Open Access
ARTICLE
Evaluation of Some Egyptian Barley Cultivars Resistance to Foliar Fungal Diseases in Drought-Prone Environments under Field Conditions
1 Basic Sciences and Their Applications Unit, Health Specialties, Applied College, King Khalid, 62529, Saudi Arabia
2 Plant Pathology Research Institute, Agriculture Research Center, Giza, 12619, Egypt
3 Department of Geography and Environmental Sustainability, College of Humanities and Social Sciences, Princess Nourah bint Abdulrahman University, Riyadh, 11671, Saudi Arabia
4 Department of Agricultural Biotechnology, College of Agriculture and Food Sciences, King Faisal University, Al-Ahsa, 31982, Saudi Arabia
5 Field Crops Research Institute, Agriculture Research Center, Giza, 12619, Egypt
6 Department of Plant Pathology, University of Florida, Gainesville, FL 32611-0180, USA
7 Department of Biological Sciences, Faculty of Science, King Abdulaziz University, Jeddah, 21589, Saudi Arabia
8 Department of Biology, College of Science, Qassim University, Buraydah, 52571, Saudi Arabia
9 Department of Plant Production (Genetic Branch), Faculty of Environmental Agricultural Sciences, Arish University, El-Arish, 45511, Egypt
10 Department of Biology, College of Science, King Khalid University, Abha, 61421, Saudi Arabia
11 Department of Botany and Microbiology, Faculty of Science, South Valley University, Qena, 83523, Egypt
12 Department of Botany and Microbiology, Faculty of Science, Damanhour University, Damanhour, 22511, Egypt
* Corresponding Author: Mohamed Abou-Zeid. Email:
(This article belongs to the Special Issue: Influence of Biotic and Abiotic Stresses Signals on Plants and their Performance at Different Environments)
Phyton-International Journal of Experimental Botany 2025, 94(2), 347-377. https://doi.org/10.32604/phyton.2025.057448
Received 18 August 2024; Accepted 10 December 2024; Issue published 06 March 2025
Abstract
Barley (Hordeum vulgare L.) is a significant global crop that thrives in various climatic and drought-stress conditions. Furthermore, increased drought intervals and more significant weather variability resulting from climate change can affect the severity of plant diseases. Therefore, two primary objectives of integrated disease management regarding climate change are identifying cultivars resistant to foliar diseases and understanding disease progression under abiotic stress. In the current study, we assessed the quantitative foliar disease resistance of 17 commercial barley cultivars under both normal and water stress conditions over two growing seasons (from 2020/21 to 2021/22). The findings demonstrated a reduced incidence of foliar fungal diseases (leaf rust, net blotch, and powdery mildew) under severe drought stress relative to standard irrigated field conditions. The barley cultivars (Giza 130, Giza 131, and Giza 133) demonstrated significant differences across all disease resistance indices. In addition, the study aimed to molecularly characterize 17 commercial barley varieties using single-cell DNA testing (SCoT) to identify genetic polymorphism and specific markers for each genotype. Eight SCoT primers were employed to investigate the genetic polymorphism among 17 barley varieties. Furthermore, these cultivars exhibited optimal performance for the majority of agricultural attributes examined, both under normal and water-stressed conditions.Keywords
Barley (Hordeum vulgare L.) ranks as the fourth-most significant cereal crop globally, primarily due to its resilience and ability to adapt to various challenging conditions [1]. Due to its high nutritional fiber content, it serves as animal feed and a staple diet for numerous populations worldwide [2–4]. According to FAOSTAT [5], global barley cultivation spanned 46.9 million hectares, yielding approximately 141 million tons in the 2019–2020 period. Barley constitutes a significant winter crop in Egypt. It thrives under various climatic and drought-stress conditions [6,7]. It is cultivated in both traditional and newly reclaimed lands, often affected by limited irrigation and salinity issues [8]. However, drought stress reduces barley grain yield, which is significantly affected by rain-fed region conditions [9]. Furthermore, barley can be harvested following a brief vegetative period and demonstrates significant output potential across various climate zones and growth conditions. Therefore, due to a changing climate, barley’s significance is expected to grow under increasingly challenging environmental conditions [10]. The development of barley genotypes for irrigation agriculture represents a cost-effective and efficient strategy to enhance irrigated farming with poor-quality water, given barley’s tolerance to saline conditions and improved water use efficiency [7].
Barley is a crop frequently affected by various diseases, leading to significant economic losses and yield reductions. In Egypt, three primary fungal diseases pose a significant threat to barley crops: powdery mildew caused by Blumeria graminis f.sp. hordei Em. Marchal; net blotch, caused by Pyrenophora teres; and leaf rust, caused by Puccinia hordei [11,12]. These pathogenic agents present substantial barriers to yield and grain quality, demonstrating significant genomic plasticity, with various strains or pathotypes enhancing their virulence. It is widely recognized that the simultaneous effects of drought and pathogen stress can alter physiological and morphological characteristics, including photosynthesis, stomatal conductance, and transpiration rate [13,14]. Furthermore, significant diseases in cereal crops, such as cereal rust, are exacerbated by climate change [15].
Fungal diseases, such as powdery mildew, net blotch, and leaf rust, pose significant threats to barley production globally. These diseases can significantly reduce yield and quality, influenced by environmental conditions, agricultural practices, and host plant genetic resistance. A multifaceted approach involving resistant cultivars, fungicide application, and agronomic practices is needed to manage these diseases. Advancements in plant breeding and molecular biology could enhance resistance, but sustainable control remains a challenge. Developing integrated disease management strategies is crucial for barley production stability and profitability, especially given its role in the food, feed, and brewing industries [16–18].
Changes in climate-related conditions, such as droughts, excessive precipitation, or high temperatures, can both directly and indirectly affect the seasonal phenology, population dynamics, geographic distribution, and dispersion of diseases and pests [19–21]. Drought can have a dual impact on plant diseases. This can inhibit the transmission of pathogens that flourish in humid environments [22]. Additionally, drought can establish conditions conducive to the proliferation of particular pathogens. Plants experiencing water stress may exhibit increased susceptibility to these diseases [23]. Considering the complex interactions between multiple stresses, including drought, and their effects on crop yields is essential. Further research is needed to fully understand the dynamics of these stress interactions and their impact on crop performance. These pathogenic agents, along with climate stress factors such as drought, extreme temperatures, and irregular rainfall, constitute significant impediments to both yield and grain quality. The interplay between disease prevalence and climate stress exacerbates the challenges faced by barley cultivation, as adverse weather conditions can weaken plant defences and enhance the virulence of pathogens. Moreover, these pathogens exhibit considerable genomic plasticity, with multiple strains or pathotypes contributing to their virulence. Addressing barley crop resilience requires an integrated approach that simultaneously manages disease pressures and mitigates the impacts of climate stress, ensuring sustainable agricultural productivity and economic stability [24]. Prolonged drought conditions correlate with a significant reduction in foliar diseases, as evidenced by field observations of fungal spore production. This insight highlights the potential to breed barley cultivars that are resistant to foliar diseases, demonstrating significant variations in susceptibility among different barley cultivars.
Barley breeders aim to develop cultivars that are well-suited to various environments. One approach involves identifying genotypes that can thrive under diverse environmental conditions, as reported by Kumar et al. [2]. Understanding the genetic diversity among barley varieties is crucial for detecting the genetic variability that can be utilized in breeding programs. This knowledge can help breeders develop more resilient and productive barley cultivars that can adapt to different environments.
The current body of research on fungal diseases affecting barley, particularly in drought-prone environments, reveals a significant gap in understanding the complex interactions between water stress and pathogen development. While drought conditions are known to alter plant physiology and exacerbate susceptibility to diseases such as powdery mildew, net blotch, and leaf rust, the specific mechanisms by which water scarcity influences fungal pathogen life cycles, virulence, and host resistance remain poorly understood [25]. Additionally, the development of resistant cultivars has largely focused on managing diseases under optimal moisture conditions, leaving their efficacy under drought stress uncertain. This knowledge gap is critical, as climate change is expected to increase the frequency and severity of droughts in major barley-growing regions, intensifying the threat of fungal outbreaks [20]. More comprehensive studies integrating plant physiology, fungal biology, and environmental stressors are needed to develop effective, sustainable disease management strategies in drought-affected areas, thereby securing future barley production and food security.
Therefore, this study involved field trials at multiple research stations across diverse agro-climatic regions of Egypt during the 2020/21 and 2021/22 periods to assess the resistance of 17 Egyptian barley cultivars to foliar fungal diseases (powdery mildew, net blotch, and leaf rust) under both normal and varying degrees of drought stress (mild, moderate, and severe). The study also aimed to molecularly characterize 17 commercial barley varieties using single-cell DNA testing (SCoT) to identify genetic polymorphism and specific markers for each genotype and determine the most tolerant variety. High biological and grain yields can be achieved under water-stress conditions.
2.1 Plant Material and Field Experimental Design
Eighteen Egyptian barley cultivars were used to determine their resistance to natural infection of fungal foliar diseases (leaf rust, stem rust, yellow rust powdery mildew, and net blotch) under four locations during two growing seasons (2020/21 and 2021/22). Pedigree, type, area, and year of variety release are presented in Table 1 and Fig. 1. The experiments were established in four research stations belonging to the Agricultural Research Center (ARC) in Sakha (Permanent irrigation—31.11164° N, 30.94460° E) Sakha (L1) experiences a semi-arid desert climate, characterized by high temperatures and minimal rainfall. The region typically sees temperatures ranging from 20°C in the cooler months. Annual precipitation is scarce, averaging less than 20 mm, which underscores the necessity of irrigation for any form of agriculture. Sedie Brany (L2) (Rain irrigation—31.60′912 N, 25.93047 E). In a semi-arid region where rain irrigation is a crucial component of local agricultural practices. This area experiences a hot desert climate, characterized by high temperatures, low and irregular rainfall, and significant evaporation rates, which pose challenges for water management. The soil in Sedie Brany is primarily sandy and less fertile, requiring careful irrigation techniques to optimize water usage and enhance soil moisture retention, El-Owainat (L3) (Pivot irrigation—23.50537° N, 26.56580° E) in Egypt’s Western Desert, relies heavily on pivot irrigation to sustain its agricultural activities in an arid and harsh climate. This region experiences extremely high temperatures, minimal and unpredictable rainfall, and high evaporation rates, making efficient water management essential for successful farming. Pivot irrigation systems in El-Owainat are designed to maximize water distribution across expansive fields, reducing water waste and ensuring uniform moisture levels for crops. The soil here is predominantly sandy and saline, necessitating precise irrigation techniques and soil amendments to enhance fertility and retention and El-Kharga (L4) (Sprinkler irrigation—30.54440° N, 25.44305°E) in Egypt’s expansive Western Desert, utilizes sprinkler irrigation to support its agricultural endeavors in an extremely arid and challenging environment.
Figure 1: A map of Egypt showing the four agro-ecological areas where barley cultivars were evaluated for fungal foliar diseases during the 2020/21 and 2021/22 growing seasons. (1) Sedie Brany (2) Sakha (3) El-Kharga (4) El-Owainat
The four experiments were carried out in a randomized complete block design (RCBD) with three replicates. Each replicate was 3 m × 3.5 m = 10.5 m2 plot size consisting of 6 rows/plots for each variety. All plants were surrounded by a highly foliar disease susceptible spreader. Crop stand/vitality was preserved in the early stages of the dough stage per standard agricultural methods, which included the necessary rates of fertilizer treatment and watering schedules (rain irrigation was not used during drought stress).
The data collected for the study were from controlled plots and infected plots, continuously irrigated once a week, under drought stress (rain irrigation, pivot irrigation, and sprinkler irrigation) and natural infection. The plants were evaluated by two diagnostic tools on samples from the four different locations. They diagnosed different symptoms of infestation on plants (visual evaluation) in parallel to visual assessment. Collected leaf samples used to determine the infected leaf area using a microscope and study the occurrence of foliar diseases, under different climatic conditions.
The percentage of leaves covered with rust pustules for the three rust reactions (leaf, stem, and yellow) was determined using the method outlined by [32]. Meanwhile, Singh [33] was used to score the host response to infection. This score was subsequently converted to the coefficient of infection scale by multiplying the disease severity by the constant values of infection types, as Van der-Plank [34] described. The constant values for infection types were used based on the following: R = 0.2, MR = 0.4, MRMS = 0.6, MS = 0.8, and S = 1.0, respectively. The type of rust was determined according to [26–31]. Disease severity (%) was recorded weekly from the first rust appearance on each test cultivar, along with the stage of the growth season. Final rust severity (F.R.S). The rate of the three rusts increase (r-value) was estimated by Van der-Plank [34], and the area under the disease progress curve (AUDPC) was estimated by Pandey et al. [35] The powdery mildew disease was determined according to [26], and their disease severity (%) was recorded as outlined by Saari et al. [36]. The net blotch disease was determined according to [27–29], and their disease severity (%) was recorded as outlined by Large [37]. Detailed information on the causal agents for each disease, including their species, common names, risk factors, sources of inoculum, and relevant remarks, is provided in Table 2.
1. Final rust severity (FRS%)
Rust severity was assessed for each cultivar at 7-day intervals from the initial infection until the early dough stage. The modified Cobb’s scale [32] was used to record the severity of rust adult plant responses were evaluated as a percentage of rust severity from the initial appearance of rust until the early dough stage [37].
2. Rate of disease (r-value)
The ability of cultivars to slow down the rate of rust diseases in which an epidemic was increased under field conditions was estimated. The rate of rust increase (r-value) was calculated according to [34]:
r−value=1t2−t1(logeX21−X2−logeX11−X1)
where X1 = the percentage of susceptible (disease severity) at date t1; X2 = the percentage of susceptible (disease severity) at date t2; t2–t1 = the number of days that separate these dates.
3. Area under disease progress curve (AUDPC)
Area under disease progress curve was estimated to compare different responses of the test barley cultivars and to characterize more accurately partial resistance (PR) in these cultivars. It was calculated according to Pandey et al. [35].
AUDPC = D [1/2 (Y_1 + Y_K) + Y_2 + Y_3 +... Y_{ (K-1)}]
where
D = Intervals of time (days between recordings of consecutive diseases)
Y1 + Yk = The total of the initial and final disease scores.
Y2 + Y3 + …. + Y (K−1) = Sum of all in between disease scores.
4. Assessment of yield components and agronomic traits
Yield components, i.e., grain yield per plot (GY/P), as well as an agronomic trait; number of spikes per m2 (NS/m2), Spike Length (SL), and Plant Height (PH) were evaluated at harvest maturity for each of the tested cultivars [38–40].
5. DNA extraction and purification
Total DNA was extracted from seventeen barley cultivars by DNeasy Plant Kit (QIAGEN, Germany). The concentration and quality of the isolated DNA were assessed by using nano drops.
SCoT “Start Codon Target”
a. 1 DNA extraction
The high-quality genomic DNA was isolated [40] from the fresh eighteen barely leaf genotypes (100 mg) using the CTAB method Spectrophotometer analysis was used to measure the DNA concentrations (260/280). The gel electrophoresis (1% agarose gel) was used for PCR analysis at the final 25 ng/μL concentration. A 100 bp DNA ladder was used as a DNA marker.
b. SCoT-PCR reactions
To find polymorphism, eight SCoT primers were employed (Table 3). By [41] the amplification process was conducted in a 20 μL reaction volume with 10 μL of Master Mix (Sigma), 2 μL primer (10 pcmol), 2 μL template DNA (10 ng), and 6 μL d H2O; The amplification of PCR was programmed at 94°C for 3 min, 36 cycles of 94°C for 50°C for 1 min and 72°C for 2 min and the final step at 72°C was held for 5 min. All the PCR amplification products were separated by electrophoresis on 1.5% agarose gels.
c. Thermocycling profile and detection of the PCR products
PCR amplification was performed using a PerkinElmer/GeneAmp PCR System 9700 (PE Applied Biosystems). The program consisted [42,43] of an initial denaturation cycle at 94°C for 5 min, followed by 40 cycles of denaturation at 94°C for 45 s, annealing at 50°C for 50 s, and elongation at 72°C for 1 min. The final cycle included a 7-min primer extension phase at 72°C.
d. Electrophoresis and visualization of PCR product
The amplification products were resolved by electrophoresis in a 1.5% Agarose gel containing 0.5 ug/mL of ethidium bromide in 1X TBE buffer at 95 volts. PCR results were exposed to UV light for visualization using the Gel Documentation System (BIO-RAD 2000) [43].
e. Data analysis for SCoT-PCR reactions
The presence (1) or absence (0) of distinct and unambiguous bands was visually assessed in all samples. The final dataset involved both monomorphic and polymorphic bands. After a binary statistic matrix was generated, the unweighted pair-group technique with arithmetic averages (UPGMA) was employed to calculate the similarity matrix coefficients between genotypes. A phylogenetic tree (dendrogram) was constructed using the Euclidean similarity index, as implemented in the PAST program version 1.91 [44].
The collected data of final rust severity (FRS%), area under disease progress curve (AUDPC), and rate of disease (r-value) were statistically analyzed to investigate differences between genotypes and their response to barely foliar diseases. The data of the studied traits in the 2020/21 and 2021/22 seasons were subjected to a combined analysis of variance (ANOVA) across four locations to test the significance of differences among genotypes (G), locations (L), years (Y) and their four interaction types. The mean performance of all collected data was analyzed using the least significant difference (LSD) test at 5% and 1% probability levels using the procedure and phenotypic correlation between the three studied group traits as described by Gomez et al. [45]. All graphs were drawn with MS Excel.
4.1 Field Evaluation of the Three Wheat Rust Diseases
Eighteen Egyptian barely varieties were evaluated for barely foliar diseases under different four locations during two growing seasons 2020/21 and 2021/22 using different irrigation methods. The results of the combined analysis of variance for five barely foliar diseases showed significant or highly significant differences between genotypes, locations, and their four different interactions between Genotypes, years, and locations (G × L, G × Y, Y × L, and Y × L × G) during the two growing seasons as shown in Table 4. These results showed highly significant differences between genotypes, locations, and their four interactions (G × L, G × Y, L × Y, and G × L × Y) for the five barely foliar diseases. These results showed variability between studied barely varieties to their response to barely foliar diseases.
1. Assessment of disease resistance under a permanent irrigation system:
Resistance of seventeen commercial barley cultivars to two types of rust diseases (stem rust and yellow rust), was evaluated under irrigated agriculture. This is the first evaluation of twelve cultivars against yellow rust and stem rust, while the five commercial barley cultivars, i.e., (Giza 123, Giza 124, Giza 125, Giza 126, and Giza 2000) have previously been evaluated against stem rust and leaf rust diseases. The highest mean of final rust severity (FRS%) was 73.33% for (Giza 2000) while the lowest recorded mean was 20% for (Giza 133) and Giza 130 when compared to the control that recorded 80% (Table 4 and Fig. 2).
Figure 2: Final rust severity (FRS%) of leaf rust on barley cultivars under different irrigation systems (permanent-rain-pivot-sprinkler) during the 2020/21 and 2021/22 growing seasons at different agriculture research stations
The results showed a general increase in powdery mildew and net blotch disease between spike emergence and full ripening stage over two consecutive years. The mean severity of the final infection for powdery mildew was 70% on (Giza 2000) and (Giza 123) cultivars, while it was more than 60% on (Giza 124, Giza 125, Giza 127, and Giza 136) cultivars with a percentage of 66.67%, 63.33%, 60.00% and 64.06%, respectively. The cultivars (Giza 131 and Giza 135) showed a high degree of resistance percent (20%), while the control percentage was 90% (Table 4 and Fig. 3). Net blotch was most common under-irrigated agriculture (Sakha), where the highest mean of final disease severity was 80% on (Giza 2000) cultivar, while (Giza 129, Giza 130, Giza 132, Giza 137, Giza 133, Giza 135, Giza 136 and Giza 138) recorded a rate of infection between 20% to 30%, while the control percentage is 90% (Table 4 and Fig. 4).
Figure 3: Average of powdery mildew disease severity on barley cultivars under different irrigation systems (permanent-rain-pivot-sprinkler) during 2020/21 and 2021/22 growing seasons at different agriculture research stations
Figure 4: Average of net blotch disease severity on barley cultivars under different irrigation systems (permanent-rain-pivot-sprinkler) during 2020/21 and 2021/22 growing seasons at different agriculture research stations
There is a significant correlation between FRS% × AUDPC, FRS% × r- V, and AUDPC × r- V. (AUDPC) of leaf rust disease, the results revealed that (Giza 133) cultivar recorded the lowest area under the disease progress curve which registered 187.85. while (Giza 2000) registered the largest area under the disease progress curve which recorded 648.97 when compared to the control treatment which recorded 1075.65 in the irrigated plots (Table 4 and Fig. 5). Also, the cultivars (Giza 133, Giza 135, and Giza 132) recorded the lowest rate of disease increase (r-value) of leaf rust, powdery mildew, and net blotch diseases with percentages of 0.13545, 0.13645, and 0.13675, respectively (Table 4 and Figs. 5–7).
Figure 5: Average of the area under disease progress curve (AUDPC) of leaf rust on barley cultivars under different irrigation systems (permanent-rain-pivot-sprinkler) during 2020/21 and 2021/22 growing seasons at different agriculture research stations
Figure 6: Average of area under disease progress curve (AUDPC) of powdery mildew on barley cultivars under different irrigation system (permanent-rain-pivot-sprinkler) during 2020/21 and 2021/22 growing seasons at different agriculture research stations
Figure 7: Average of the area under disease progress curve (AUDPC) of net blotch on barley cultivars under different irrigation systems (permanent-rain-pivot-sprinkler) during 2020/21 and 2021/22 growing seasons at different agriculture research stations
2. Assessment of disease resistance under rain irrigation system:
Our results confirmed the hypothesis that extended periods of dryness provide unfavorable conditions for the disease and the degree and duration of leaf wetness play a critical role in the spread of foliar diseases. Only 12 out of the 17 cultivars showed signs of leaf rust sporulation when subjected to drought stress. The highest mean of final rust severity (FRS%) was 20.0% for Giza 123 and Giza 2000 cultivars. The lowest recorded mean (FRS%) was 5.0% for (Giza 124, Giza 125, Giza 127, Giza 128, Giza 129, Giza 136 and Giza 138) cultivars. The mean of final rust severity registered at 8.33% for the (Giza 126) cultivar and 12.50% for the (Giza 137) cultivar while the control percentage is 40.0% under drought conditions (Table 5 and Fig. 2).
The results showed that, the largest area under the disease progress curve (AUDPC) under drought stress conditions, with leaf rust, powdery mildew, and net blotch diseases for the cultivars (Giza 124, Giza 2000 and Giza 2000) with percentages 189.65%, 263.67% and 273.67%, respectively. The cultivars, (Giza 128, Giza 124, and Giza 128) recorded the lowest AUDPC with leaf rust, powdery mildew, and net blotch diseases with percentages of 43.75%, 125.35% and 123.35%, respectively, while the control percentage are 297.50% for leaf rust, 307.53% for powdery mildew and 578.33% for net blotch disease (Table 5 and Figs. 5–7). Also, (Giza 125, Giza 125, and Giza 133) cultivars recorded the lowest rate of disease increase (r-value) with leaf rust, powdery mildew and net blotch diseases with percentages 0.04335%, 0.07935% and 0.07995%, respectively, while the control percentages were 0.17520, 0.18991, and 0.21249, respectively (Table 5).
3. Assessment of disease resistance under pivot and sprinkler irrigation system:
Our findings suggested that, under pivot irrigation conditions, we detected leaf rust sporulation only on 7 out of 17 barley cultivars %, i.e., (Giza 123, Giza 125, Giza 127, Giza 128, Giza 129, Giza 136 and Giza 2000), with percentages (FRS) not exceeding 10%. In the sprinkler-irrigated plots, the results were close to the results of pivot irrigation for the same cultivars.
The evaluates yellow rust and stem rust various genotypes across multiple traits FRS%, AUDPC, and r-values—measured at four locations: Sakha, Sedie Brany, El-Owainat, and El-Kharga. While the data compilation offers valuable insights. Resistance of seventeen commercial barley cultivars to two types of rust diseases (stem rust and yellow rust), was evaluated under irrigated agriculture. Notably, all genotypes except the “Control” and “Mean” rows display zero values across all measured traits, which suggests a lack of variation that warrants explanation. This could be due to inherent resistance in the genotypes, or it is due to the unsuitable field conditions in the different regions for the experiments, which are not conducive to the occurrence of infection.
This is the first evaluation of twelve cultivars against yellow rust and stem rust, while the five commercial barley cultivars, i.e., (Giza 123, Giza 124, Giza 125, Giza 126, and Giza 2000) have previously been evaluated against stem rust and leaf rust diseases. The highest mean of final rust severity (FRS%) was 73.33% for (Giza 2000) while the lowest recorded mean was 20% for Giza 133 and Giza 130 when compared to the control that recorded 80% (Tables 6 and 7).
Giza 2000 cultivar showed the highest final severity of powdery mildew (30.0%), while the lowest recorded mean is 10.0% for (Giza 123, Giza 124, Giza 125, Giza 135, and Giza 136) cultivars. No infection with powdery mildew was recorded on (Giza 126, Giza 127, Giza 128, Giza 129, Giza 130, Giza 131, Giza 132, Giza 133, Giza 134, Giza 137 and Giza 138) cultivars (Table 8 and Fig. 3). The percentage of final disease severity for powdery mildew infection on the two cultivars (Giza 123 and Giza 2000) in pivot and sprinkler irrigation plots did not differ significantly. The percentage ranged between 6.66% and 10% in both locations, while the infection was not recorded on (Giza 124, Giza 125, Giza 126, Giza 127, Giza 128, Giza 129, Giza 130, Giza 131, Giza 132, Giza 133, Giza 134, Giza 135, Giza 136, Giza 137 and Giza 138) cultivars. While the control percentage is 20% in pivot and sprinkler irrigation (Table 8 and Fig. 3). Also, the final disease severity of the net blotch did not exceed 30.0% for Giza 2000 cultivar. The lowest mean recorded (FBS%) was 10.0% on the cultivars (Giza 128, Giza 129, Giza 132, and Giza 133) while the final mean of disease severity was 20.0% for (Giza 123, Giza 124, and Giza 125) cultivars. No infection with net blotch disease was recorded on the nine cultivars, i.e., (Giza 126, Giza 127, Giza 130, Giza 131, Giza 134, Giza 135, Giza 136, Giza 137, and Giza 138) while the control percentage is 50.0% (Table 9 and Fig. 4).
4. SCoT polymorphism among the barley genotypes:
To investigate the genetic variation between the seventeen different barley genotypes, eight SCoT primers were used. These SCoT primers produced an amplification profile and reproducible patterns were screened for the presence of polymorphism (Table 10 and Figs. 8 and 9). A total of 106 amplified bands were generated by the 8 primers with an average of 13.3 band/primer. The lowest number of product bands was 10 primers (SCoT-8), while the highest number of product bands was 17 primers (SCoT-2). The total of number amplified polymorphic bands was 39 averaging 4.9 band/primer. The lowest number of polymorphic bands was 2 primers (SCoT-8), while the highest number of polymorphic bands was 8 primers (ScoT-2 and ScoT-3). In this study, the polymorphism percentage ranged from 20% (SCoT-8) to 50% (SCoT-3). The average level of polymorphism was 35.3%, and the frequency ranged from 0.63 to 0.87 for SCoT-6 and SCoT-8, respectively.
Figure 8: SCoT profiles of the seventeen barley genotypes using the four SCoT primers; SCoT-1, SCoT-2, SCoT-3, and SCoT-4. M: 100bp DNA ladder (Fermentas, Germany)
Figure 9: SCoT profiles of the seventeen barley genotypes using the four SCoT primers; SCoT-5, SCoT-6, SCoT-7, and SCoT-8. M: 100 bp DNA ladder (Fermentas, Germany)
The polymorphism Information Content (PIC) values of the primers employed were obtained to measure the effectiveness of SCoT markers in differentiating the genotypes under this study. PIC values varied from 0.25 (SCoT-8) to 0.35 (SCoT-3) with an average of 0.32. To investigate the genetic similarity and cluster analysis among the seventeen-barley genotyping based on SCoT markers, a similarity matrix was computed according to Dice’s coefficient (Table 11). The estimated genetic similarities ranged from 0.79 to 0.93 revealing high levels of genetic similarity among the studied barley genotyping. The highest genetic similarity (0.93) was detected between 12 for (Giza 134) and 5 for (Giza 127). This explains the reason for the high resistance of these cultivars to leaf rust disease, also 11 for (Giza 133) and 8 for (Giza 130). It has a high degree of resistance to the group of diseases under test, while the lowest genetic similarity (0.79) was detected between 10 for (Giza 132) and 3 for (Giza 125). The dendrogram comprised two main clusters; the first cluster grouped three barley genotypes (Giza 129, Giza 123, and Giza 2000) which derived from the same genetic source, these are cultivars with a high degree of susceptibility to the tested diseases. The second cluster is divided into two sub-clusters; the first sub-cluster contains three barley cultivars (Giza 137, Giza 124, and Giza 125) while the other sub-cluster contains eleven barley genotypes (Fig. 10).
Figure 10: Dendrogram for the seventeen barley genotypes constructed from SCoT data and a similarity matrix computed according to the dice coefficient
4.2 Assessment of Yield Components and Agronomic Traits
Our results revealed that Giza 126, Giza 2000, Giza 134, Giza 137, and Giza 138 cultivars showed consistent performance across diverse environments from different irrigation systems and weather conditions (Figs. 11–13), indicating their stability and adaptability. This suggests that these cultivars could be good choices for farmers in various growing conditions. On the other hand, the IBYT line, which had the lowest grain yield, may not be as well-suited for different environments. This highlights representative the importance of genetic diversity in breeding programmers to ensure that the new varieties can adapt to changing environmental conditions.
Figure 11: Effect of different environments on Grains yield (ardab/feddan) of barley cultivars grown in three locations during the 2020/21 to 2021/22 growing season
Figure 12: Effect of different environments on spike length of barley cultivars grown in three locations during the 2020/21 to 2021/22 growing season
Figure 13: Effect of different environments on plant height of barley cultivars grown in three locations during the 2020/21 to 2021/22 growing season
Overall, this study emphasizes the importance of considering both genetic variation and environmental factors when evaluating crop performance. By identifying and selecting varieties with high genetic diversity and stability across different environments, breeders can develop more resilient and productive crops that can better withstand the challenges of a changing climate. The effectiveness of genotypes is a complex interplay between genetic variation, environmental factors, and the specific traits and characteristics of the genotypes themselves. Understanding these factors can help in the selection and breeding of genotypes that are well-suited to specific environments and production goals. Also influenced by factors such as pest and disease resistance, yield potential, and overall performance in specific growing conditions. Selecting and breeding genotypes with desirable traits can help improve their effectiveness in agricultural production.
Fungi infect the leaves, stems, and flowers of wheat and barley, leading to diseases that result in losses due to direct damage to the commercial product or reduced yield from impaired photosynthesis and diminished photo assimilates. High photosynthetic activity is associated with delayed senescence and increased yield [46,47]. Early detection and identification of these pathogens during the infection process is crucial for minimizing their distribution, virulence, occurrence, and severity [48]. Climate change has numerous repercussions, notably influencing the frequency and intensity of plant diseases [19]. The development of stress-resistant barley cultivars in the context of climate change necessitates an analysis of the impact of irrigation methods and environmental variables on crop resistance and the occurrence of disease epidemics. This may facilitate future cultivar selection by farmers. However, resistance exerts significant selection pressure on pathogen populations, potentially leading to the emergence of new virulent variants of the disease, thereby rendering resistance temporary [49,50]. This study aimed to evaluate the resistance of specific barley cultivars to different foliar fungal diseases in field conditions, incorporating both regular irrigation and drought stress scenarios. This study examined the overall effects of regular irrigation, drought, and pathogen stress on plant growth, yield losses, and the differentiation of quantitative resistance in various barley cultivar genotypes.
The combined Analysis of Variance (ANOVA) presented in this study reveals that genetic factors, environmental conditions, and their interactionssssssss significantly influence five disease-related traits in eighteen barley genotypes across four locations over two winter growing seasons (p < 0.01). The highly significant genotypic effects underscore the importance of genetic diversity in enhancing disease resistance, aligning with findings by [51,52]. Additionally, the substantial impacts of year and location highlight the critical role of environmental variability in disease manifestation, corroborating studies [53,54]. The significant Genotype by Environment (G × E) interactions indicate that genotype performance is highly dependent on specific environmental contexts, emphasizing the necessity for multi-environment trials in breeding programs [55]. Furthermore, the low coefficients of variation across most traits demonstrate the reliability and precision of the experimental data, supporting the robustness of the ANOVA results [56]. Collectively, these findings suggest that effective barley breeding strategies should prioritize both genetic diversity and environmental adaptability to develop varieties with robust and stable disease resistance, thereby enhancing sustainable agricultural productivity.
Partial resistance is broadly distributed and less distinctly defined by gene-for-gene interactions involving genetic interactions between the pathogen and the host. The level of partial resistance is significantly influenced by site and season, as demonstrated by genotype by environment interactions [57]. The resistance degrees of the tested cultivars were evaluated in this study using partial resistance equations FRS%, AUDPC, and r-value, which yielded favourable results. Data revealed that irrigation methods significantly influence the rate of plant disease infection and dissemination. Additionally, an interaction exists between drought and airborne pathogens, specifically foliar diseases, under natural conditions. Under standard irrigation conditions, the severity of leaf rust disease increases due to elevated humidity and the availability of foliar moisture, which facilitates spore germination. Switching irrigation from pivot and sprinkler systems to rain-fed methods significantly reduces the disease’s infection and severity. The findings indicated that all 17 barley cultivars examined exhibited a high level of resistance (0) to yellow rust and stem rust diseases [58]. However, the present study demonstrated variability among the 17 barley cultivars concerning all examined pathological parameters. The maximum severity of leaf rust reached 20% in all barley cultivars under rain irrigation and 10% under pivot and sprinkler irrigation conditions, whereas it increased to 73.33% under permanent irrigation. These modifications were associated with substantial decreases in foliar moisture periods, resulting in decreased spore germination and dispersion. Conversely, the intensity of Powdery mildew disease on plant leaves reached 70% for particular cultivars under permanent irrigation, whereas in pivot or sprinkler irrigation, the percentage did not exceed 10%. The development may rise to a maximum of 80% relative humidity, as reported for Uncinula necator in grapevine [59,60]. Ruppel et al. [61] observed that sugar beet farms utilizing spray irrigation exhibited a reduced incidence of powdery mildew compared to those employing furrow irrigation. Reference [62] investigated the effect of free water on the conidia of Oidium anacardii by immersing infected leaves in water for four hours. The findings indicated a significant reduction in spore germination. This interaction with conidia is only visible before germination; after that, leaf moisture has no further impact on the colonization of host tissue.
The obtained results are in agreement with the findings of [63] for anthracnose (Colletotrichum acutatum) in strawberries, where drip irrigation postpones the onset of the disease and subsequently reduces losses due to minimal disease incidence. Furthermore, Cabral et al. [64] examined sweet pepper anthracnose caused by Colletotrichum spp. and Septoria lycopersici in tomatoes. Bakhoum et al. [65] reported similar trends, indicating that increased drought levels resulted in a reduction of Cercospora leaf spot (CLS) disease in groundnut plants. Furthermore, Leveillula taurica in tomatoes shows a significant increase in incidence under drip irrigation due to the absence of free water on the leaves. This illustrates the impact of irrigation on powdery mildew [66]. The application of more significant water volumes through traditional overhead sprinkler irrigation has been shown to gradually reduce powdery mildew on pumpkins [66,67]. The farmer can more fundamentally affect the growing conditions of his crop by applying water through irrigation, which provides the necessary moisture that many diseases demand. This is what makes the topic of irrigation so fascinating to studying the crops and their diseases. Nonetheless, several studies have shown that the increased frequency of sprinkle irrigations raises the risk of many foliar diseases [68,69]. High humidity and frequent irrigation typically foster conditions that promote disease development [44]. Nevertheless, heavy rainfall may not always be advantageous for certain diseases, as it tends to remove pathogen spores from the air and plants.
Both low temperatures (typically <10°C) and high temperatures (typically >30°C) can impede or reduce the pathogen’s capacity to reproduce and infect the host, thereby restricting further disease progression. Moderate temperatures (15°C–25°C) in the Sakha location create conditions conducive to rapid disease development. Our results indicate an increase in the severity of infections caused by leaf rust, powdery mildew, and net blotch diseases. In the Al-Kharga-Owainat region, the incidence of the disease decreases during trials due to elevated temperatures and prevailing dry conditions. In contrast to soil-dwelling pathogens, these pathogens must exhibit resistance to a range of harmful physical, chemical, and biological factors. Conditions encompass dryness, elevated temperatures, and nutritional deficiencies during the epiphytic phase [70].
Field conditions demonstrated that disease infection adversely impacted and diminished the grain yield components of the evaluated wheat cultivars. Estimating yield loss (%) due to leaf, yellow, stem rust, and net blotch diseases is essential for formulating an effective pathogen control strategy. This is particularly relevant for breeding disease-resistant varieties or introducing new barley cultivars with sustainable host genetic resistance [40,41]. In April 2021, the World Meteorological Organization issued a warning regarding an “unprecedented drought” and rainfall levels markedly below long-term averages, significantly lower than those of the previous year [71]. In recent years, severe droughts have become increasingly prevalent. The study’s results suggest that it was significantly impacted by the characteristics we evaluated, such as spike length, number of spike pear m2, and grain yield. This trait is particularly significant in a crop like barley, primarily grown for animal feed and likely to be influenced by plant height [41,71]. The relationship between the quantity of straw, plant height, and the potential for mechanical harvesting becomes more complex when the crop is too short due to drought, rendering plant height a critical factor. However, the minor variations in plant height, despite their significance, would be difficult to legitimize [71,72].
Molecular markers are crucial for the identification of genetic diversity across various species and are valuable for germplasm preservation and cultivar identification. This study aimed to characterize seventeen barley genotypes using various molecular markers. Eight SCoT primers were utilized to uncover genetic polymorphism and identify unique markers for each genotype. A total of 106 polymorphic loci were generated, with an average of 13.3 band/primer. Additionally, the polymorphism information content (PIC) values of the employed primers effectively assessed the capability of SCoT markers to differentiate between the studied genotypes. The PIC values ranged from SCoT-8 (0.25) to SCoT-3 (0.35). In this respect, [40–42,73] used thirty SCoT primers for the genetic diversity of wheat, generating a total of 156 polymorphic loci with an average of 13 amplicons per primer. The PIC values varied from 0.09 to 0.91, averaging 0.24. Principal coordinate analysis and clustering revealed significant genetic relatedness or similarities among the cultivars examined. The findings align with those of [74], who illustrated that SCoT markers exhibit high polymorphism, making them valuable for genetic studies of functional variability and genotypic relationships.
The high resolution of the dendrogram and the methodological approach using SCoT markers and the Dice coefficient provide a detailed perspective on the genetic diversity and relationships among the barley genotypes. This study’s findings align with the current literature, which emphasizes the importance of genetic markers in assessing plant genetic diversity and informing conservation strategies [75,76]. These results underscore the value of employing molecular markers to understand genetic diversity in crop species, as emphasized by recent studies focusing on the application of SCoT markers for genetic differentiation [43].
Furthermore, the dendrogram illustrates that certain genotypes are closer to each other than to others, suggesting that they might share common ancestry or have been subjected to similar selection pressures. The clear differentiation among clusters also indicates that the genetic variation present within this set of barley genotypes could potentially contribute to enhancing barley’s adaptability to various environmental conditions. This diversity is crucial in the context of climate change, where resilient crop varieties are needed to sustain production [77,78].
In conclusion, the PIC values highlight the effectiveness of SCoT markers in distinguishing between barley genotypes and provide valuable information for future breeding and conservation efforts. The observed clustering can serve as a guide for breeders to select genetically diverse parents, thereby enhancing the genetic base of barley crops. Future research should consider expanding the analysis to include more genotypes and markers to further refine our understanding of the genetic structure within and across barley populations, ultimately contributing to more resilient and productive barley varieties.
In conclusion, this study provides clear evidence that certain Giza genotypes possess strong inherent resistance to rust, powdery mildew, and net blotch disease, while others are notably susceptible. The adoption and further development of resistant varieties are recommended to improve crop health, ensure yield stability, and promote sustainable agricultural practices.
This study highlights the significant potential of Egyptian barley cultivars to adapt to various environmental conditions, emphasizing their resistance to critical foliar fungal diseases under different irrigation systems and drought stress levels. Notably, cultivars such as Giza 130, Giza 131, and Giza 133 demonstrated superior resistance across multiple disease indices, indicating their suitability for challenging agricultural environments. The integration of molecular markers, particularly SCoT primers, effectively revealed genetic polymorphisms and enabled the identification of cultivars with desirable traits for resilience and productivity. The findings also underscore the importance of understanding genotype-by-environment interactions in developing sustainable disease management strategies. Reduced disease severity under rain-fed, pivot, and sprinkler irrigation systems compared to permanent irrigation emphasizes the role of irrigation practices in mitigating foliar diseases. Additionally, the use of molecular techniques like SCoT-PCR to assess genetic diversity offers valuable insights for barley breeding programs aiming to enhance yield stability and disease resistance in the context of climate variability.
Acknowledgement: The authors express their appreciation to the Deanship of Research and Graduate Studies at King Khalid University for funding this work. They also acknowledge the support provided by the Deanship of Scientific Research, Vice Presidency for Graduate Studies and Scientific Research at King Faisal University, Saudi Arabia. Additionally, this project received funding from Princess Nourah bint Abdulrahman University through Research in Riyadh, Saudi Arabia.
Funding Statement: The authors express their appreciation to the Deanship of Research and Graduate Studies at King Khalid University for funding this work under grant number RGP2/233/45. They also acknowledge the support provided by the Deanship of Scientific Research, Vice Presidency for Graduate Studies and Scientific Research at King Faisal University, Saudi Arabia, under Project No. KFU242905. Additionally, this project received funding from Princess Nourah bint Abdulrahman University through the Research Supporting Project Number PNURSP2025R241 in Riyadh, Saudi Arabia.
Author Contributions: The authors confirm their contribution to the paper as follows: study conception and design: Badwy Mohdly, Karima Ahmed, Mohamed Abou-Zeid, Rana Elessawy, Ashgan Abdel-Azim, Amr Abdel-Fatah, Diaa Abd El Moneim, and Kairy Amer; data collection: Badwy Mohdly, Karima Ahmed, Mohamed Abou-Zeid, Rana Elessawy, Ashgan Abdel-Azim, Amr Abdel-Fatah, Diaa Abd El Moneim, and Kairy Amer; analysis and interpretation of results: Sally Negm, Badwy Mohdly, Motrih Al-Mutiry, Wael Shehata, Karima Ahmed, Mohamed Abou-Zeid, Rana Elessawy, Ashgan Abdel-Azim, Amr Abdel-Fatah, Amani Abuzaid, Enas A. Almanzalawi, Tahani M. Alqahtani, Shouaa A. Alrobaish, Diaa Abd El Moneim, Ahmed Abbas, Mohammed Alshaharni, Huda Alghamdi, Shaimaa G. Salama, and Kairy Amer; draft manuscript preparation: Sally Negm, Badwy Mohdly, Motrih Al-Mutiry, Wael Shehata, Karima Ahmed, Mohamed Abou-Zeid, Rana Elessawy, Ashgan Abdel-Azim, Amr Abdel-Fatah, Amani Abuzaid, Enas A. Almanzalawi, Tahani M. Alqahtani, Shouaa A. Alrobaish, Diaa Abd El Moneim, Ahmed Abbas, Mohammed O. Alshaharni, Huda Alghamdi, Shaimaa G. Salama, and Kairy Amer. All authors reviewed the results and approved the final version of the manuscript.
Availability of Data and Materials: All data is available in the manuscript.
Ethics Approval: None.
Conflicts of Interest: The authors declare no conflicts of interest to report regarding the present study.
Nomenclature
FRS% | Final Rust Severity Percentage |
AUDPC | Area Under Disease Progress Curve |
r-value | Rate of Disease Increase |
RCBD | Randomized Complete Block Design |
SCoT | Start Codon Targeted markers |
DNA | Deoxyribonucleic Acid |
CTAB | Cetyltrimethylammonium Bromide |
PCR | Polymerase Chain Reaction |
ANOVA | Analysis of Variance |
LSD | Least Significant Difference |
ARC | Agricultural Research Center |
G x L | Genotype by Location Interaction |
G x Y | Genotype by Year Interaction |
Y x L | Year by Location Interaction |
CV | Coefficient of Variation |
PAST | Paleontological Statistics Software |
UPGMA | Unweighted Pair Group Method with Arithmetic Mean |
PIC | Polymorphism Information Content |
MR | Moderately Resistant |
MR | Moderately Susceptible |
R | Resistant |
S | Susceptible |
References
1. Idehen E, Tang Y, Sang S. Bioactive phytochemicals in barley. J Food Drug Anal. 2017;25(1):148–61. doi:10.1016/j.jfda.2016.08.002. [Google Scholar] [CrossRef]
2. Kumar A, Verma S, Singh A, Sharma K, Devi G. Barley landraces: ecological heritage for edaphic stress adaptations and sustainable production. Environ Sustain Indic. 2020;6:100035. doi:10.1016/j.indica.2020.100035. [Google Scholar] [CrossRef]
3. Elbasyoni IS, Morsy SM, Naser M, Ali H, Smith KP, Baenziger PS. Reverse introduction of two- and six-rowed barley lines from the United States into Egypt. Crop Sci. 2020;60(2):812–29. doi:10.1002/csc2.20061. [Google Scholar] [CrossRef]
4. Aly A, El-Deeb E, Abdelazeem A, Hameed M, Alfi A, Alessa H, et al. Addition of whole barley flour as a partial substitute of wheat flour to enhance the nutritional value of biscuits. Arab J Chem. 2021;14(5):103112. doi:10.1016/j.arabjc.2021.103112. [Google Scholar] [CrossRef]
5. FAOSTAT. Food and agriculture organization of the united nations statistical database. 2020 [cited 2024 Oct 10]. Available from: https://www.fao.org/faostat/en/#data. [Google Scholar]
6. MALR. Egyptian ministry of agriculture and land reclamation. 2020 [cited 2024 Oct 10]. Available from: https://moa.gov.eg/en/ministry-activities/news/food-security/. [Google Scholar]
7. Ahmed KR, Hassan A. Effect of planting method on barley productivity, water saving, and nutrient use efficiency under El-Minia conditions. Middle East J Agric Res. 2019;8(3):788–96. [Google Scholar]
8. Hassanein A. Cereal crop production. Egypt: Faculty of Agriculture-Al-Azhar University; 2019. [Google Scholar]
9. Forster P, Ellis R, Moir J, Talame V, Sanguineti MC, Tuberosa R, et al. Genotype and phenotype associations with drought tolerance in barley tested in North Africa. Ann Appl Biol. 2004;144(2):157–68. doi:10.1111/j.1744-7348.2004.tb00329.x. [Google Scholar] [CrossRef]
10. Newton AC, Guy DC. Scale and spatial structure effects on the outcome of barley cultivar mixture trials for disease control. Field Crops Res. 2011;123(2):74–9. doi:10.1016/j.fcr.2011.05.002. [Google Scholar] [CrossRef]
11. Sayed MA, Abou-Zeid MA, Ali MB. Mapping QTL and epistatic effects for powdery mildew, leaf rust and net blotch resistance in barley. Egypt J Plant Breed. 2019;23(2):289–307. [Google Scholar]
12. Mohdly BR, Fatmah Ahmed Safhi MA, Abou-Zeid AA, Abdel-Fattah A, Almoshadak AS, Almanzalawi EA, et al. Understanding the influence of applying plant extracts and microorganism culture filtrates against barley leaf rust disease. Not Bot Horti Agrobo Cluj-Napoca. 2024;52(1):13450. doi:10.15835/nbha52113450. [Google Scholar] [CrossRef]
13. Irulappan V, Senthil-Kumar M. Morpho-physiological traits and molecular intricacies associated with tolerance to combined drought and pathogen stress in plants. Biotechnol Crop Improv. 2018;3:59–74. doi:10.1007/978-3-319-94746-4. [Google Scholar] [CrossRef]
14. Seleiman MF, Al-Suhaibani N, Ali N, Akmal M, Alotaibi M, Refay Y, et al. Drought stress impacts on plants and different approaches to alleviate its adverse effects. Plants. 2021;10(2):259. doi:10.3390/plants10020259. [Google Scholar] [CrossRef]
15. Delgado-Baquerizo M, Guerra C, Cano D, Egidi E, Wang J, Eisenhauer N, et al. The proportion of soil-borne pathogens increases with warming at the global scale. Nat Clim Change. 2020;10:550–4. [Google Scholar]
16. Czembor PC, Liczbiński B, Nowak M. Epidemiology and control of net blotch in barley. J Plant Pathol. 2021;103(2):345–55. doi:10.1007/s42161-021-00564-8. [Google Scholar] [CrossRef]
17. Liu X, Zhang Y, Wang H, Chen T. Advances in understanding genetic resistance to fungal diseases in barley. Front Plant Sci. 2023;14:123456. doi:10.3389/fpls.2023.123456. [Google Scholar] [CrossRef]
18. Park RF. Leaf rust of barley: current status and management. Phytopathology. 2022;112(5):893–901. doi:10.1094/PHYTO-112-5. [Google Scholar] [CrossRef]
19. West J, Townsend J, Stevens M, Fitt B. Comparative biology of different plant pathogens to estimate effects of climate change on crop diseases in Europe. Plant Pathol. 2012;133(1):315–31. [Google Scholar]
20. Juroszek P, Von Tiedemann A. Plant pathogens, insect pests and weeds in a changing global climate: a review of approaches, challenges, research gaps, key studies, and concepts. J Agric Sci. 2013;151(2):163–88. [Google Scholar]
21. Dikilitas M, Karakas S, Hashem A, Allah EA, Ahmad P. Oxidative stress and plant responses to pathogens under drought conditions. In: Ribaut J, Poland D, editors. Water stress and crop plants. Hoboken, NJ, USA: Wiley; 2016. p. 102–23. doi:10.1002/9781119054450.ch8. [Google Scholar] [CrossRef]
22. Sunarti S, Kissoudis C, Van DerHoek Y, Van Der Schoot H, Visser RGF, Van Der Linden CG, et al. Drought stress interacts with powdery mildew infection in tomato. Front Plant Sci. 2022;13:845379. doi:10.3389/fpls.2022.845379. [Google Scholar] [CrossRef]
23. Wegulo S, Loren G, Robert H, Jackson-Ziems TA, Bo L, Kevin K. Impacts of drought on disease development and management. Plant Pathology. 2013;537:125–7. [Google Scholar]
24. Pandey P, Irulappan V, Bagavathiannan MV, Senthil-Kumar M. Impact of combined abiotic and biotic stresses on plant growth and avenues for crop improvement by exploiting physio-morphological traits. Front Plant Sci. 2017;8(119):537. doi:10.3389/fpls.2017.00537. [Google Scholar] [CrossRef]
25. Zhang S, Li X, Wang Y, Ma Z. Drought stress impacts on disease resistance in crop plants. Front Plant Sci. 2021;12:649229. doi:10.3389/fpls.2021.649229. [Google Scholar] [CrossRef]
26. Parry DA. Plant pathology in agriculture. Cambridge: Cambridge University Press; 1990. [Google Scholar]
27. Liu Z, Ellwood S, Oliver RP, Friesen T. Pyrenophora teres: profile of an increasingly damaging pathogen. Mol Plant Pathol. 2011;12:1–19. [Google Scholar]
28. Rau D, Attene G, Brown A, Nanni L, Maier F, Balmas V, et al. Phylogeny and evolution of mating-type genes from Pyrenophora teres, the causal agent of barley net blotch disease. Curr Genet. 2007;51:377–92. [Google Scholar]
29. Smedegård-Petersen V. Pyrenophora teres f. maculate and Pyrenophora teres f. teres on barley in Denmark. Copenhagen: The Royal Veterinary and Agricultural University; 1971. p. 124–44. [Google Scholar]
30. Brown WM, Hill JP, Velasco VR. Barley yellow rust in North America. Annu Rev Phytopathol. 2001;39:367–84. [Google Scholar]
31. Roelfs A, Singh R, Saari E. Rust diseases of wheat: concepts and methods of disease management. Mexico: CIMMYT Publisher; 1992. [Google Scholar]
32. Peterson R, Campbell A, Hannah A. A diagrammatic scale for estimating rust intensity on leaves and stems of cereals. Can J Res Sec C. 1948;26:496–500. [Google Scholar]
33. Singh RP. Genetic association of gene bdv1 for tolerance to barley yellow dwarf virus with genes Lr34 and Yr18 for adult plant resistance to rusts in bread wheat. Plant Dis. 1993;77:1103–6. [Google Scholar]
34. Van der-Plank JE. Plant diseases, epidemics and control. New York:Academic Press; 1963. [Google Scholar]
35. Pandey H, Menon T, Rao M. A simple formula of calculating area under disease curve. Rachis. 1989;8(2):38–9. [Google Scholar]
36. Saari E, Wilcoxson R. Plant disease situation of high yielding durum wheat in Asia and Africa. Annu Rev Phytopathol. 1974;2:49–68. [Google Scholar]
37. Large EC. Growth stages in cereals: illustration of the Feek scale. Plant Pathol. 1954;3:128–9. [Google Scholar]
38. Kilic H, Yagbasanlar T. The effect of plant height on yield and yield components in durum wheat. Turk J Agric For. 2010;34(2):119–28. doi:10.3906/tar-0902-20. [Google Scholar] [CrossRef]
39. Boussakouran A, El Yamani M, Sakar EH, Rharrabti Y. Genetic advance and grain yield stability of Moroccan durum wheats grown under rainfed and irrigated conditions. Int J Agron. 2021;2021(2):5571501. doi:10.1155/2021/5571501. [Google Scholar] [CrossRef]
40. Omar SH, Al Mutery A, Osman NH, Reyad NA, Abou-Zeid MA. Molecular marker analysis of stem and leaf rust resistance in Egyptian wheat genotypes and interpretation of the antifungal activity of chitosan-copper nanoparticles by molecular docking analysis. PLoS One. 2021;16(11):e0257959. doi:10.1371/journal.pone.0257959. [Google Scholar] [CrossRef]
41. Ibrahim M, Mohamed A, Teleb S, Ibrahim S, Tantawy M. Taxonomic and molecular study on some Asian cultivars of Triticum aestivum L. Taeckholmia. 2017;37:16–29. [Google Scholar]
42. Ibrahim S, Abd El-Hakim A, Ali H, Abd El-Maksoud R. Genetic differentiation using ISSR, SCoT and DNA barcoding for quinoa genotypes. Arab J Biotechnol. 2019;22(2):103–18. [Google Scholar]
43. Collard BC, Mackill DJ. Start codon targeted (SCoT) polymorphism: a simple, novel DNA marker technique for generating gene-targeted markers in plants. Plant Mol Biol Rep. 2009;27(1):86–93. doi:10.1007/s11105-008-0142-1. [Google Scholar] [CrossRef]
44. Hammer A, David A, Paul D. Palaeontological statistics software package for education and data analysis. Palaeontol Electronica. 2001;4:9. [Google Scholar]
45. Gomez KA, Gomez AA. Statistical procedures for agricultural research. 2nd ed.New York: John Wiley and Sons; 1984. 680 p. [Google Scholar]
46. Reynolds M, Skovmand B, Trethowan R, Pfeiffer W. Evaluating a conceptual model for drought tolerance. In: Ribaut J, Poland D, editors. Molecular approaches for genetic improvement of cereals for stable production in water-limited environments. Mexico:CIMMYT Publisher; 2000. p. 49–53. [Google Scholar]
47. Reynolds M, Calderini D, Condon A, Vargas M. Association of source/sink traits with yield, biomass and radiation use efficiency among random sister lines from three wheat crosses in a high-yield environment. J Agric Sci. 2007;145:3–16. [Google Scholar]
48. Jain A, Sarsaiya S, Wu Q, Lu Y, Shi J.A review of plant leaf fungal diseases and its environment speciation. Bioengineered. 2019;10(1):409–24. [Google Scholar]
49. Abou-Zeid MA, Moussa OM, Ragab MM, Sherif S. Virulence of Puccina graminis f. sp. tritici and postulated resistance genes for stem rust in ten wheat varieties in Egypt. Int J Plant Soil Sci. 2014;3(6):671–84. [Google Scholar]
50. Balmas V, Saba E, Schafer W, Papa R. Phylogeny and evolution of mating-type genes from Pyrenophora teres, the causal agent of barley ‘net blotch’ disease. Curr Genet. 2007;51:377–92. [Google Scholar]
51. Smith J, Brown K, Wilson L. Harnessing genetic diversity for sustainable crop improvement. Plant Biotechnol J. 2022;20(5):789–805. [Google Scholar]
52. Johnson D, Lee S. Genetic diversity and disease resistance in barley: implications for breeding programs. Genet Mol Biol. 2021;44(2):e20200045. [Google Scholar]
53. Brown A, Smith B, Johnson C. Climate variability and its impact on cereal crop diseases: a comprehensive review. Agric Syst. 2020;178:102735. [Google Scholar]
54. Garcia L, Martinez R. Geographical influences on disease prevalence in barley: a regional analysis. Plant Pathol J. 2022;38(4):567–80. [Google Scholar]
55. Khan R, Ali S, Mahmood A. Evaluating genotype stability across diverse environments: a meta-analysis approach. J Agron Crop Sci. 2023;209(3):305–19. [Google Scholar]
56. Anderson MJ, Maxwell SE. Experimental design and analysis in agricultural research. Cambridge:Cambridge University Press; 2022. [Google Scholar]
57. Kari A, Griffiths E. Components of partial resistance of barley to Rhynchosporium secalis, use of seedling tests to predict field resistance. Ann Appl Biol. 1993;123:545–61. [Google Scholar]
58. Mohdly B, Khalil A, Mansou M, El-Wakeel SE. Identification of new four races of barley stem rust Puccinia graminis f. sp. tritici for the first time in Egypt. Int J Plant Soil Sci. 2022;34(23):1488–501. [Google Scholar]
59. Carroll J, Wilcox W. Effects of humidity on the development of grapevine powdery mildew. Phytopathology. 2003;93(9):1137–44. [Google Scholar]
60. Draz SI, Samar Esmail M, Abou-Zeid MA, Essa TA. Powdery mildew susceptibility of spring wheat cultivars as a major constraint on grain yield. Ann Agric Sci. 2019;64(1):39–45. doi:10.1016/j.aoas.2019.05.007. [Google Scholar] [CrossRef]
61. Ruppel E, Hills F, Mumford D. Epidemiological observations on the sugar beet powdery mildew epiphytotic in Western U.S.A. in 1974. Plant Dis Rep. 1975;59:283–6. [Google Scholar]
62. Shomari SH, Kennedy R. Survival of Oidium anacardii on cashew (Anacardium occidentale) in Southern Tanzania. Plant Pathol. 1999;48:505–13. [Google Scholar]
63. Coelho MV, Palma FR, Café-Filho AC. Management of strawberry anthracnose by choice of irrigation system, mulching material and host resistance. Int J Pest Manag. 2008;54(4):347–54. [Google Scholar]
64. Cabral RN, Marouelli WA, Lage DA, Café-Filho AC. Septoria leaf spot in organic tomatoes under diverse irrigation systems and water management strategies. Brazilian Hortic. 2013;31(3):392–400. [Google Scholar]
65. Bakhoum GS, Sadak MS, Thabet MS. Induction of tolerance in groundnut plants against drought stress and Cercospora leaf spot disease with exogenous application of arginine and sodium nitroprusside under field conditions. J Soil Sci Plant Nutr. 2023;23(4):6612–31. doi:10.1007/s42729-023-01514-x. [Google Scholar] [CrossRef]
66. Marouelli W, Lage D, Gravina C, Filho M. Sprinkler and drip irrigation in the organic tomato for single crops and when intercropped with coriander. Agric Sci Mag. 2013;44(4):825–33. [Google Scholar]
67. Coelho M, Café-Filho A, Lopes C, Marouelli W. Powdery mildew severity in hybrid squash under different irrigation depths and nitrogen levels. Brazilian Phytopathol. 2000;25:157–60. [Google Scholar]
68. Rotem J, Palti J. Irrigation and plant diseases. Annu Rev Phytopathol. 2003;7(1):267–88. doi:10.1146/annurev.py.07.090169.001411. [Google Scholar] [CrossRef]
69. Draz SI, Samar Esmail M, Abou-Zeid MA, Hafez Y. Changeability in stripe rust infection and grain yield of wheat associated with climatic factors. Environ Biodivers Soil Secur. 2019;2(0):143–53. doi:10.21608/jenvbs.2019.6674.1040. [Google Scholar] [CrossRef]
70. Arab SA, Shal MHEl, Eissa ST, Abou-Zeid MA. Assessment of some wheat genotypes for resistance to some diseases and their effect on yield and yield components. Plant Cell Biotechnol Mol Biol. 2021;22(13&14):73–83. [Google Scholar]
71. Salimi M, Razavi KC, Amiri MN, Esmaeili M, Khorramdel S, Moghani H, et al. Stability of agronomic traits of barley evolutionary populations under drought conditions in Iran. Agronomy. 2023;13(7):1931. doi:10.3390/agronomy13071931. [Google Scholar] [CrossRef]
72. Abou-Zeid MA, Abd Elhameed AS, Abd El-Wahab MMH. Evaluation of new wheat genotypes with genetics for stem rust resistance diversity and some yield components under Egyptian field conditions. Egypt J Plant Breed. 2018;22(4):849–71. [Google Scholar]
73. Atia MAM, El-Khateeb EA, Abd El-Maksoud RM, Abou-Zeid MA, Salah A, Abdel-Hamid AME. Mining of leaf rust resistance genes content in Egyptian bread wheat collection. Plants. 2021;10(7):1378. doi:10.3390/plants10071378. [Google Scholar] [CrossRef]
74. Xiong FQ, Zhong RC, Han ZQ, Jiang J, He LQ, Zhuang WJ, et al.Start codon targeted polymorphism for evaluation of functional genetic variation and relationships in cultivated peanut (Arachis hypogaea L.) genotypes. Mol Biol Rep. 2011;38(5):3487–94. [Google Scholar]
75. Ganie SH, Ganie SA, Khan MS, Ahmad A, Khattak N, Yasin M. Assessment of genetic diversity and population structure of barley using SCoT markers. Plant Genet Resour. 2019;17(5):407–16. doi:10.1007/s12298-019-00796-5. [Google Scholar] [CrossRef]
76. Dwivedi SL, Pandey M, Varshney RK, Prasad MK, Prasad R, Rai DK. Application of molecular markers in plant breeding: evidence and opportunities. Adv Agron. 2020;163(4):215–68. doi:10.1016/bs.agron.2020.01.002. [Google Scholar] [CrossRef]
77. Varshney RK, Kumar S, Kumar A, Pandey M, Nagarajan V, et al. Breeding climate resilient crops: opportunities and challenges. Theor Appl Genet. 2021;134(6):1729–52. doi:10.1007/s00122-021-03779-9. [Google Scholar] [CrossRef]
78. Singh B, Kumar S, Pandey M, Varshney RK, Kumar A. Genetic enhancement of crop tolerance to abiotic stresses in the era of climate change: progress and prospects. Front Plant Sci. 2018;9:1860. doi:10.3389/fpls.2018.01860. [Google Scholar] [CrossRef]
Cite This Article
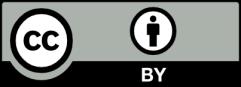
This work is licensed under a Creative Commons Attribution 4.0 International License , which permits unrestricted use, distribution, and reproduction in any medium, provided the original work is properly cited.