined cyclic load. Eng Struct. 2023;280(1):115700. doi:10.1016/j.engstruct.2023.115700. [Google Scholar] [CrossRef]
7. Li YY, Nie JG, Ding R, Fan JS. Seismic performance of squat UHPC shear walls subjected to high-compression shear combined cyclic load. Eng Struct. 2023;276(4):115369. doi:10.1016/j.engstruct.2022.115369. [Google Scholar] [CrossRef]
8. Chen M, Gao Y, Zhou M, Sun L, Gao L, Huang Z. Experimental analysis of seismic behavior of steel reinforced concrete squat shear walls with steel plates. J Civ Archit Environ Eng. 2017;39(6):2017.06.004. [Google Scholar]
9. El-Dakhakhni WW, Banting BR, Miller SC. Seismic performance parameter quantification of shear-critical reinforced concrete masonry squat walls. J Struct Eng. 2013;139(6):0000713. doi:10.1061/(ASCE)ST.1943-541X.0000713. [Google Scholar] [CrossRef]
10. Kim JH, Lee Y, Park HG. Shear strength model for flanged squat walls in nuclear power plants. J Korea Concr Inst. 2022;34(3):311–23. doi:10.4334/JKCI.2022.34.3.311. [Google Scholar] [CrossRef]
11. Fathalla E, Ringeisen B, Lenges M, Mihaylov B. Shear behavior of full-scale squat shear walls with and without precast pre-walls. J Adv Concr Technol. 2024;22(2):86–102. doi:10.3151/jact.22.86. [Google Scholar] [CrossRef]
12. Akl A, Ezzeldin M. Seismic collapse risk assessment of low-aspect-ratio reinforced concrete shear walls using the FEMA P695 methodology. J Struct Eng. 2023;149(2):04022237. doi:10.1061/(ASCE)ST.1943-541X.0003505. [Google Scholar] [CrossRef]
13. Chen XL, Fu JP, Yao JL, Gan JF. Prediction of shear strength for squat RC walls using a hybrid ANN-PSO model. Eng Comput. 2018;34(2):547–56. doi:10.1007/s00366-017-0547-5. [Google Scholar] [CrossRef]
14. Hidalgo PA, Ledezma CA, Jordan RM. Seismic behavior of squat reinforced concrete shear walls. Earthq Spectra. 2002;18(2):149–66. doi:10.1193/1.1490353. [Google Scholar] [CrossRef]
15. Gulec CK, Whittaker AS, Stojadinovic B. Shear strength of squat rectangular reinforced concrete walls. ACI Struct J. 2008;105(4):488–97. [Google Scholar]
16. Rong XL, Zheng SS, Zhang YX, Dong LG, Liu H, Dai KY. Seismic behavior of frost-damaged squat RC shear walls under artificial climate environment: a further experimental research. Arch Civ Mech Eng. 2020;20(4):1327–45. doi:10.1007/s43452-020-00081-7. [Google Scholar] [CrossRef]
17. Zheng S, Qin Q, Yang W, Gan C, Zhang Y, Ding S. Experimental research on the seismic behaviors of squat RC shear walls under offshore atmospheric environment. J Harbin Inst Technol. 2015;47(12):210–22. [Google Scholar]
18. Yu ZJ, Han SJ, Jang SJ, Yun HD. Seismic performance of strain-hardening cement composite (SHCC) squat shear walls with vertical slits. Appl Mech Mater. 2014;525:427–33. doi:10.4028/www.scientific.net/AMM.525.427. [Google Scholar] [CrossRef]
19. Nagib MT, Sakr MA, El-khoriby SR, Khalifa TM. Cyclic behavior of squat reinforced concrete shear walls strengthened with ultra-high-performance fiber-reinforced concrete. Eng Struct. 2021;246(3):112999. doi:10.1016/j.engstruct.2021.112999. [Google Scholar] [CrossRef]
20. Tariq M, Khan A, Ullah A, Zamin B, Kashyzadeh KR, Ahmad M. Gene expression programming for estimating shear strength of RC squat wall. Buildings. 2022;12(7):0918. doi:10.3390/buildings12070918. [Google Scholar] [CrossRef]
21. Sivaguru V, Rao GA. Strength and behavior of reinforced concrete squat shear walls with openings under cyclic loading. ACI Struct J. 2021;118(5):51732832. doi:10.14359/51732832. [Google Scholar] [CrossRef]
22. Peng Y, Wu H, Zhuge Y. Strength and drift capacity of squat recycled concrete shear walls under cyclic loading. Eng Struct. 2015;100:356–68. doi:10.1016/j.engstruct.2015.06.025. [Google Scholar] [CrossRef]
23. Zheng S, Li Q, Qin Q, Gan C, Liu W, Dong L. Restoring force model of freezing-thawing damaged squat reinforced concrete shear walls. J Build Struct. 2018;39(3):2018.03.014. [Google Scholar]
24. Yang KH, Mun JH, Hwang YH, Song JK. Cyclic tests on slip resistance of squat heavyweight concrete shear walls with construction joints. Eng Struct. 2017;141:334–44. doi:10.1016/j.engstruct.2017.03.054. [Google Scholar] [CrossRef]
25. Eid R, Dancygier AN, Jaber G. Mechanical properties of low-performance concrete (LPC) and shear capacity of old unreinforced LPC squat walls. Materials. 2021;14(23):237310. doi:10.3390/ma14237310. [Google Scholar] [PubMed] [CrossRef]
26. Tong X, Fang Z, Luo X, Gong L. Study on shear capacity of ultra-high-performance concrete squat shear walls. Case Stud Constr Mater. 2020;12:e00314. doi:10.1016/j.cscm.2019.e00314. [Google Scholar] [CrossRef]
27. Hosseini SA, Kheyroddin A, Mastali M. An experimental investigation into the impacts of eccentric openings on the in-plane behavior of squat RC shear walls. Eng Struct. 2019;197:109410. doi:10.1016/j.engstruct.2019.109410. [Google Scholar] [CrossRef]
28. Zheng SS, Zhou Y, Li QQ, Long L, Dong LG, He JC. Experimental study on aseismic behavior of squat RC shear walls due to chloride ion erosion. Eng Mech. 2018;36(12):2018.11.0592. doi:10.6052/j.issn.1000-4750.2018.11.0592. [Google Scholar] [CrossRef]
29. Zheng S, Li Q, Qin Q, Zuo H, Dong L, Liu W. Experimental study on seismic behaviors of corroded squat reinforced concrete shear walls. J Build Struct. 2019;40(8):2017.0212. [Google Scholar]
30. Zheng SS, Sang ZW, Zhou Y. Seismic test and shear strength prediction of squat RC shear walls under acidic environment. Eng Mech. 2023;40(3):213–24. doi:10.6052/j.issn.1000-4750.2021.09.074. [Google Scholar] [CrossRef]
31. Yang W, Zheng SS, Zhang DY, Sun LF, Gan CL. Seismic behaviors of squat reinforced concrete shear walls under freeze-thaw cycles: a pilot experimental study. Eng Struct. 2016;124(1):367–78. doi:10.1016/j.engstruct.2016.06.013. [Google Scholar] [CrossRef]
32. Habibi F, Sheikh SA, Vecchio F, Panesar DK. Effects of alkali-silica reaction on concrete squat shear walls. ACI Struct J. 2018;115(5):51702238. doi:10.14359/51702238. [Google Scholar] [CrossRef]
33. Gulec CK, Whittaker AS, Stojadinovic B. Peak shear strength of squat reinforced concrete walls with boundary barbells or flanges. ACI Struct J. 2009;106(3):368–77. [Google Scholar]
34. Lim WG, Kang SW, Yun HD. Shear behavior of squat steel fiber reinforced concrete (SFRC) shear walls with vertical slits. Appl Mech Mater. 2013;372:207–12. doi:10.4028/www.scientific.net/AMM.372.207. [Google Scholar] [CrossRef]
35. Kim JH, Park HG. Shear and shear-friction strengths of squat walls with flanges. ACI Struct J. 2020;117(6):51728075. doi:10.14359/51728075. [Google Scholar] [CrossRef]
36. Yang HJ, Yun HD. Vertical seam effect on seismic performance of reinforced concrete squat shear walls with rectangular cross-section. Adv Mater Res. 2013;663:159–63. doi:10.4028/www.scientific.net/AMR.663.159. [Google Scholar] [CrossRef]
37. Nagib MT, Sakr MA, El-khoriby SR, Khalifa TM. Interfacial shear behavior between UHPFRC layers and normal concrete substrate for shear-strengthened squat RC shear walls under cyclic loading. Eng Struct. 2022;254:113850. doi:10.1016/j.engstruct.2022.113850. [Google Scholar] [CrossRef]
38. Kang SW, Yun HD. Effect of cement matrix’s type on the shear performance of lightly reinforced squat shear walls subjected to cyclic loading. Adv Mater Res. 2013;658:42–50. doi:10.4028/www.scientific.net/AMR.658.42. [Google Scholar] [CrossRef]
39. Miao L, Jin L, Chen F, Du X. Experiment study on seismic performance and size effect in BFRP-RC squat shear walls with different horizontal reinforcement ratios. Eng Struct. 2023;295:116888. doi:10.1016/j.engstruct.2023.116888. [Google Scholar] [CrossRef]
40. Elzokra AA, Al Houri A, Habib A, Habib M, Malkawi AB. Shrinkage behavior of conventional and nonconventional concrete: a review. Civ Eng J. 2020;6(9):1839–51. doi:10.28991/cej-2020-03091586. [Google Scholar] [CrossRef]
41. Al Houri A, Habib A, Elzokra A, Habib M. Tensile testing of soils: history, equipment, and methodologies. Civ Eng J. 2020;6(3):591–601. doi:10.28991/cej-2020-03091494. [Google Scholar] [CrossRef]
42. Jin C, Su Y, Pan Z, Meng S. Experimental study and finite element modelling of squat shear walls under combined cyclic loads and high axial loads. Buildings. 2023;13(8):2104. doi:10.3390/buildings13082104. [Google Scholar] [CrossRef]
43. Liu M, Yuan G, Sun W, Shu Q, Zhao Z, Lu L. Experimental and numerical investigation on the seismic performance of RC squat shear walls with single post-opening reinforced by steel plates. Eur J Environ Civ Eng. 2024;28(1):2200464. doi:10.1080/19648189.2023.2200464. [Google Scholar] [CrossRef]
44. Shadan F, Khaloo A, Shadan P. Numerical study on flexural strengthening of squat RC shear wall using FRP laminates. Sci Iran. 2015;22(1):0000. [Google Scholar]
45. Hung CC, Hsieh PL. Comparative study on shear failure behavior of squat high-strength steel reinforced concrete shear walls with various high-strength concrete materials. Structures. 2020;23:293–304. doi:10.1016/j.istruc.2019.11.002. [Google Scholar] [CrossRef]
46. Woods JE, Lau DT, Yang YS. Image analysis method used in the seismic rehabilitation of squat reinforced concrete shear walls using CFRP sheets. In: Proceedings of the 24th Australasian Conference on the Mechanics of Structures and Materials, 2017; Australia. [Google Scholar]
47. Xu G, Zheng L, Zhou W, Zhai C, Wang D. Quasi-static tests of squat reinforced concrete shear walls with openings. Eng Struct. 2023;293:116666. doi:10.1016/j.engstruct.2023.116666. [Google Scholar] [CrossRef]
48. Trost B. Interaction of sliding, shear, and flexure in the seismic response of squat reinforced concrete shear walls (Doctoral Dissertation). ETH Zurich: Switzerland; 2017. [Google Scholar]
49. Pizarro Pohl D, Kovarbasic M, Stojadinovic B. Experimental investigation of the sliding failure mode in full-scale squat reinforced concrete shear wall specimen. In: Proceedings of the Third European Conference on Earthquake Engineering and Seismology–3ECEES, 2022; Bucharest; p. 938–44. doi:10.3929/ethz-b-000584291 [Google Scholar] [CrossRef]
50. Schuler H, Trost B. Sliding shear resistance of squat walls under reverse loading: mechanical model and parametric study. ACI Struct J. 2016;113(4):51688748. [Google Scholar]
51. Faraone G, Hutchinson T, Piccinin R, Silva J. Damage patterns in squat and flexural RC shear walls. In:Structures Congress 2020-Selected Papers from the Structures Congress 2020, 2020; USA; p. 1–12. [Google Scholar]
52. Thomson ED, Perdomo ME, Picn R, Marante ME, Flrez-Lpez J. Simplified model for damage in squat RC shear walls. Eng Struct. 2009;31(10):1335–45. doi:10.1016/j.engstruct.2009.05.020. [Google Scholar] [CrossRef]
53. Devine RD, Barbachyn SM, Thrall AP, Kurama YC. Effect of aspect ratio, flanges, and material strength on squat reinforced concrete shear walls. ACI Struct J. 2020;117(5):51725845. doi:10.14359/51725845. [Google Scholar] [CrossRef]
54. Luo P, Liu J. An experimental study of the mechanical behavior of squat shear walls built with precast concrete two-way hollow slabs. J S Afr Inst Civ Eng. 2022;64(2):2309–8775. doi:10.17159/2309-8775/2022/v64no2a5. [Google Scholar] [CrossRef]
55. Zhang L, Han X, Chen X, Ji J. Experimental study on the seismic behavior of squat SRC shear walls with high axial load ratio. Buildings. 2022;12(8):1238. doi:10.3390/buildings12081238. [Google Scholar] [CrossRef]
56. Kim JH, Kim YJ, Park HG. Modeling of shear strength for squat reinforced concrete walls with boundary elements. ACI Struct J. 2023;120(6):51739090. doi:10.14359/51739090. [Google Scholar] [CrossRef]
57. Hung CC, Li H, Chen HC. High-strength steel reinforced squat UHPFRC shear walls: cyclic behavior and design implications. Eng Struct. 2017;141(6):374–86. doi:10.1016/j.engstruct.2017.02.068. [Google Scholar] [CrossRef]
58. Ganesan N, Indira PV, Seena P. High-performance fiber-reinforced concrete squat shear walls with barbells. Indian Concr J. 2015;89(4):123–35. [Google Scholar]
59. Chen XL, Fu JP, Sun L, Yao JL. An alternative shear strength equation of reinforced concrete squat walls for ensuring the deformation capacity. Int J Earth Sci Eng. 2016;9(5):123–31. [Google Scholar]
60. Chetchotisak P, Chomchaipol W, Teerawong J, Shaingchin S. Strut-and-tie model for predicting shear strength of squat shear walls under earthquake loads. Eng Struct. 2022;256:114042. doi:10.1016/j.engstruct.2022.114042. [Google Scholar] [CrossRef]
61. Hosseini SM, Yekrangnia M, Shakiba M, Bazli M, Oskouei AV. Experimental study on seismic performance of squat RC shear walls reinforced with hybrid steel and GFRP rebars. Structures. 2024;64:106487. doi:10.1016/j.istruc.2024.106487. [Google Scholar] [CrossRef]
62. Ma J, Li B. Influence of lateral loading direction on the peak shear strength of non-rectangular reinforced concrete squat walls. Adv Struct Eng. 2019;22(11):42071. doi:10.1177/1369433219842071. [Google Scholar] [CrossRef]
63. Gondia A, Ezzeldin M, El-Dakhakhni W. Mechanics-guided genetic programming expression for shear-strength prediction of squat reinforced concrete walls with boundary elements. J Struct Eng. 2020;146(11):0002734. doi:10.1061/(ASCE)ST.1943-541X.000273. [Google Scholar] [CrossRef]
64. Kassem W. Shear strength of squat walls: a strut-and-tie model and closed-form design formula. Eng Struct. 2015;84:101–12. doi:10.1016/j.engstruct.2014.11.027. [Google Scholar] [CrossRef]
65. Massone LM, Orakcal K, Wallace JW. Modeling of squat structural walls controlled by shear. ACI Struct J. 2009;106(5):51663105. doi:10.14359/51663105. [Google Scholar] [CrossRef]
66. Massone LM. Strength prediction of squat structural walls via calibration of a shear-flexure interaction model. Eng Struct. 2010;32(4):1231–40. doi:10.1016/j.engstruct.2009.12.018. [Google Scholar] [CrossRef]
67. Hendawi S, Frangopol DM. Design of composite hybrid plate girder bridges based on reliability and optimization. Struct Saf. 1994;15(1–2):149–65. doi:10.1016/0167-4730(94)90057-4. [Google Scholar] [CrossRef]
68. Abed FH, AlHamaydeh MH, Barakat SA. Nonlinear finite-element analysis of buckling capacity of pretwisted steel bars. J Eng Mech. 2013;139(7):791–801. doi:10.1061/(ASCE)EM.1943-7889.0000528. [Google Scholar] [CrossRef]
69. Kizilarslan E, Kenarangi H, Bruneau M. Finite element modeling of composite plate shear walls/concrete-filled (C-PSW/CF). Structures. 2024;65:106668. doi:10.1016/j.istruc.2024.106668. [Google Scholar] [CrossRef]
70. Belletti B, Esposito R, Damoni C. Numerical prediction of the response of a squat shear wall subjected to monotonic loading through PARC-CL model. In: Proceedings of the 8th International Conference on Fracture Mechanics of Concrete and Concrete Structures, FraMCoS, 2013; Spain; p. 1–12. [Google Scholar]
71. Damoni C, Belletti B, Lilliu G. Control of cracking in RC structures: numerical simulation of a squat shear wall. In: Proceedings of the 8th International Conference on Fracture Mechanics of Concrete and Concrete Structures, FraMCoS 2013, 2013; Spain; p. 1–15. [Google Scholar]
72. Damoni C, Belletti B, Esposito R. Numerical prediction of the response of a squat shear wall subjected to monotonic loading. Eur J Environ Civ Eng. 2014;18(7):896–753. doi:10.1080/19648189.2014.896753. [Google Scholar] [CrossRef]
73. Gopalarathnam M, Kumar M. Nonlinear finite element dynamic analysis of squat shear wall with openings. Int J Adv Struct Eng. 2013;5:27. doi:10.1186/2008-6695-5-27. [Google Scholar] [CrossRef]
74. Kolozvari K, Orakcal K, Wallace JW. New OpenSees models for simulating nonlinear flexural and coupled shear-flexural behavior of RC walls and columns. Comput Struct. 2018;196:246–62. doi:10.1016/j.compstruc.2017.10.010. [Google Scholar] [CrossRef]
75. Petrone F, McKenna F, Do T, McCallen D. A versatile numerical model for the nonlinear analysis of squat-to-tall reinforced-concrete shear walls. Eng Struct. 2021;242:112406. doi:10.1016/j.engstruct.2021.112406. [Google Scholar] [CrossRef]
76. Rasoolinejad M, Baant ZP. Size effect of squat shear walls extrapolated by microplane model M7. ACI Struct J. 2019;116(3):51714478. doi:10.14359/51714478. [Google Scholar] [CrossRef]
77. Alhamaydeh MH, Barakat SA, Abed FH. Multiple regression modeling of natural rubber seismic-isolation systems with supplemental viscous damping for near-field ground motion. J Civ Eng Manag. 2013;19(5):665–82. doi:10.3846/13923730.2013.799089. [Google Scholar] [CrossRef]
78. Habib A, Yildirim U. Simplified modeling of rubberized concrete properties using multivariable regression analysis. Mater Constr. 2022;72(347):e289. doi:10.3989/mc.2022.13621. [Google Scholar] [CrossRef]
79. Habib A, Yildirim U. Estimating mechanical and dynamic properties of rubberized concrete using machine learning techniques: a comprehensive study. Eng Comput. 2022;39(8):3129–78. doi:10.1108/EC-09-2021-0527. [Google Scholar] [CrossRef]
80. Moein MM, Saradar A, Rahmati K, Mousavinejad SHG, Bristow J, Aramali V, et al. Predictive models for concrete properties using machine learning and deep learning approaches: a review. J Build Eng. 2023;63:105444. doi:10.1016/j.jobe.2022.105444. [Google Scholar] [CrossRef]
81. Zhang S, Xu J, Lai T, Yu Y, Xiong W. Bond stress estimation of profiled steel-concrete in steel reinforced concrete composite structures using ensemble machine learning approaches. Eng Struct. 2023;294:116725. doi:10.1016/j.engstruct.2023.116725. [Google Scholar] [CrossRef]
82. Habib A, Yildirim U, Habib M. Applying kernel principal component analysis for enhanced multivariable regression modeling of rubberized concrete properties. Arab J Sci Eng. 2023;48(4):5383–96. doi:10.1007/s13369-022-07435-8. [Google Scholar] [CrossRef]
83. Habib A, Barakat S, Al-Toubat S, Junaid MT, Maalej M. Developing machine learning models for identifying the failure potential of fire-exposed FRP-strengthened concrete beams. Arab J Sci Eng. 2024;5(1):19. doi:10.1007/s13369-024-09497-2. [Google Scholar] [CrossRef]
84. Al Houri A, Habib A, Al-Sadoon ZA. Artificial intelligence-based design and analysis of passive control structures: an overview. J Soft Comput Civ Eng. 2024. doi:10.22115/scce.2024.450722.1832. [Google Scholar] [CrossRef]
85. Shrif M, Al-Sadoon ZA, Barakat S, Habib A, Mostafa O. Optimizing gene expression programming to predict shear capacity in corrugated web steel beams. Civ Eng J. 2024;10(5):1370–85. doi:10.28991/CEJ-2024-010-05-02. [Google Scholar] [CrossRef]
86. Asgarkhani N, Kazemi F, Jankowski R. Machine learning-based prediction of residual drift and seismic risk assessment of steel moment-resisting frames considering soil-structure interaction. Comput Struct. 2023;289(4):107181. doi:10.1016/j.compstruc.2023.107181. [Google Scholar] [CrossRef]
87. Asgarkhani N, Kazemi F, Jakubczyk-Gałczyńska A, Mohebi B, Jankowski R. Seismic response and performance prediction of steel buckling-restrained braced frames using machine-learning methods. Eng Appl Artif Intell. 2024;128(5):107388. doi:10.1016/j.engappai.2023.107388. [Google Scholar] [CrossRef]
88. Goh ATC, Zhang W. MARS and neural network models for shear strength prediction of squat reinforced concrete walls: Shear strength of squat reinforced concrete walls. In: Modeling and simulation techniques in structural engineering. 2016; USA: IGI Global. p. 1–12. doi: 10.4018/978-1-5225-0588-4.ch010. [Google Scholar] [CrossRef]
89. Nguyen DD, Tran VL, Ha DH, Nguyen VQ, Lee TH. A machine learning-based formulation for predicting shear capacity of squat flanged RC walls. Structures. 2021;29:1850–66. doi:10.1016/j.istruc.2020.12.054. [Google Scholar] [CrossRef]
90. Feng DC, Wang WJ, Mangalathu S, Taciroglu E. Interpretable XGBoost-SHAP machine-learning model for shear strength prediction of squat RC walls. J Struct Eng. 2021;147(11):0003115. doi:10.1061/(ASCE)ST.1943-541X.0003115. [Google Scholar] [CrossRef]
91. Le Nguyen K, Trinh HT, Banihashemi S, Pham TM. Machine learning approaches for lateral strength estimation in squat shear walls: a comparative study and practical implications. Expert Syst Appl. 2024;239:122458. doi:10.1016/j.eswa.2023.122458. [Google Scholar] [CrossRef]
92. Sulaiman BH, Ibrahim AM, Imran HJ. Study the efficiency of the XGBoost algorithm for squat RC wall shear strength prediction and parametric analysis. Diyala J Eng Sci. 2024;17(1):17110. doi:10.24237/djes.2024.17110. [Google Scholar] [CrossRef]
93. Nguyen TH, Nguyen DD. Improved data-driven models for estimating shear capacity of squat rectangular reinforced concrete walls. Asian J Civ Eng. 2024;25(3):941–56. doi:10.1007/s42107-023-00941-6. [Google Scholar] [CrossRef]
94. Kazemi F, Asgarkhani N, Jankowski R. Optimization-based stacked machine-learning method for seismic probability and risk assessment of reinforced concrete shear walls. Expert Syst Appl. 2024;255:124897. doi:10.1016/j.eswa.2024.124897. [Google Scholar] [CrossRef]
95. Shabana I, Farghaly AS, Benmokrane B. Shear strength of glass fiber-reinforced polymer-reinforced concrete squat walls: strut-and-tie model. ACI Struct J. 2023;120(2):51738347. doi:10.14359/51738347. [Google Scholar] [CrossRef]
96. Shabana I, Farghaly AS, Benmokrane B. Shear stiffness of earthquake-resistant concrete squat walls reinforced with glass fiber-reinforced polymer bars. ACI Struct J. 2023;120(2):51738345. doi:10.14359/51738345. [Google Scholar] [CrossRef]
97. Kim JH, Park HG. Shear strength of flanged squat walls with 690 MPa reinforcing bars. ACI Struct J. 2022;119(2):51734142. doi:10.14359/51734142. [Google Scholar] [CrossRef]
98. Weng PW, Li YA, Tu YS, Hwang SJ. Prediction of the lateral load-displacement curves for reinforced concrete squat walls failing in shear. J Struct Eng. 2017;143(10):0001872. doi:10.1061/(ASCE)ST.1943-541X.00018. [Google Scholar] [CrossRef]
99. Ocampo-Escobar AF, Vidot-Vega AL. Effects of concrete parameters in the lateral stiffness of reinforced concrete squat walls. Int J Adv Struct Eng. 2019;11:321–30. doi:10.1007/s40091-019-0233-5. [Google Scholar] [CrossRef]
100. Looi DTW, Su R, Cheng B, Zhou MJ. Ultimate drift prediction models of rectangular squat reinforced concrete shear walls. In: Proceedings of the 24th Australasian Conference on the Mechanics of Structures and Materials, ACMSM24 2016, 2017; Australia; p. 1–12. [Google Scholar]
101. Seif Eldin HM, Ashour A, Galal K. Seismic performance parameters of fully grouted reinforced masonry squat shear walls. Eng Struct. 2019;187:201–14. doi:10.1016/j.engstruct.2019.02.069. [Google Scholar] [CrossRef]
102. Massone LM, Ulloa MA. Shear response estimate for squat reinforced concrete walls via a single panel model. Earthq Struct. 2014;7(5):647–61. doi:10.12989/eas.2014.7.5.647. [Google Scholar] [CrossRef]
103. Mun JH, Yang KH. Load capacity of squat RC shear walls by strut-and-tie model. Mag Concr Res. 2016;68(24):1206–19. doi:10.1680/jmacr.15.00523. [Google Scholar] [CrossRef]
104. Mun JH, Yang KH, Song JK. Shear behavior of squat heavyweight concrete shear walls with construction joints. ACI Struct J. 2017;114(4):51689785. doi:10.14359/51689785. [Google Scholar] [CrossRef]
105. Aydin AC, Bayrak B, Maali M, Kilic M, Alcan HG. Shear wall design within the light of prominent standards. Civ Eng Beyond Limits. 2020;4:13–9. doi:10.36937/cebacom.2020.004.003. [Google Scholar] [CrossRef]
Cite This Article
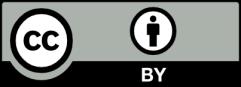
This work is licensed under a Creative Commons Attribution 4.0 International License , which permits unrestricted use, distribution, and reproduction in any medium, provided the original work is properly cited.