Open Access
REVIEW
Climate Change and Aquatic Phytoremediation of Contaminants: Exploring the Future of Contaminant Removal
Laboratory of Plant Stress Physiology, Department of Botany, Biological Sciences Sector, Federal University of Paraná, Paraná, 81531-980, Brazil
* Corresponding Author: Marcelo Pedrosa Gomes. Email:
Phyton-International Journal of Experimental Botany 2024, 93(9), 2127-2147. https://doi.org/10.32604/phyton.2024.056360
Received 21 July 2024; Accepted 11 September 2024; Issue published 30 September 2024
Abstract
Climate change, driven by anthropogenic activities, profoundly impacts ecosystems worldwide, particularly aquatic environments. This review explores the multifaceted effects of climate change on the phytoremediation capabilities of aquatic plants, focusing on the physiological responses to key environmental factors such as temperature, carbone dioxide (CO2) and ozone (O3) levels, pH, salinity, and light intensity. As global temperatures rise, moderate increases can enhance photosynthesis and biomass production, boosting the plants’ ability to absorb and detoxify contaminants, such as metals, pharmaceuticals, and nutrients. However, extreme temperatures and salinity levels impose stress, disrupting metabolic processes and reducing phytoremediation efficiency. Elevated CO2 levels generally stimulate growth and nutrient uptake, enhancing phytoremediation, but can also lead to nutrient imbalances and water acidification, complicating these benefits. Conversely, increased O3 levels cause oxidative stress, damaging plant tissues and undermining phytoremediation efforts. This review also highlights the critical role of light intensity and pH in regulating plant growth and contaminant uptake. Optimal light conditions and moderate pH changes can significantly enhance phytoremediation, while reduced light due to increased water turbidity and extreme pH fluctuations pose significant challenges. The interplay between these factors and the microbial communities associated with aquatic plants is explored, revealing complex interactions that influence overall remediation efficiency. By synthesizing current research, this review provides a comprehensive understanding of how climate change influences the physiological processes of aquatic plants and their phytoremediation capacity. The findings underscore the need for adaptive management strategies to harness the benefits of phytoremediation in mitigating water pollution under changing climatic conditions. This review calls for further research into the synergistic and antagonistic interactions between climate variables to develop resilient phytoremediation systems that effectively address environmental contaminants in a warming world.Keywords
Climate change, driven primarily by anthropogenic greenhouse gas emissions, reshapes ecosystems worldwide, significantly impacting plant physiology and phytoremediation capabilities. Predictions indicate that by the end of the 21st century, global surface temperatures could rise by 1.5°C to 5.7°C, with the most likely increase being around 2°C to 4°C under moderate emission scenarios [1]. Additionally, atmospheric CO2 concentrations are projected to increase from the current 400 to over 700 ppm, while light intensity and duration will fluctuate due to changes in cloud cover and solar radiation [1]. These shifts in environmental conditions have cascading effects on the physiological processes of aquatic plants, particularly those involved in the phytoremediation of contaminants such as metals, pharmaceuticals, and nutrients and their ability to remediate ecological contaminants.
Higher temperatures accelerate evaporation rates, leading to the salinization of water bodies [2,3], which imposes osmotic stress on aquatic plants. These plants must adjust their physiological mechanisms to cope with higher salt concentrations [4], diverting resources away from phytoremediation processes and potentially reducing their efficiency in contaminant uptake. Changes in light intensity and duration due to climate alterations can disrupt photosynthesis. Increased water turbidity from extreme weather events limits light penetration, reducing the growth and biomass of submerged plants [5] and directly affecting their capacity to absorb and process contaminants. Similarly, increased ozone (O3) levels are detrimental, causing oxidative stress and damaging cellular components, undermining the plants’ overall health [5] and phytoremediation abilities. In contrast, elevated atmospheric CO2 levels can stimulate plant growth by enhancing photosynthesis [5], resulting in better removal of water contaminants by plants.
Climate change also alters pH and water chemistry [6], affecting the solubility and bioavailability of nutrients [5] and pollutants. Plants must adapt their metabolic pathways to these shifts, which can hinder their efficiency in removing contaminants from the water. Furthermore, nutrient dynamics within aquatic ecosystems are changing. Higher temperatures accelerate organic matter decomposition, releasing nutrients like nitrogen and phosphorus more rapidly, while extreme weather events can lead to nutrient runoff [7]. These nutrient imbalances affect plant health and phytoremediation potential [7].
The frequency and severity of extreme climatic events, such as floods and droughts, are increasing [1], causing significant abiotic stress and physical damage to plants. This disruption can diminish their growth and capacity to mitigate pollutants. Moreover, climate change impacts the interaction between plants and their associated microbiomes. Temperature, humidity, and water chemistry shifts can destabilize these microbial communities [8], crucial in supporting plant health and enhancing phytoremediation processes. Phenological changes, such as alterations in the timing of flowering and growth cycles, are another consequence of climate change [9,10]. These shifts can desynchronize plant activities with environmental conditions, reducing their ability to absorb and detoxify pollutants optimally. Additionally, climate change may facilitate the invasion of non-native species [7], which can outcompete local aquatic plants for resources, thereby reducing the effectiveness of native plants in phytoremediation and altering the overall dynamics of the ecosystem. Furthermore, the environmental changes induced by climate change can affect the intrinsic physiological mechanisms [7] that enable plants to tolerate specific contaminants. These alterations could recalibrate plants’ tolerance and uptake capabilities, leading to beneficial or detrimental outcomes. Enhanced tolerance and uptake could improve phytoremediation effectiveness, whereas reduced tolerance might impair the plant’s ability to detoxify its environment.
The field of phytoremediation has seen substantial growth. A search on Google Scholar using the terms “Soil and Phytoremediation” or “Water and Phytoremediation” yielded over 25,000 publications since 2020, reflecting the substantial growth in this field. However, while phytoremediation in soil has been extensively studied, with the majority of these publications focused on soil, research related to water phytoremediation has been comparatively less represented [11,12], particularly in the context of the compounded effects of climate change on these processes. While individual studies have explored the impact of temperature, CO2 levels, or salinity on plant physiology, few have integrated these factors to examine their combined effects on phytoremediation efficiency under changing climatic conditions. This study addresses this gap by analyzing how climate change influences aquatic macrophytes’ physiological and biochemical responses. The novelty of this work lies in its comprehensive approach to understanding the interplay between various climate variables—such as temperature, CO2 and O3 levels, pH, salinity, and light intensity—and their collective impact on the efficiency of phytoremediation. By synthesizing current research across these domains, this study offers new insights into the complex interactions that can enhance or diminish aquatic plants’ phytoremediation capabilities under changing climatic conditions. Understanding these interactions is crucial for developing sustainable environmental management practices that can mitigate the negative impacts of climate change on water quality and ecosystem health. The findings underscore the urgent need for adaptive strategies to preserve and enhance phytoremediation efficiency as the climate changes. This study offers novel insights into the complex interactions between climate variables and plant functions by examining the cellular and biochemical mechanisms affected by climate change.
As the world faces the profound impacts of climate change, the need for innovative and sustainable solutions to address environmental challenges has become increasingly pressing. One such challenge is the growing threat of water pollution, exacerbated by the changing climate [13]. Eutrophication, the excessive enrichment of water bodies with nutrients, and other pollution pose significant risks to aquatic ecosystems and human health [14]. In this context, the role of aquatic phytoremediation, the use of aquatic plants to remove contaminants from water, has gained significant attention as a promising approach to address water quality issues [14]. Phytoremediation offers a sustainable and cost-effective alternative to conventional water treatment methods, making it an attractive option for communities and regions with limited resources. This method provides a high level of remediation without compromising the physical and chemical integrity of the environment. Moreover, it offers the potential for further extraction of contaminants from the plant biomass [15].
Aquatic macrophytes, or aquatic plants, have been the focus of significant research in phytoremediation, as they have demonstrated the ability to remove a wide range of contaminants from water bodies [15,16]. Species such as Eichhornia crassipes, Elodea canadensis, Azolla filiculoides, Lemna minor, L. gibba, Myriophyllum spicatum, Pistia stratiotes, and Salvinia molesta have shown promising results in this regard [15–21]. For instance, Buta et al. [22] demonstrated that E. crassipes, L. minor, and P. stratiotes have high phytoremediation potential for removing nitrogen species, phosphorus (P), iron (Fe), and chromium (Cr) from wastewater. After just one week of contact, these plants removed up to 99% of Cr, 97% of lithium (Li), 100% of ammoniacal nitrogen (NH3), 95% of P, and 96% of Fe. Similarly, E. canadensis removed up to 66% of Cr [23], 66.16% of arsenic (As) [24], 23% of enrofloxacin (Enro), and 30% of glyphosate [21] within only a few hours of exposure to contaminated water. S. molesta also demonstrates high efficacy in removing metals, particularly copper (Cu) and Cr (>50% removal), and to a lesser extent, zinc (Zn), lead (Pb), nickel (Ni), and cobalt (Co) [25]. Additionally, it can accumulate mercury (Hg), with bioconcentration factors reaching 856 [26], and reclaim more than 58% of ciprofloxacin (Cipro) from the medium after only 96 h of exposure [27].
As the effects of climate change continue to manifest, the importance of developing effective and sustainable solutions to address water pollution will only grow. Exploring aquatic phytoremediation as a tool for contaminant removal presents an opportunity to enhance water quality, promote ecosystem health, and foster resilient communities in the face of a changing climate. However, the successful implementation of phytoremediation is not without its challenges. The presence of multiple types of contaminants and climatic and hydrological conditions can limit the growth and effectiveness of the plants [16,28]. For instance, the presence of glyphosate in water can increase E. canadensis’ capacity to remove Enro by up to 700% due to the stimulation of the plant’s metabolism of the antibiotic. Conversely, the presence of Enro in water decreased the plant’s capacity to remediate glyphosate by up to 50% [21]. Factors such as temperature, pH, light, and salinity can all impact the ability of aquatic plants to remove pollutants effectively [29]. Therefore, it is essential to understand the effect of climate change on the remediation capacity of plants.
3 Temperature and Its Effects on Aquatic Plant Physiology and Phytoremediation
3.1 Effects of Temperature on Aquatic Plant Physiology
Temperature is a crucial factor affecting the physiological processes of aquatic plants. It influences respiration, photosynthesis, nitrogen metabolism, and oxidative metabolism. These processes are fundamental for plant growth, energy production, and stress responses, directly linking to the plant’s ability to tolerate and remediate environmental contaminants.
As temperatures rise, respiration rates generally increase to an optimum point, enhancing metabolic activity and energy production and facilitating greater uptake and detoxification of contaminants. For example, a temperature increase of 2.5°C is expected to boost respiration and primary production by 31% and 28%, respectively [30], and rising temperatures could even increase potential species richness in certain lake types [31]. However, temperatures exceeding the optimal range can lead to increased respiration that surpasses photosynthesis, resulting in a net loss of carbon and energy [32] (Table 1). Excessive temperatures can cause Rubisco deactivation and reduce chloroplast electron transport rates, co-limiting photosynthesis [33]. This imbalance impairs growth and reduces phytoremediation efficiency. Similarly, optimal temperatures enhance enzymatic activities involved in the Calvin cycle, leading to increased photosynthetic rates and biomass production (Table 1), critical for phytoremediation. However, temperatures above the optimum range can cause photoinhibition and damage to photosystem II, reducing the efficiency of light energy conversion and carbon fixation [34]. Elevated temperatures can also accelerate nitrogen uptake and assimilation [35,36], enhancing plants’ growth and detoxification abilities. However, extreme temperatures can disrupt nitrogen metabolism [35], accumulating toxic ammonium and reducing the synthesis of essential proteins and enzymes. Moreover, increased temperatures can indirectly affect plant nutrition by altering nutrient cycles. For instance, Kramer et al. [37] observed that temperatures from 29°C to 30°C promote cyanobacterial growth but suppress nitrogen fixation rates in lake communities. Temperature increases are also predicted to accelerate litter decomposition rates, potentially impacting carbon sequestration and affecting plant nutrition [38]. Temperature-induced oxidative stress is another significant challenge for aquatic plants. Elevated temperatures may increase the production of reactive oxygen species (ROS), causing oxidative damage to cellular components [39]. However, some plants counteract this by upregulating antioxidant enzymes, which may improve the plants’ tolerance and contaminant-removal capacity [40].
Aquatic plants have evolved various mechanisms to adapt to temperature fluctuations, such as changes in membrane fluidity, enzyme activity, and gene expression [41]. These adaptations enable some species to thrive in a broader range of temperature conditions, enhancing their effectiveness in phytoremediation under varying climatic conditions. For instance, an increase in temperature from 20°C to 30°C favored the harmful effects of the antibiotic Cipro on the mitochondrial activity of Ricciocarpus natans. However, it also enhanced the activity of antioxidant enzymes, preventing Cipro-induced oxidative stress and resulting in increased uptake of Cipro by the plants [42]. Similarly, in response to rising temperatures, aquatic plants modify pigment and membrane lipid composition, increasing membrane fluidity and, thus, cell permeability to water contaminants [43]. Understanding the complex interplay between temperature and plant physiology is crucial for designing and optimizing aquatic phytoremediation systems that can effectively remove contaminants in a changing climate.
3.2 Secondary Effects of Temperature Changes
Temperature changes have several secondary effects on the aquatic environment, which can further influence aquatic plants’ physiology and phytoremediation capabilities. For instance, increased temperatures accelerate evaporation rates, leading to higher water salinity. Elevated salinity levels impose osmotic stress on aquatic plants, disrupting ion balance and water uptake mechanisms [4] (Table 1). This osmotic stress can alter the plants’ physiological pathways, potentially enhancing their tolerance and uptake of specific contaminants, such as metals and pharmaceuticals (Fig. 1). For instance, heightened antioxidant enzyme activities under osmotic stress may improve the phytoremediation capacity of plants by mitigating oxidative damage from contaminants [55]. However, increased salinity can also result in decreased remediation ability by aquatic plants, as seen for metals (Cu, Zn, cadmium (Cd), and Pb) in E. canadensis and Potamogeton natans [50].
Figure 1: This figure illustrates the effects of moderate climate change (A) and extreme climate conditions (B) on submerged, floating, and emerging aquatic plants, highlighting the variations in their physiological responses and phytoremediation capacities. Moderate climate change (A): Mild increases in temperature and CO2 levels, along with improved light and pH conditions, enhance photosynthesis, growth, and remediation capabilities. Floating and emerging macrophytes exhibit more excellent positive responses compared to submerged species. Extreme conditions (B): Under extreme conditions characterized by rising temperatures, increased salinity, elevated O3 concentrations, pH fluctuations, and higher water turbidity, photosynthesis, growth, and remediation capabilities are reduced. Submerged species are particularly vulnerable to these stressors than floating and emerging macrophytes
Changes in precipitation patterns and water availability due to rising temperatures can also affect hydrological regimes, impacting the distribution and concentration of contaminants in aquatic environments [52,53]. Increases in rainfall and extreme weather events can lead to higher contaminant loads in waterways, and the interactive effects between rising contaminant concentrations and temperature in water are difficult to predict. For instance, increased temperature and nitrogen loading have significantly boosted cyanobacterial growth rates in lakes. However, this also impairs nitrogen fixation, contributing to nitrogen limitation in these ecosystems, which can profoundly affect plant communities, including the phytoremediation capacities of macrophytes. Nutrients such as nitrogen, phosphorus, and sulfur are crucial for a plant’s ability to cope with contaminants, modulating their phytoremediation capacity [56,57]. Fluctuating water levels can lead to drought and flooding, each presenting unique challenges and opportunities for phytoremediation. Drought conditions might concentrate pollutants, increasing the burden on plants, while flooding can disperse contaminants, potentially reducing their bioavailability but increasing the spatial extent of contamination. Moreover, increasing temperatures are closely linked to rising sea levels, contributing to the salinization of coastal and freshwater environments [58]. This salinization exacerbates osmotic stress on aquatic ecosystems, further influencing plant’s capacity for phytoremediation.
Climate-induced shifts in pest and disease patterns may also lead to increased use of pesticides and herbicides, contributing to higher levels of these contaminants in water bodies [59,60]. The emerging threat of insect pests driven by rising global temperatures is a clear example of this issue. A study on the sweet potato whitefly (Bemisia tabaci) in the southeastern United States found that warmer temperatures were associated with earlier and more abundant whitefly activity, particularly in areas with higher insecticide use [61]. The study suggests that frequent insecticide applications, driven by these climate-induced pest outbreaks, may disrupt biological control mechanisms, resulting in even more persistent pest problems. This creates a potential feedback loop, or “pesticide treadmill,” where increased temperatures and pest abundance lead to further pesticide use, exacerbating the contamination of water bodies as global climate change accelerates [62]. These chemicals can interact with the physiological responses of aquatic plants, affecting their growth and contaminant uptake capacities. For example, certain pesticides can inhibit or enhance specific metabolic pathways involved in phytoremediation, complicating predictions of plant performance under climate change scenarios. In E. canadensis, Roundup (a glyphosate-based herbicide) inhibits the activity of cytochrome P450, thereby decreasing the metabolism and uptake of the antibiotic Enro by plants. However, the presence of Enro in water increased glyphosate uptake and toxicity [21].
3.3 Case Studies and Comparative Analyses
Studies have explored the impacts of temperature on the phytoremediation capabilities of aquatic plants. Huynh et al. [44] investigated the removal of trace elements (Cd, As, Pb, Zn, and Cu) by water hyacinth (Eichornia crassipes) under different temperature regimes. Their findings showed that temperatures exceeding 33°C stifle plant development and decrease trace element-removal capacity. Similarly, Haris et al. [63] observed that temperature significantly affects the remediation capacity of water hyacinth, with optimal nutrient removal occurring at 30°C. Higher temperatures increased phosphorus release from organic matter, reducing the plant’s remediation efficiency. In contrast, increasing temperature from 11°C to 32°C enhanced the cyanide metabolism rate of weeping willows (Eleocharis acicularis), an emergent macrophyte, by 46% for every 10°C increase due to higher enzyme activity without causing increased cyanide accumulation or toxicity [64]. However, higher temperatures also improved the remediation capacity of E. acicularis for As-contaminated leachate. The absorption of As by E. acicularis was higher at an average temperature of 20.5°C (0.8% of total As absorbed) compared to 4.2°C (0.3% of total As absorbed) [65].
Fritioff et al. [50] observed increased metal (Cu, Zn, Cd, Pb) accumulation in E. canadensis and P. natans when the temperature was raised from 5°C to 20°C. Additionally, the Cu-remediation capacity of Typha latifolia improved with increasing temperatures from 18°C to 32°C [66]. A recent review by Pang et al. [67] reported that the metal-remediation capacity of macrophytes generally increases with temperature up to an optimal range of 20°C–30°C. Beyond 30°C, remediation capacity can decrease due to plant stress, with maximum removal observed around 25°C–30°C for the metals studied. Similarly, increased temperature (from 20°C to 30°C) favored ciprofloxacin (Cipro) uptake by Ricciocarpus natans [42]. However, increased temperature (from 12°C to 28°C) had little effect on the bioaccumulation of the herbicide isoproturon in freshwater macrophytes Egeria densa and Ludwigia natans. Still, it increased the herbicide burden in E. densa [68].
Temperature increases can also enhance selenium (Se) accumulation by giant reed (Arundo donax) by altering microbial activity, affecting selenium’s mobility, bioavailability, and volatility [69]. Indeed, temperature increases can modulate microbial activity in bioremediation, impacting the efficacy of natural biodegradation or the injection of biological materials [70]. For example, higher summer temperatures were associated with increased Pb and Co remediation capacity of Pistia stratiotes, likely due to lower dissolved oxygen levels and bacterial abundance, compared to lower winter remediation capacity [71]. Weirich et al. [72] observed increased nitrogen removal efficiency by P. stratiotes and E. crassipes during summer. Additionally, constructed wetlands planted with aquatic macrophytes showed increased denitrification rates as water temperatures rose from 12°C to around 26°C [73]. Temperature also influences the remediation capacity of both T. domingensis and Pontederia parviflora. Temperatures above 20°C, in the mesophilic range, allowed for optimal microbial and plant metabolism, leading to high removal efficiencies for chemical oxygen demand (COD) and suspended solids. Conversely, temperatures below 20°C, in the psychrophilic range, reduced the metabolism of microorganisms and aquatic plants, leading to lower remediation capacity. Large temperature fluctuations, with drops of over 10°C in a single day, negatively impacted the stability and performance of these systems [54].
Climate change can also influence the distribution and prevalence of aquatic plant species, affecting their availability and suitability for phytoremediation. Studies have shown that certain floating species, such as L. minor, exhibit greater tolerance to high temperatures than other morphotypes of macrophytes [29]. Increased temperatures and freshwater salinization favor the growth of floating macrophytes and phytoplankton [74] while decreasing the metal remediation capacity of submerged macrophytes like E. canadensis [50]. However, the metal (Mn and Fe) removal capacity of phytoplanktonic species such as Synechococcus elongatus (Cyanobacteria) and Chlorococcum infusionum (Chlorophyta) remains unaffected under these conditions [75]. These findings suggest that floating macrophytes, such as duckweed species, may be more suitable for phytoremediation under warmer and more saline conditions. Nevertheless, compared to submerged macrophytes, floating plants have proven to be less effective in removing some contaminants, such as the antibiotic erythromycin [76], which could result in lower overall effectiveness of phytoremediation programs. A potential solution is the use of mixed cultures of floating macrophytes. For example, more excellent growth rates were observed for both L. minor and S. molesta when grown together in water contaminated with the antibiotics Cipro and sulfamethoxazole (Sulfa), compared to systems with each plant growing separately. This co-culture approach resulted in more excellent removal of Cipro and Sulfa in the mixed system [77].
In summary, temperature impacts on aquatic phytoremediation are multifaceted and require a comprehensive understanding of plant physiology, environmental interactions, and system-level optimization (Fig. 1; Table 2).
4 Light Intensity and Its Effects on Aquatic Plant Physiology and Phytoremediation
4.1 Effects of Light Intensity on Aquatic Plant Physiology
Light is a fundamental factor affecting the physiological processes of aquatic plants. Changes in light conditions due to climate alterations can significantly impact these processes [7]. Light intensity directly influences photosynthetic rates and the growth patterns of aquatic plants (Table 1). Optimal light conditions enhance the efficiency of photosystem II and the Calvin cycle, leading to increased carbon fixation and biomass production [78], which are essential for phytoremediation. Conversely, low light conditions can lead to etiolation, reduced growth rates, and lower biomass [78], compromising phytoremediation efficiency. Moreover, reduced light penetration due to increased water turbidity from extreme weather events can limit photosynthesis [79], reducing the energy available for growth and contaminant uptake, especially in submerged plants [80]. Underwater darkening can also alter the ecological dynamics of ecosystems, particularly impacting plants with notable phytoremediation capacities. For example, a study conducted in East China examined the effects of light attenuation on the growth and photosynthetic traits of native phytoremediator plants like water thyme (Hydrilla verticillata) and Eurasian watermilfoil (Myriophyllum spicatum) compared to the invasive Carolina fanwort (Cabomba caroliniana). The study found that while light attenuation inhibits the growth of native submerged plants, it facilitates the growth of invasive species like C. caroliniana, which exhibited superior growth and photosynthetic traits under low underwater light conditions [80]. Using native species in phytoremediation programs is crucial to avoid introducing exotic species with potential ecological impacts. Changes in light conditions will affect the success of these programs, particularly in environments susceptible to underwater darkening.
On the other hand, excessive light intensity can also induce stress responses, such as the production of ROS [81,82], which can damage plant tissues and compromise their phytoremediation capabilities [55]. Light intensity can also affect the plant’s accumulation and distribution of contaminants by affecting their growth. Higher light levels may stimulate the production of chelating compounds, such as phytochelatins and metallothioneins, which can sequester and compartmentalize metals, enhancing their removal from the environment [83]. For instance, higher light intensities in the terrestrial metal hyperaccumulator plant Noccaea caerulescens increased biomass production and metal accumulation, improving phytoremediation efficiency [84]. However, caution is necessary, as hyperaccumulators may mobilize metals but only accumulate some, potentially increasing the risk of metal leaching [84].
Aquatic plants have developed various adaptive strategies to cope with fluctuations in light intensity. Some species, such as water hyacinth and duckweed, exhibit a high degree of phenotypic plasticity, allowing them to acclimate to a wide range of light conditions [83]. Other plants may allocate resources to specific physiological processes, such as increased production of light-harvesting pigments or antioxidant enzymes, to mitigate the effects of excessive or limited light [85].
4.2 Secondary Effects of Light Intensity Changes
Increased water turbidity, a consequence of climate-induced extreme weather events [3], reduces light penetration in aquatic environments. As discussed above, this reduced light availability affects photosynthesis and growth, decreasing phytoremediation efficiency. Turbidity also impacts the distribution of pollutants, potentially increasing their bioavailability in the water column [86] (Table 1). Climate change can also alter seasonal light patterns, affecting the timing and duration of light availability [87]. These changes can disrupt the phenology of aquatic plants, such as flowering and growth cycles, leading to mismatches between peak light availability and periods of active growth. Such disruptions can reduce phytoremediation efficiency, as plants may not be in their optimal growth phase when contaminants are most prevalent.
Understanding the relationship between light intensity, biomass production, and phytoremediation is crucial for developing effective strategies to enhance the removal of contaminants from aquatic environments. By optimizing light conditions and selecting plant species with high phenotypic plasticity or other adaptive traits, we can improve the efficiency of phytoremediation systems, making them more resilient to the impacts of climate change.
4.3 Case Studies and Comparative Analyses
Light significantly influences the growth and physiology of aquatic macrophytes, which in turn impacts their phytoremediation capabilities. Photosynthetic rates increase with light intensity up to a certain point, beyond which photoprotective mechanisms are activated to prevent damage [88]. Light-induced changes in the cellular redox environment play a crucial role in metabolic regulation, affecting various pathways, including carbon, nitrogen, and secondary metabolism [89], all of which are intrinsically involved in the phytoremediation capacity of macrophytes [55]. For instance, under low-light conditions, such as those caused by increased turbidity due to climate events, biomass production is reduced, and nutrient uptake in submerged species is adversely affected [90]. In submerged macrophytes like Potamogeton crispus, shaded conditions (42% and 11% of full sunlight) lead to reduced tissue soluble protein, soluble carbohydrates (SC) contents, and the SC/free amino acid (FAA) ratio, while increasing FAA concentrations, and promoting the activity of antioxidant enzymes, such as superoxide dismutase and guaiacol peroxidase. This results in aggravated carbon and nitrogen consumption and oxidative stress, disrupting the plant’s capacity for nutrient removal and contributing to the decline of submerged macrophytes in eutrophic lakes [91]. Conversely, intermediate light levels (around 30%–50% shade) are optimal for the phytoremediation potential of M. aquaticum, as this light regime promotes the greatest plant biomass and growth [92]. Light availability also affects the toxicity of herbicides to aquatic plants. For example, the herbicide atrazine decreased shoot length in E. canadensis grown under low-light conditions. Still, not biomass, while under optimal light conditions, atrazine significantly decreased both shoot length and biomass [93]. In Lemna sp. and Spirogyra sp., increases in light intensity have been shown to enhance the plant’s ability to remove pharmaceuticals and endocrine-disrupting chemicals from wastewater. The highest removal efficiencies were achieved in uncovered planted systems [94]. Similarly, increased light intensity enhanced the removal of diclofenac and Sulfa in wetlands. However, this enhancement was attributed to the photodegradation of the contaminants [95] rather than improved plant performance. Many organic pollutants, including pharmaceuticals, are particularly susceptible to photodegradation [96,97]. Therefore, even moderate increases in light intensity could potentially enhance remediation by facilitating the cleavage of these molecules. However, this process may also lead to the generation of intermediary metabolites, which could exhibit a higher level of toxicity to plants compared to their parent compounds [98], thereby compromising the plant’s performance in contaminant uptake.
Despite its importance, there are relatively few studies on the effect of light on the remediation capacity of macrophytes. It appears that changes in the light environment due to climate change predominantly affect submerged species of macrophytes due to increased water turbidity and decreased light availability. In contrast, floating and emerging plants seem tolerant to increased light intensity and regimes (Fig. 1). Understanding these light-dependent mechanisms is crucial for improving phytoremediation strategies and deserves more attention in future research.
5 CO2 and O3 Levels, and pH Fluctuations and Their Effects on Plant Physiology and Phytoremediation
The delicate balance of CO2, O3, and pH levels in aquatic ecosystems profoundly impacts the health and functioning of aquatic plants, which are vital for phytoremediation efforts (Table 1). Increased CO2 levels can enhance photosynthesis through the CO2 fertilization effect, leading to higher carbon fixation rates and growth [45]. Zhang et al. [99] demonstrated that dissolved inorganic carbon enhances primary production in submerged macrophytes, suggesting a co-limitation of carbon alongside traditional nutrients. The authors also found that CO2 fertilization accounted for over half of the biomass increase in northern extra-tropical forests. Similarly, Haverd et al. [100] highlighted a global CO2 fertilization effect, estimating a 30% increase in photosynthesis since 1900. This increased growth can improve the plants’ capacity to uptake and sequester contaminants, essential for phytoremediation efforts. However, while CO2 fertilization generally promotes growth, several factors can complicate these dynamics. For instance, vapor pressure deficit (VPD) can mitigate these benefits, as Li et al. [101] noted that VPD could offset up to 68.21% of the CO2 fertilization effect.
Several macrophytes have demonstrated phenotypic adaptations to varying partial pressures of CO2 (pCO2), indicating that they can maintain normal function in fluctuating CO2 environments [102]. Since most macrophytes can utilize either free CO2 or bicarbonate (HCO3−), they may exhibit stable responses to changes in pCO2 as long as one of these carbon forms is available. Consequently, they may be less vulnerable to climate-induced changes in pCO2 levels than other more sensitive taxonomic groups [102]. However, excessive CO2 can also lead to imbalances in nutrient uptake, potentially affecting the plants’ overall health and phytoremediation efficiency. For instance, while CO2 enrichment boosts photosynthesis and biomass production [45], it can also significantly reduce tissue concentrations of essential nutrients such as Fe and Mg [46]. These deficiencies impair the plants’ physiological functions, reducing their ability to tolerate and remediate contaminants effectively. Elevated CO2 levels can reduce iron solubility in water, leading to Fe deficiency, critical for chlorophyll production and photosynthesis. Similarly, CO2-induced Mg imbalances can impair chlorophyll synthesis and enzyme function, ultimately reducing the plant’s growth and phytoremediation capabilities [46].
Increasing CO2 levels also drive water acidification, disrupting plant physiology and remediation capacities since pH is a critical factor influencing nutrient availability, metal solubility, and overall plant health [103]. Ocean acidification is expected to drop 0.3–0.4 pH units in the surface ocean by 2100 if anthropogenic CO2 emissions continue at the current rate [104]. From 1982 to 2021, global surface ocean pH declined by −0.0166 ± 0.0010 per decade, with regional variations [105]. Even if atmospheric CO2 levels were reduced, the recovery of ocean acidification would take decades to centuries [106]. Freshwater ecosystems are similarly impacted. For instance, if increasing atmospheric pCO2 is the only forcing factor, pH in the Laurentian Great Lakes will decline at the same rate and magnitude as the surface ocean through 2100 [104]. Additionally, lakes in the northeastern USA are experiencing pH decreases, potentially increasing aluminum toxicity for fish [107].
As acidification progresses, plants must invest additional energy to maintain their acid-base balance and metabolic processes, affecting their growth, reproduction, and remediation capacities [45]. At optimal pH levels (typically around neutral), nutrient uptake is maximized, supporting healthy development and phytoremediation. However, extreme pH levels (either acidic or alkaline) can reduce nutrient availability and increase the solubility of toxic metals [108], posing challenges to plant health and contaminant uptake. pH changes can also influence the speciation and interaction of multiple contaminants [108,109], affecting their bioavailability and plant uptake [108]. Additionally, pH alterations can impact the composition and activity of the rhizosphere microbiome, which plays a vital role in nutrient cycling and contaminant degradation [51]. Optimal pH supports a diverse and active microbial community, enhancing phytoremediation, while extreme pH conditions can disrupt microbial activity and reduce contaminant degradation.
Elevated O3 levels cause oxidative stress in plants, producing ROS that damage cellular components [110,111]. This oxidative stress can impair photosynthesis, respiration, and overall plant growth. While plants upregulate antioxidant enzymes to counteract oxidative stress, chronic O3 exposure can overwhelm these defenses, reducing the plant’s capacity to tolerate and remediate contaminants. Additionally, O3 can significantly alter the composition and activity of the plant-associated microbiome, which is crucial in supporting plant health and enhancing phytoremediation [48]. CO2 and O3 levels can influence the plant-associated microbiome, with elevated CO2 potentially stimulating microbial activity, thereby enhancing nutrient and contaminant uptake [48]. However, high O3 levels can disrupt beneficial microbial communities, reducing phytoremediation efficiency. Elevated O3 levels have been shown to reduce phyllospheric bacterial diversity [112] and alter fungal community composition in plants [113]. Moreover, O3 exposure exacerbates plant disease severity by altering microbial co-occurrence networks [114]. Understanding the complex interplay between CO2, O3, and pH levels and their effects on aquatic plant physiology is crucial for optimizing phytoremediation strategies. By managing these environmental factors, we can enhance the resilience and effectiveness of phytoremediation systems in mitigating water pollution.
5.1 Case Studies and Comparative Analyses
Elevated CO2 levels have been shown to increase the phytoremediation efficiency of Noccaea caerulescens by enhancing biomass production and metal accumulation and reducing oxidative damage [115]. Similarly, CO2 enrichment stimulates the accumulation of sediment-derived minerals like Al, Fe, P, and N in the submerged macrophyte Vallisneria americana, with the effects being more pronounced at a lower pH of 5 compared to a higher pH of 7.3 [116]. This suggests that CO2 levels, in combination with pH, can significantly influence the nutrient uptake and overall health of aquatic plants.
In soil environments, elevated CO2 generally enhances rhizoremediation and phytoextraction of metals by increasing biomass and microbial activity in the rhizosphere, while elevated O3 has the opposite effect [48]. High O3 levels decrease the inputs of assimilates to the rhizosphere, negatively impacting decomposition processes, rhizoremediation, and metal phytoextraction efficiency. Elevated O3 also adversely affects microbial biomass, reducing the effectiveness of phytoremediation [48]. Increased CO2 significantly enhances the abundance of bacterial and functional genes related to CO2 assimilation and carbon decomposition, promoting photoautotrophy, hydrocarbon degradation, and cellulolysis [47]. However, elevated CO2 levels reduce the abundance of chemoautotrophic bacteria, including nitrifying bacteria. Culturing E. crassipes under elevated CO2 conditions decreases photosynthetic bacteria. Still, it increases bacteria involved in complex carbon decomposition due to root exudates. These interactions can decrease bacterial diversity and the abundance of CO2-assimilating, nitrifying, and certain carbon-degrading bacteria with denitrifying properties. Consequently, the interactions between aquatic plants and the bacterial community in eutrophic waters under elevated CO2 can benefit the environment and help mitigate the greenhouse effect [47]. Conversely, rising concentrations of CO2 and O3 have been shown to decrease the removal of polycyclic aromatic hydrocarbon (PAH) pollutants in grassland soils. This reduction in PAH degradation is linked to shifts in soil microbial community structure, specifically a reduction in gram-positive bacteria essential for soil enzyme production and PAH degradation [49]. These findings highlight the complex interplay between CO2, O3, and pH levels and their effects on phytoremediation. While elevated CO2 can enhance biomass production and microbial activity, thus improving phytoremediation efficiency, elevated O3 can negate these benefits by reducing microbial biomass and nutrient inputs to the rhizosphere. Additionally, changes in pH due to increased CO2 levels can further complicate these interactions, affecting nutrient availability and metal solubility.
Aquatic macrophytes respond differently to environmental factors such as CO2, O3, and pH. Floating and emerging macrophytes are most resilient to elevated CO2, showing notable enhancements in photosynthesis and biomass production [117]. This is attributed to their superior carbonic anhydrase activity, which is related to their higher carbonic anhydrase activity and bolsters their phytoremediation capacity in scenarios of increased CO2. In contrast, water acidification may adversely affect submerged macrophytes [117]. These plants are highly sensitive to pH fluctuations; optimal pH levels facilitate healthy growth and nutrient uptake, while extreme pH levels can decrease nutrient availability and enhance the solubility of toxic metals, thereby compromising phytoremediation efficiency. Emergent macrophytes can tolerate a broader range of pH levels than submerged types [118]. However, extreme pH levels can still affect nutrient availability and microbial activity, potentially reducing their effectiveness. Floating macrophytes are generally less sensitive to pH fluctuations than submerged types [117]. They may tolerate a broader range of pH levels, maintaining phytoremediation capabilities even under varying conditions. However, extreme pH levels can still impact nutrient uptake and microbial interactions. Regarding O3, submerged macrophytes are generally less affected by elevated O3, as atmospheric O3 does not readily dissolve in water, meaning their phytoremediation capacity is not significantly impacted. On the other hand, emergent macrophytes are more exposed to atmospheric O3, which can cause oxidative stress, reduce photosynthesis, and impair growth. Chronic exposure to high O3 levels can decrease phytoremediation efficiency by damaging plant tissues and reducing assimilated inputs to the rhizosphere. Floating macrophytes are directly exposed to atmospheric O3, which can cause oxidative damage and impair growth. While they exhibit high phenotypic plasticity and can adapt to varying O3 levels, chronic exposure may still reduce their effectiveness in phytoremediation (Fig. 1).
Despite the growing body of research on terrestrial systems, there is a notable lack of information regarding the impacts of CO2, O3, and pH fluctuations on aquatic phytoremediation. However, possible scenarios can be inferred. For instance, pharmaceuticals can be cleaved by ozonation and pH changes [119,120]. Thus, increased atmospheric CO2 (leading to water acidification) and higher O3 levels could enhance the degradation of organic contaminants, potentially aiding in their removal from water bodies. This suggests that elevated CO2 and O3 may affect plant health and growth; they could also contribute to the breakdown of certain pollutants, offering a mixed but potentially beneficial impact on phytoremediation in aquatic environments.
6 Prediction of the Overall Effect of Climate Changes
The overall effect of climate change on the phytoremediation capacity of aquatic plants is complex and multifaceted. Based on current research, several predictions can be made. First, under moderate climate change, aquatic plants are likely to enhance their growth and uptake of contaminants. Mild temperature and CO2 levels increase are expected to improve photosynthesis and development, boosting the plant’s ability to absorb and sequester contaminants. Additionally, optimal light conditions and moderate pH changes can further enhance these capabilities, making phytoremediation more efficient under such conditions. However, the situation changes drastically under extreme climate conditions. Extreme temperatures, high salinity, elevated O3 levels, and severe pH fluctuations can induce significant stress in aquatic plants. This stress reduces growth and, consequently, the capacity for phytoremediation. Moreover, the increased frequency and intensity of extreme weather events, such as droughts and floods, can disrupt water availability and oxygen levels, compromising the effectiveness of phytoremediation.
The interactions between various climate factors also play a crucial role. There is a delicate balance between synergistic effects, such as CO2 fertilization and moderate temperature increases, and antagonistic effects, such as oxidative stress from elevated O3 levels and extreme temperatures. This balance will ultimately determine the net impact on phytoremediation. Plants with robust adaptation and resilience strategies, including enhanced antioxidant defenses and metabolic adjustments, will be better equipped to mitigate the negative consequences of climate change and take advantage of any positive effects. Overall, the future of phytoremediation in the context of climate change will depend on the interplay of these various factors and the ability of aquatic plants to adapt to the changing environment.
7 Conclusion and Future Perspectives
The analysis presented in this review highlights the significant impacts of climate change on the phytoremediation capabilities of aquatic plants. The analysis presented in this review highlights the significant effects of climate change on the phytoremediation capabilities of aquatic plants. With fewer studies exploring phytoremediation in water compared to soil, it is evident that the aquatic dimension of this technology remains underexplored, particularly in the context of the complex interactions between climate variables. The multifaceted impacts of increased CO2 and O3 levels, temperature fluctuations, and pH changes pose significant challenges and opportunities for utilizing aquatic macrophytes in environmental remediation. Moderate climate changes, such as slight temperature and CO2 level increases, can enhance photosynthesis, growth, and contaminant uptake, thereby improving phytoremediation efficiency. However, extreme conditions, including high temperatures, elevated salinity, and severe pH fluctuations, can induce stress in aquatic plants, reducing their growth and remediation capacities. Moreover, the interactions between these various climate factors can synergize or antagonize the plants’ phytoremediation abilities. The balance of these effects will depend on the plants’ inherent adaptive and resilience mechanisms, such as antioxidant defenses and metabolic adjustments.
Given the complexity of these interactions, future research should focus on understanding the detailed physiological and biochemical responses of different macrophyte morphotypes under varying climate scenarios. This knowledge is crucial for developing adaptive management strategies to optimize phytoremediation efforts in the face of ongoing climatic shifts. Additionally, investigating the long-term effects of climate-induced changes on the microbial communities associated with aquatic plants, which play a crucial role in supporting phytoremediation, is essential for enhancing the resilience of these systems. Continued research and innovation are necessary to ensure the effectiveness of phytoremediation practices in a rapidly changing world. Developing resilient phytoremediation systems that can adapt to and thrive under extreme climate conditions will be vital for enhancing water quality, promoting ecosystem health, and ensuring the sustainability of these practices. By addressing these challenges, we can better harness the potential of aquatic macrophytes to mitigate the environmental impacts of climate change and contribute to preserving global water resources.
The findings of this review have practical implications for managing water bodies and developing sustainable environmental remediation practices. By identifying the specific conditions under which phytoremediation is most effective, this study provides a foundation for designing adaptive management strategies that can be implemented to mitigate the impacts of climate change on water quality and ecosystem health. Future research should continue to build on these findings, focusing on translating scientific insights into practical applications that can enhance the resilience and effectiveness of phytoremediation systems in a warming world.
Acknowledgement: None.
Funding Statement: Author expresses his gratitude for the contribution from the Conselho Nacional de Desenvolvimento Científico e Tecnológico (CNPq, Finance Code 302226/2022-2) for the research productivity fellowship awarded to Marcelo Pedrosa Gomes.
Availability of Data and Materials: Data sharing does not apply to this article as no datasets were generated or analyzed during the current study.
Ethics Approval: Not applicable.
Conflicts of Interest: The author declares that there are no conflicts of interest to report regarding the present study.
References
1. IPCC. Climate change 2021: the physical science basis. Contribution of working group I to the sixth assessment report of the intergovernmental panel on climate change. Cambridge, UK and New York, NY, USA: Cambridge University Press; 2021. doi:10.1017/9781009157896. [Google Scholar] [CrossRef]
2. Hassani A, Azapagic A, Shokri N. Global predictions of primary soil salinization under changing climate in the 21st century. Nat Commun. 2021;12:6663. doi:10.1038/s41467-021-26907-3. [Google Scholar] [PubMed] [CrossRef]
3. Tripathy KP, Mukherjee S, Mishra AK, Mann ME, Williams AP. Climate change will accelerate the high-end risk of compound drought and heatwave events. Proc Natl Acad Sci. 2023;120. doi:10.1073/pnas.2219825120. [Google Scholar] [PubMed] [CrossRef]
4. Balasubramaniam T, Shen G, Esmaeili N, Zhang H. Plants’ response mechanisms to salinity stress. Plants. 2023;12:2253. doi:10.3390/plants12122253. [Google Scholar] [PubMed] [CrossRef]
5. Seth P, Sebastian J. Plants and global warming: challenges and strategies for a warming world. Plant Cell Rep. 2024;43:27. doi:10.1007/s00299-023-03083-w. [Google Scholar] [PubMed] [CrossRef]
6. Dixit A, Madhav S, Mishra R, Srivastav AL, Garg P. Impact of climate change on water resources, challenges and mitigation strategies to achieve sustainable development goals. Arab J Geosci. 2022;15:1296. doi:10.1007/s12517-022-10590-9. [Google Scholar] [CrossRef]
7. Becklin KM, Anderson JT, Gerhart LM, Wadgymar SM, Wessinger CA, Ward JK. Examining plant physiological responses to climate change through an evolutionary lens. Plant Physiol. 2016;2016:00793. doi:10.1104/pp.16.00793. [Google Scholar] [PubMed] [CrossRef]
8. Singh N, Das PP. Climate changing impact on microbes and their interactions with plants: an overview. In: Parray JA, editor. Climate change and microbiome dynamics. Climate change management. Springer, Cham; 2023. p. 177–90. doi:10.1007/978-3-031-21079-2_12. [Google Scholar] [CrossRef]
9. Hassan T, Gulzar R, Hamid M, Ahmad R, Waza SA, Khuroo AA. Plant phenology shifts under climate warming: a systematic review of recent scientific literature. Environ Monit Assess. 2024;196:36. doi:10.1007/s10661-023-12190-w. [Google Scholar] [PubMed] [CrossRef]
10. Dixit S, Sivalingam PN, Baskaran RKM, Senthil-Kumar M, Ghosh PK. Plant responses to concurrent abiotic and biotic stress: unravelling physiological and morphological mechanisms. Plant Physiol Reports. 2024;29:6–17. doi:10.1007/s40502-023-00766-0. [Google Scholar] [CrossRef]
11. Truu J, Truu M, Espenberg M, Nõlvak H, Juhanson J. Phytoremediation and plant-assisted bioremediation in soil and treatment wetlands: a review. Open Biotechnol J. 2015;9:85–92. doi:10.2174/1874070701509010085. [Google Scholar] [CrossRef]
12. Zhakypbek Y, Kossalbayev BD, Belkozhayev AM, Murat T, Tursbekov S, Abdalimov E, et al. Reducing heavy metal contamination in soil and water using phytoremediation. Plants. 2024;13:1534. doi:10.3390/plants13111534. [Google Scholar] [PubMed] [CrossRef]
13. du Plessis A. Primary water quality challenges, contaminants and the world’s dirtiest places. In: Water as an inescapable risk. Springer, Cham; 2019. vol. 79, p. 79–114. doi:10.1007/978-3-030-03186-2_5. [Google Scholar] [CrossRef]
14. Cantonati M, Poikane S, Pringle CM, Stevens LE, Turak E, Heino J, et al. Characteristics, main impacts, and stewardship of natural and artificial freshwater environments: consequences for biodiversity conservation. Water. 2020;12:260. doi:10.3390/w12010260. [Google Scholar] [CrossRef]
15. Kochi LY, Freitas PL, Maranho LT, Juneau P, Gomes MP. Aquatic macrophytes in constructed wetlands: a fight against water pollution. Sustainability. 2020;12:1–21. doi:10.3390/su12219202. [Google Scholar] [CrossRef]
16. Marques RZ, Oliveira PGD, Barbato ML, Kitamura RSA, Maranho LT, Brito JCM, et al. Green solutions for antibiotic pollution: assessing the phytoremediation potential of aquatic macrophytes in wastewater treatment plants. Environ Pollut. 2024;357:124376. doi:10.1016/j.envpol.2024.124376. [Google Scholar] [PubMed] [CrossRef]
17. Anawar HM, Sanchez AG, Alam MTK, Rahman MM. Phytofiltration of water polluted with arsenic and heavy metals. Int J Environ Pollut. 2008;33:292. doi:10.1504/IJEP.2008.019400. [Google Scholar] [CrossRef]
18. Marcolino MS, Ziegelmann PK, Souza-Silva MVR, Nascimento IJB, Oliveira LM, Monteiro LS, et al. Clinical characteristics and outcomes of patients hospitalized with COVID-19 in Brazil: results from the Brazilian COVID-19 registry. Int J Infect Dis. 2021;107:300–10. doi:10.1016/j.ijid.2021.01.019. [Google Scholar] [PubMed] [CrossRef]
19. Poklonov VA, Ostroumov SA, Anikina EV, Sadchikov AP, Glebov VV, Erofeeva VV. Elodea canadensis: introduction, phytoremediation and spread control. Vestn North-Eastern Fed Univ. 2024;21:24–32. doi:10.25587/2222-5404-2024-21-1-24-32. [Google Scholar] [CrossRef]
20. González-Tavares C, Salazar-Hernández M, Talavera-López A, Salgado-Román JM, Hernández-Soto R, Hernández JA. Removal of Ni(II) and Cu(II) in Aqueous solutions using treated water Hyacinth (Eichhornia crassipes) as Bioadsorbent. Separations. 2023;10:289. doi:10.3390/separations10050289. [Google Scholar] [CrossRef]
21. Gomes MP, Tavares DS, Richardi VS, Marques RZ, Wistuba N, Moreira de Brito JC, et al. Enrofloxacin and Roundup® interactive effects on the aquatic macrophyte Elodea canadensis physiology. Environ Pollut. 2019;249:453–62. doi:10.1016/j.envpol.2019.03.026. [Google Scholar] [PubMed] [CrossRef]
22. Buta E, Borşan IL, Omotă M, Trif EB, Bunea CI, Mocan A, et al. Comparative remediation potential of Eichhornia crassipes, Lemna minor, and Pistia stratiotes in two treatment facilities in Cluj County, Romania. Horticulturae. 2023;9:503. doi:10.3390/horticulturae9040503. [Google Scholar] [CrossRef]
23. Sankaranarayanan V, Velusamy S, Sankaran P, Kandasamy K. Phytoremediation potential of Elodea canadensis for reduction of chromium–optimization using response surface methodology. Desalin Water Treat. 2023;293:192–9. doi:10.5004/dwt.2023.29514. [Google Scholar] [CrossRef]
24. Picco P, Hasuoka P, Verni E, Savio M, Pacheco P. Arsenic species uptake and translocation in Elodea canadensis. Int J Phytoremediation. 2019;21:693–8. doi:10.1080/15226514.2018.1556588. [Google Scholar] [PubMed] [CrossRef]
25. Sharma K, Saxena P, Kumari A. Bioaccumulation of multi-metals and associated oxidative stress in Salvinia molesta D. S. Mitchell. Int J Plant Environ. 2023;9:63–8. doi:10.18811/ijpen.v9i01.10. [Google Scholar] [CrossRef]
26. Sitarska M, Traczewska T, Filarowska W, Hołtra A, Zamorska-Wojdyła D, Hanus-Lorenz B. Phytoremediation of mercury from water by monocultures and mixed cultures pleustophytes. J Water Process Eng. 2023;52:103529. doi:10.1016/j.jwpe.2023.103529. [Google Scholar] [CrossRef]
27. Kitamura RSA, Brito JCM, Silva de Assis HC, Gomes MP. Physiological responses and phytoremediation capacity of floating and submerged aquatic macrophytes exposed to ciprofloxacin. Environ Sci Pollut Res. 2022;30:622–39. doi:10.1007/s11356-022-22253-z. [Google Scholar] [PubMed] [CrossRef]
28. Singh S, Singh A. Phytoremediation: a sustainable approach for restoration of metal contaminated sites. Int J Sci Res. 2016;5:2171–4. doi:10.21275/v5i2.NOV161464. [Google Scholar] [CrossRef]
29. Rai PK. Heavy metal phytoremediation from aquatic ecosystems with special reference to macrophytes. Crit Rev Environ Sci Technol. 2009;39:697–753. doi:10.1080/10643380801910058. [Google Scholar] [CrossRef]
30. Segatto PL, Battin TJ, Bertuzzo E. A network-scale modeling framework for stream metabolism, ecosystem efficiency, and their response to climate change. Water Resour Res. 2023;59:1217. doi:10.1029/2022WR034062. [Google Scholar] [CrossRef]
31. Lewerentz A, Hoffmann M, Hovestadt T, Raeder U, Sarmento Cabral J. Synergistic effects between global warming and water quality change on modelled macrophyte species richness. Oikos. 2023;2023(10):e09803. doi:10.1111/oik.09803. [Google Scholar] [CrossRef]
32. Crous KY, Uddling J, De Kauwe MG. Temperature responses of photosynthesis and respiration in evergreen trees from boreal to tropical latitudes. New Phytol. 2022;234:353–74. doi:10.1111/nph.17951. [Google Scholar] [PubMed] [CrossRef]
33. Scafaro AP, Posch BC, Evans JR, Farquhar GD, Atkin OK. Rubisco deactivation and chloroplast electron transport rates co-limit photosynthesis above optimal leaf temperature in terrestrial plants. Nat Commun. 2023;14:2820. doi:10.1038/s41467-023-38496-4. [Google Scholar] [PubMed] [CrossRef]
34. Öncel I, Keleş Y, Üstün AS. Interactive effects of temperature and heavy metal stress on the growth and some biochemical compounds in wheat seedlings. Environ Pollut. 2000;107:315–20. doi:10.1016/S0269-7491(99)00177-3. [Google Scholar] [PubMed] [CrossRef]
35. Hasanuzzaman M, Nahar K, Alam M, Roychowdhury R, Fujita M. Physiological, biochemical, and molecular mechanisms of heat stress tolerance in plants. Int J Mol Sci. 2013;14:9643–84. doi:10.3390/ijms14059643. [Google Scholar] [PubMed] [CrossRef]
36. Allen CR, Burr MD, Camper AK, Moss JJ, Stein OR. Seasonality, C:N ratio and plant species influence on denitrification and plant nitrogen uptake in treatment wetlands. Ecol Eng. 2023;191:106946. doi:10.1016/j.ecoleng.2023.106946. [Google Scholar] [CrossRef]
37. Kramer BJ, Gobler CJ. Simulated heat waves promote the growth but suppress the N2 fixation rates of Dolichospermum spp. and cyanobacterial communities in temperate lakes. Ecol Indic. 2023;147:109983. doi:10.1016/j.ecolind.2023.109983. [Google Scholar] [CrossRef]
38. Ouyang X, Kristensen E, Zimmer M, Thornber C, Yang Z, Lee SY. Response of macrophyte litter decomposition in global blue carbon ecosystems to climate change. Glob Chang Biol. 2023;29:3806–20. doi:10.1111/gcb.16693. [Google Scholar] [PubMed] [CrossRef]
39. Hendrix S, Dard A, Meyer AJ, Reichheld J-P. Redox-mediated responses to high temperature in plants. J Exp Bot. 2023;74:2489–507. doi:10.1093/jxb/erad053. [Google Scholar] [PubMed] [CrossRef]
40. Zhanassova K, Kurmanbayeva A, Gadilgereyeva B, Yermukhambetova R, Iksat N, Amanbayeva U, et al. ROS status and antioxidant enzyme activities in response to combined temperature and drought stresses in barley. Acta Physiol Plant. 2021;43:114. doi:10.1007/s11738-021-03281-7. [Google Scholar] [CrossRef]
41. Liu Z, Zhou T, Gao D. Genetic and epigenetic regulation of growth, reproduction, disease resistance and stress responses in aquaculture. Front Genet. 2022;13:191. doi:10.3389/fgene.2022.994471. [Google Scholar] [PubMed] [CrossRef]
42. Gomes MP, de Brito JCM, Bicalho EM, Silva JG, de Fátima Gomides M, Garcia QS, et al. Ciprofloxacin vs. temperature: antibiotic toxicity in the free-floating liverwort Ricciocarpus natans from a climate change perspective. Chemosphere. 2018;202:410–9. doi:10.1016/j.chemosphere.2018.03.048. [Google Scholar] [PubMed] [CrossRef]
43. Rawat N, Singla-Pareek SL, Pareek A. Membrane dynamics during individual and combined abiotic stresses in plants and tools to study the same. Physiol Plant. 2021;171:653–76. doi:10.1111/ppl.13217. [Google Scholar] [PubMed] [CrossRef]
44. Huynh AT, Chen Y-C, Tran BNT. A small-scale study on removal of heavy metals from contaminated water using water hyacinth. Processes. 2021;9:1802. doi:10.3390/pr9101802. [Google Scholar] [CrossRef]
45. Falkenberg LJ, Bellerby RGJ, Connell SD, Fleming LE, Maycock B, Russell BD, et al. Ocean acidification and human health. Int J Environ Res Public Health. 2020;17:4563. doi:10.3390/ijerph17124563. [Google Scholar] [PubMed] [CrossRef]
46. Li F, Guo D, Gao X, Zhao X. Water deficit modulates the CO2 fertilization effect on plant gas exchange and leaf-level water use efficiency: a meta-analysis. Front Plant Sci. 2021;12:1642. doi:10.3389/fpls.2021.775477. [Google Scholar] [PubMed] [CrossRef]
47. Shi M, Li J, Zhou Q, Wang G, Zhang W, Zhang Z, et al. Interactions between elevated CO2 levels and floating aquatic plants on the alteration of bacterial function in carbon assimilation and decomposition in eutrophic waters. Water Res. 2020;171:115398. doi:10.1016/j.watres.2019.115398. [Google Scholar] [PubMed] [CrossRef]
48. Formánek P, Rejšek K, Vranová V. Effect of elevated CO2, O3, and UV radiation on soils. Sci World J. 2014;2014:1–8. doi:10.1155/2014/730149. [Google Scholar] [PubMed] [CrossRef]
49. Ai F, Eisenhauer N, Jousset A, Butenschoen O, Ji R, Guo H. Elevated tropospheric CO2 and O3 concentrations impair organic pollutant removal from grassland soil. Sci Rep. 2018;8:5519. doi:10.1038/s41598-018-23522-z. [Google Scholar] [PubMed] [CrossRef]
50. Fritioff Å., Kautsky L, Greger M. Influence of temperature and salinity on heavy metal uptake by submersed plants. Environ Pollut. 2005;133:265–74. doi:10.1016/j.envpol.2004.05.036. [Google Scholar] [PubMed] [CrossRef]
51. Laurich JR, Lash E, O’Brien AM, Pogoutse O, Frederickson ME. Community interactions among microbes give rise to host-microbiome mutualisms in an aquatic plant. mBio. 2024;15:10. doi:10.1128/mbio.00972-24. [Google Scholar] [PubMed] [CrossRef]
52. Bolan S, Padhye LP, Jasemizad T, Govarthanan M, Karmegam N, Wijesekara H, et al. Impacts of climate change on the fate of contaminants through extreme weather events. Sci Total Environ. 2024;909:168388. doi:10.1016/j.scitotenv.2023.168388. [Google Scholar] [PubMed] [CrossRef]
53. Golladay SW, Battle J. Effects of flooding and drought on water quality in gulf coastal plain streams in Georgia. J Environ Qual. 2002;31:1266–72. doi:10.2134/jeq2002.1266. [Google Scholar] [PubMed] [CrossRef]
54. Souza DC, Iwakura L, de Lima SB, Locastro JK. Comparative effect of temperature on the performance of Typha domingensis pers. and Pontederia parviflora Alexander in phytotreatment. Acta Sci Technol. 2019;41:39038. doi:10.4025/actascitechnol.v41i1.39038. [Google Scholar] [CrossRef]
55. Gomes MP, Kitamura RSA, Marques RZ, Barbato ML, Zámocký M. The role of H2O2-scavenging enzymes (Ascorbate Peroxidase and Catalase) in the tolerance of Lemna minor to antibiotics: implications for phytoremediation. Antioxidants. 2022;11:151. doi:10.3390/antiox11010151. [Google Scholar] [PubMed] [CrossRef]
56. Ye JY, Tian WH, Jin CW. Nitrogen in plants: from nutrition to the modulation of abiotic stress adaptation. Stress Biol. 2022;2:4. doi:10.1007/s44154-021-00030-1. [Google Scholar] [PubMed] [CrossRef]
57. Cao Y, Ma C, Yu H, Tan Q, Dhankher OP, White JC, et al. The role of sulfur nutrition in plant response to metal(loid) stress: facilitating biofortification and phytoremediation. J Hazard Mater. 2023;443:130283. doi:10.1016/j.jhazmat.2022.130283. [Google Scholar] [PubMed] [CrossRef]
58. O’Connor D, Zheng X, Hou D, Shen Z, Li G, Miao G, et al. Phytoremediation: climate change resilience and sustainability assessment at a coastal brownfield redevelopment. Environ Int. 2019;130:104945. doi:10.1016/j.envint.2019.104945. [Google Scholar] [PubMed] [CrossRef]
59. Skendžić S, Zovko M, Živković IP, Lešić V, Lemić D. The impact of climate change on agricultural insect pests. Insects. 2021;12:440. doi:10.3390/insects12050440. [Google Scholar] [PubMed] [CrossRef]
60. Thomson B, Rose M. Environmental contaminants in foods and feeds in the light of climate change. Qual Assur Saf Crop Foods. 2011;3:2–11. doi:10.1111/j.1757-837X.2010.00086.x. [Google Scholar] [CrossRef]
61. Crossley MS, Smith OM, Barman AK, Croy JR, Schmidt JM, Toews MD, et al. Warmer temperatures trigger insecticide-associated pest outbreaks. Pest Manag Sci. 2024;80:1008–15. doi:10.1002/ps.7832. [Google Scholar] [PubMed] [CrossRef]
62. Cornish CM, Sweetman JN. A perspective on how glyphosate and 2,4-D in wetlands may impact climate change. Front Environ Sci. 2023;11:1682. doi:10.3389/fenvs.2023.1282821. [Google Scholar] [CrossRef]
63. Haris H, Fai CM, Binti Bahruddin AS, Dinesh AAA. Effect of temperature on nutrient removal efficiency of water hyacinth for phytoremediation treatment. Int J Eng Technol. 2018;7:81–4. doi:10.14419/ijet.v7i4.35.22330. [Google Scholar] [CrossRef]
64. Yu X-Z, Trapp S, Zhou P-H, Chen L. Effect of temperature on the uptake and metabolism of cyanide by weeping willows. Int J Phytoremediat. 2007;9:243–55. doi:10.1080/15226510701376141. [Google Scholar] [PubMed] [CrossRef]
65. Okazaki K, Kurahashi T, Yamazaki S, Sakakibara M. Temperature dependence for purification of leachate containing heavy metals by phytoremediation using the artificial channel. IOP Conf Ser: Earth Environ Sci. 2020;589:12019. doi:10.1088/1755-1315/589/1/012019. [Google Scholar] [CrossRef]
66. Adriano DC, Fulenwider A, Sharitz RR, Ciravolo TG, Hoyt GD. Growth and mineral nutrition of cattail (Typha) as influenced by thermal alteration. J Environ Qual. 1980;9:649–53. doi:10.2134/jeq1980.00472425000900040022x. [Google Scholar] [CrossRef]
67. Pang YL, Quek YY, Lim S, Shuit SH. Review on phytoremediation potential of floating aquatic plants for heavy metals: a promising approach. Sustainability. 2023;15:1290. doi:10.3390/su15021290. [Google Scholar] [CrossRef]
68. Grollier T, Feurtet-Mazel A, Boudou A, Ribeyre F. Role of temperature on isoproturon bioaccumulation and effects on two freshwater rooted macrophytes: Elodea densaand Ludwigia natans. Ecotoxicol Environ Saf. 1997;36:205–12. doi:10.1006/eesa.1996.1496. [Google Scholar] [PubMed] [CrossRef]
69. El-Ramady HR, Abdalla N, Alshaal T, Elhenawy AS, Shams MS, Faizy SE-DA, et al. Giant reed for selenium phytoremediation under changing climate. Environ Chem Lett. 2015;13:359–80. doi:10.1007/s10311-015-0523-5. [Google Scholar] [CrossRef]
70. EPA. Consideration of climate change at contaminated groundwater sites; 2021. Available from: https://www.epa.gov/system/files/documents/2024-01/consideration-of-climate-change-at-contaminated-groundwater-sites.pdf. [Accessed 2024]. [Google Scholar]
71. El-Liethy MA, Dakhil MA, El-Keblawy A, Abdelaal M, Halmy MWA, Elgarhy AH, et al. Temporal phytoremediation potential for heavy metals and bacterial abundance in drainage water. Sci Rep. 2022;12:8223. doi:10.1038/s41598-022-11951-w. [Google Scholar] [PubMed] [CrossRef]
72. Weirich CE, Feiden A, Souza CS, Marchetti CR, Aleixo V, Klosowski ÉS. Temperature influences swine wastewater treatment by aquatic plants. Sci Agric. 2021;78:41. doi:10.1590/1678-992x-2019-0325. [Google Scholar] [CrossRef]
73. Bachand PAM, Horne AJ. Denitrification in constructed free-water surface wetlands: II. Effects of vegetation and temperature. Ecol Eng. 1999;14:17–32. doi:10.1016/S0925-8574(99)00017-8. [Google Scholar] [CrossRef]
74. Moreira MH, They NH, Rodrigues LR, Alvarenga-Lucius L, Pita-Barbosa A. Salty freshwater macrophytes: the effects of salinization in freshwaters upon non-halophyte aquatic plants. Sci Total Environ. 2023;857:159608. doi:10.1016/j.scitotenv.2022.159608. [Google Scholar] [PubMed] [CrossRef]
75. Gomes MP, Kochi LY, Freitas PL, Figueredo CC, Juneau P. Periphytic Algae and Cyanobacteria from the rio doce basin respond differently to metals and salinity, showing different potential for bioremediation. Plants. 2021;10:2349. doi:10.3390/plants10112349. [Google Scholar] [PubMed] [CrossRef]
76. Rocha CS, Kochi LY, Ribeiro GB, Rocha DC, Carneiro DNM, Gomes MP. Evaluating aquatic macrophytes for removing erythromycin from contaminated water: floating or submerged? Int J Phytoremediat. 2022;24:1–9. doi:10.1080/15226514.2021.1991268. [Google Scholar] [PubMed] [CrossRef]
77. Kochi LY, Kitamura RSA, Rocha CS, Brito JCM, Juneau P, Gomes MP. Synergistic removal of Ciprofloxacin and Sulfamethoxazole by Lemna minor and Salvinia molesta in mixed culture: implications for Phytoremediation of antibiotic-contaminated water. Water. 2023;15:1899. doi:10.3390/w15101899. [Google Scholar] [CrossRef]
78. Leister D. Enhancing the light reactions of photosynthesis: strategies, controversies, and perspectives. Mol Plant. 2023;16:4–22. doi:10.1016/j.molp.2022.08.005. [Google Scholar] [PubMed] [CrossRef]
79. Gomes MP, Juneau P. Temperature and light modulation of herbicide toxicity on algal and cyanobacterial physiology. Front Environ Sci. 2017;5:50. doi:10.3389/fenvs.2017.00050. [Google Scholar] [CrossRef]
80. Huang X, Ke F, Lu J, Xie H, Zhao Y, Yin C, et al. Underwater light attenuation inhibits native submerged plants and facilitates the invasive co-occurring plant Cabomba caroliniana. Divers Distrib. 2023;29:543–55. doi:10.1111/ddi.13678. [Google Scholar] [CrossRef]
81. Mathur S, Agrawal D, Jajoo A. Photosynthesis: response to high temperature stress. J Photochem Photobiol B Biol. 2014;137:116–26. doi:10.1016/j.jphotobiol.2014.01.010. [Google Scholar] [PubMed] [CrossRef]
82. Shi Y, Ke X, Yang X, Liu Y, Hou X. Plants response to light stress. J Genet Genomics. 2022;49:735–47. doi:10.1016/j.jgg.2022.04.017. [Google Scholar] [PubMed] [CrossRef]
83. Lacoul P, Freedman B. Environmental influences on aquatic plants in freshwater ecosystems. Environ Rev. 2006;14:89–136. doi:10.1139/a06-001. [Google Scholar] [CrossRef]
84. Luo J, He W, Xing X, Wu J, Sophie GUXW. The variation of metal fractions and potential environmental risk in phytoremediating multiple metal polluted soils using Noccaea caerulescens assisted by LED lights. Chemosphere. 2019;227:462–9. doi:10.1016/j.chemosphere.2019.04.083. [Google Scholar] [PubMed] [CrossRef]
85. Ansari AA, Naeem M, Gill SS, Al Zuaibr FM. Phytoremediation of contaminated waters: an eco-friendly technology based on aquatic macrophytes application. Egypt J Aquat Res. 2020;46:371–6. doi:10.1016/j.ejar.2020.03.002. [Google Scholar] [CrossRef]
86. kumar Aravind J, Krithiga T, Sathish S, Renita AA, Prabu D, Lokesh S, et al. Persistent organic pollutants in water resources: fate, occurrence, characterization and risk analysis. Sci Total Environ. 2022;831:154808. doi:10.1016/j.scitotenv.2022.154808. [Google Scholar] [PubMed] [CrossRef]
87. Barnes PW, Robson TM, Zepp RG, Bornman JF, Jansen MAK, Ossola R, et al. Interactive effects of changes in UV radiation and climate on terrestrial ecosystems, biogeochemical cycles, and feedbacks to the climate system. Photochem Photobiol Sci. 2023;22:1049–91. doi:10.1007/s43630-023-00376-7. [Google Scholar] [PubMed] [CrossRef]
88. Wimalasekera R. Effect of light intensity on photosynthesis. In: Ahmad P, Ahanger MA, Alyemeni MN, Alam P, editors. Photosynthesis, productivity and environmental stress. New Jersey: Wiley; 2019. p. 65–73. doi: 10.1002/9781119501800.ch4. [Google Scholar] [CrossRef]
89. Borbély P, Gasperl A, Pálmai T, Ahres M, Asghar MA, Galiba G, et al. Light intensity-and spectrum-dependent redox regulation of plant metabolism. Antioxidants. 2022;11:1311. doi:10.3390/antiox11071311. [Google Scholar] [PubMed] [CrossRef]
90. Gao J, Hu W, Wang J, Cui Y, Li L. Response of growth-related traits of submerged macrophytes to light reduction: a meta-analysis. Sustainability. 2023;15:5918. doi:10.3390/su15075918. [Google Scholar] [CrossRef]
91. Zhang M, Cao T, Ni L, Xie P, Li Z. Carbon, nitrogen and antioxidant enzyme responses of Potamogeton crispus to both low light and high nutrient stresses. Environ Exp Bot. 2010;68:44–50. doi:10.1016/j.envexpbot.2009.09.003. [Google Scholar] [CrossRef]
92. Wersal RM, Madsen JD. Influences of light intensity variations on growth characteristics of Myriophyllum aquaticum. J Freshw Ecol. 2013;28:147–64. doi:10.1080/02705060.2012.722067. [Google Scholar] [CrossRef]
93. Brain RA, Hoberg J, Hosmer AJ, Wall SB. Influence of light intensity on the toxicity of atrazine to the submerged freshwater aquatic macrophyte Elodea canadensis. Ecotoxicol Environ Saf. 2012;79:55–61. doi:10.1016/j.ecoenv.2011.12.001. [Google Scholar] [PubMed] [CrossRef]
94. Garcia-Rodríguez A, Matamoros V, Fontàs C, Salvadó V. The influence of Lemna sp. and Spirogyra sp. on the removal of pharmaceuticals and endocrine disruptors in treated wastewaters. Int J Environ Sci Technol. 2015;12:2327–38. doi:10.1007/s13762-014-0632-x. [Google Scholar] [CrossRef]
95. Lee E, Shon HK, Cho J. Role of wetland organic matters as photosensitizer for degradation of micropollutants and metabolites. J Hazard Mater. 2014;276:1–9. doi:10.1016/j.jhazmat.2014.05.001. [Google Scholar] [PubMed] [CrossRef]
96. Gamelas SRD, Tomé JPC, Tomé AC, Lourenço LMO. Porphyrin-containing materials for photodegradation of organic pollutants in wastewaters: a review. Catal Sci Technol. 2024;14:2352–89. doi:10.1039/D4CY00092G. [Google Scholar] [CrossRef]
97. Bano K, Mittal SK, Singh PP, Kaushal S. Sunlight driven photocatalytic degradation of organic pollutants using a MnV2O6/BiVO4 heterojunction: mechanistic perception and degradation pathways. Nanoscale Adv. 2021;3:6446–58. doi:10.1039/D1NA00499A. [Google Scholar] [PubMed] [CrossRef]
98. Zacchini M. Bismuth interaction with plants: uptake and transport, toxic effects, tolerance mechanisms—a review. Chemosphere. 2024;360:142414. doi:10.1016/j.chemosphere.2024.142414. [Google Scholar] [PubMed] [CrossRef]
99. Zhang Y, Liu Z, Wu Y, Ma S, Cao W, Lai C, et al. Relationships between biomass of phytoplankton and submerged macrophytes and physicochemical variables of water in Lake Caohai, China: implication for mitigation of cyanobacteria blooms by CO2 fertilization. J Hydrol. 2023;617:129111. doi:10.1016/j.jhydrol.2023.129111. [Google Scholar] [CrossRef]
100. Haverd V, Smith B, Canadell JG, Cuntz M, Mikaloff-Fletcher S, Farquhar G, et al. Higher than expected CO2 fertilization inferred from leaf to global observations. Glob Chang Biol. 2020;26:2390–402. doi:10.1111/gcb.14950. [Google Scholar] [PubMed] [CrossRef]
101. Li S, Wang G, Zhu C, Lu J, Ullah W, Fiifi Tawia Hagan D, et al. Vegetation growth due to CO2 fertilization is threatened by increasing vapor pressure deficit. J Hydrol. 2023;619:129292. doi:10.1016/j.jhydrol.2023.129292. [Google Scholar] [CrossRef]
102. Hasler CT, Butman D, Jeffrey JD, Suski CD. Freshwater biota and rising CO2? Ecol Lett. 2016;19:98–108. doi:10.1111/ele.12549. [Google Scholar] [PubMed] [CrossRef]
103. Anthony KRN, Kline DI, Diaz-Pulido G, Dove S, Hoegh-Guldberg O. Ocean acidification causes bleaching and productivity loss in coral reef builders. Proc Natl Acad Sci. 2008;105:17442–6. doi:10.1073/pnas.0804478105. [Google Scholar] [PubMed] [CrossRef]
104. Phillips J, McKinley G, Bennington V, Bootsma H, Pilcher D, Sterner R, et al. The potential for CO2-induced acidification in freshwater: a great lakes case study. Oceanography. 2015;25:136–45. doi:10.5670/oceanog.2015.37. [Google Scholar] [CrossRef]
105. Ma D, Gregor L, Gruber N. Four decades of trends and drivers of global surface ocean acidification. Global Biogeochem Cycles. 2023;37:673. doi:10.1029/2023GB007765. [Google Scholar] [CrossRef]
106. Jiang J, Cao L, Jin X, Yu Z, Zhang H, Fu J, et al. Response of ocean acidification to atmospheric carbon dioxide removal. J Environ Sci. 2024;140:79–90. doi:10.1016/j.jes.2023.04.029. [Google Scholar] [PubMed] [CrossRef]
107. Johannesson KH, Horne JD, Misra A, Aliperta C, Meletis OV, Santore RC, et al. Acidification of Northeastern USA lakes from rising anthropogenic-sourced atmospheric carbon dioxide and its effects on aluminum speciation. Geophys Res Lett. 2023;50:33. doi:10.1029/2023GL104957. [Google Scholar] [CrossRef]
108. Shi Y, Li Y. Impacts of ocean acidification on physiology and ecology of marine invertebrates: a comprehensive review. Aquat Ecol. 2024;58:207–26. doi:10.1007/s10452-023-10058-2. [Google Scholar] [CrossRef]
109. Marques RZ, da Silva Nogueira K, de Oliveira Tomaz AP, Juneau P, Wang S, Gomes MP. Emerging threat: antimicrobial resistance proliferation during epidemics—a case study of the SARS-CoV-2 pandemic in South Brazil. J Hazard Mater. 2024;470:134202. doi:10.1016/j.jhazmat.2024.134202. [Google Scholar] [PubMed] [CrossRef]
110. Singh AA, Ghosh A, Agrawal M, Agrawal SB. Secondary metabolites responses of plants exposed to ozone: an update. Environ Sci Pollut Res. 2023;30:88281–312. doi:10.1007/s11356-023-28634-2. [Google Scholar] [PubMed] [CrossRef]
111. Nowroz F, Hasanuzzaman M, Siddika A, Parvin K, Caparros PG, Nahar K, et al. Elevated tropospheric ozone and crop production: potential negative effects and plant defense mechanisms. Front Plant Sci. 2024;14:886. doi:10.3389/fpls.2023.1244515. [Google Scholar] [PubMed] [CrossRef]
112. Li P, Wu X, Gao F. Ozone pollution, water deficit stress and time drive poplar phyllospheric bacterial community structure. Ecotoxicol Environ Saf. 2023;262:115148. doi:10.1016/j.ecoenv.2023.115148. [Google Scholar] [PubMed] [CrossRef]
113. Chung H, Zak DR, Lilleskov EA. Fungal community composition and metabolism under elevated CO2 and O3. Oecologia. 2006;147:143–54. doi:10.1007/s00442-005-0249-3. [Google Scholar] [PubMed] [CrossRef]
114. Bhandari R, Sanz-Saez A, Leisner CP, Potnis N. Xanthomonas infection and ozone stress distinctly influence the microbial community structure and interactions in the pepper phyllosphere. ISME Commun. 2023;3:1875. doi:10.1038/s43705-023-00232-w. [Google Scholar] [PubMed] [CrossRef]
115. Luo J, Yang G, Igalavithana AD, He W, Gao B, Tsang DCW, et al. Effects of elevated CO2 on the phytoremediation efficiency of Noccaea caerulescens. Environ Pollut. 2019;255:113169. doi:10.1016/j.envpol.2019.113169. [Google Scholar] [PubMed] [CrossRef]
116. Titus J, Andorfer J. Effects of CO2 enrichment on mineral accumulation and nitrogen relations in a submersed macrophyte. Freshw Biol. 1996;36:661–71. doi:10.1046/j.1365-2427.1996.00135.x. [Google Scholar] [CrossRef]
117. Frost-Christensen H, Sand-Jensen K. Comparative kinetics of photosynthesis in floating and submerged Potamogeton leaves. Aquat Bot. 1995;51:121–34. doi:10.1016/0304-3770(95)00455-9. [Google Scholar] [CrossRef]
118. Weaver CI, Wetzel RG. Carbonic anhydrase levels and internal lacunar CO2 concentrations in aquatic macrophytes. Aquat Bot. 1980;8:173–86. doi:10.1016/0304-3770(80)90049-2. [Google Scholar] [CrossRef]
119. Rozas O, Baeza C, Núñez K, Rossner A, Urrutia R, Mansilla HD. Organic micropollutants (OMPs) oxidation by ozone: effect of activated carbon on toxicity abatement. Sci Total Environ. 2017;590-591:430–9. doi:10.1016/j.scitotenv.2016.12.120. [Google Scholar] [PubMed] [CrossRef]
120. Gorito AM, Pesqueira JFJR, Moreira NFF, Ribeiro AR, Pereira MFR, Nunes OC, et al. Ozone-based water treatment (O3, O3/UV, O3/H2O2) for removal of organic micropollutants, bacteria inactivation and regrowth prevention. J Environ Chem Eng. 2021;9:105315. doi:10.1016/j.jece.2021.105315. [Google Scholar] [CrossRef]
Cite This Article
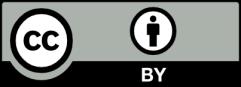
This work is licensed under a Creative Commons Attribution 4.0 International License , which permits unrestricted use, distribution, and reproduction in any medium, provided the original work is properly cited.