Open Access
ARTICLE
L-glutamic Acid and L-aspartic Acid Supplementation Mitigate Heavy Metal-Induced Stress in Phaseolus vulgaris L.
Department of Agricultural Biotechnology, Faculty of Agriculture, Ataturk University, Erzurum, 25240, Turkey
* Corresponding Author: Esra Arslan Yuksel. Email:
(This article belongs to the Special Issue: Abiotic and Biotic Stress Tolerance in Crop)
Phyton-International Journal of Experimental Botany 2024, 93(9), 2189-2207. https://doi.org/10.32604/phyton.2024.055053
Received 14 July 2024; Accepted 06 August 2024; Issue published 30 September 2024
Abstract
Heavy metal contamination in the environment, resulting from human activities or natural processes, poses a significant and widespread challenge. L-glutamic (L-glu) and L-aspartic acid (L-asp) treatments have been reported to improve plant metabolism of heavy metal-exposed plants, but the role of these amino acids in the resistance to lead (Pb2+), cadmium (Cd2+), arsenic (As3+) and nickel (Ni2+) treated-bean are unclear when applied together. This study investigated the L-glu and L-asp supplementation-induced changes in some physio-biochemical parameters and some stress-related gene expression levels in Pb2+, Cd2+, As3+, and Ni2+-stressed Phaseolus vulgaris (Elkoca and Gina) grown in nutrient solution. The combination of two L-glu and L-asp (1.5 and 3 mM) and Cd2+ (1 mM), Pb2+ (1 mM), As3+ (2 mg/L−1), and Ni2+ (1 mM) concentrations were used. The physio-biochemical parameters of the leaves were determined based on the chlorophyll content (SPAD), relative water content (RWC), hydrogen peroxide (H2O2), malondialdehyde (MDA), and electrolyte conductivity (EC) contents, and superoxide dismutase (SOD), peroxidase (POD) and ascorbate peroxidase (APX) enzyme activities. Additionally, the metal tolerance protein (MTP) genes, known for their role in heavy metal detoxification, and as PvSOD, PvPOD, and PvAPX antioxidant enzyme genes were analyzed for their temporal expression patterns in leaves. Preliminary results indicate a nuanced response of PvMTPs genes, highlighting potential variations in genotype-specific expression. Among the analyzed PvMTP7, PvMTP8, PvMTP9, PvMTP10, PvMTP11, and PvMTP13 genes, PvMTP10 exhibited particularly higher expression values in all treatments. In heavy metal-exposed beans, there was a concurrent decrease in SPAD, RWC, H2O2, and MDA contents, coupled with an increase in EC values. In turn, L-glu and L-asp pretreatments decreased the harmful effects of heavy metals by increasing the SPAD and RWC values and decreasing the EC, H2O2, and MDA contents and they reached these values close to control. Also, they improved the expressions of the above-mentioned genes. The 3 mM L-glu and L-asp appear to be more effective than 1.5 mM L-glu and L-asp in alleviation of the heavy metal’s toxic effects on two genotypes of bean. The findings contribute to our understanding of some stress-related gene expression levels and physio-biochemical responses under heavy metal stresses and offer insights into the potential application of L-glu and L-asp in enhancing stress tolerance in plants.Keywords
Heavy metals represent a significant abiotic factor contributing to environmental pollution, as these metals are non-degradable and remain in the ecosystem for extended periods [1]. Soil contamination with heavy metals leads to losses in agricultural yield and serious damage by entering the food chain. While a sufficient amount of essential heavy metals takes part in normal plant growth and development, excessive accumulation of both essential (zinc, manganese, iron, cobalt, copper, etc.), and non-essential heavy metals (mercury, silver, cadmium, lead, etc.), cause toxic symptoms [2]. Lead (Pb2+), cadmium (Cd2+), arsenic (As3+), and nickel (Ni2+) are widely recognized as highly toxic heavy metals to plants, with their deleterious effects surpassing those of other heavy metals. Numerous studies have emphasized the remarkable toxic effects of Pb2+, Cd2+, As3+, and Ni2+ due to their ability to disrupt essential metabolic processes within plant cells [3–5]. The toxicity of these metals arises from their similarities with essential nutrients, leading to their uptake by plants through transport systems designed for essential elements [6].
The damaging mechanisms of these metals contain (a) binding metals to sulphydryl groups in proteins and enzymes, leading to inhibition of activity or deconstruct of structure [7], and (b) stimulate the production of reactive oxygen species (ROS) that initiate lipid peroxidation and disrupt the cell membrane structure [8,9]. This oxidative damage compromises the membrane’s selective permeability, resulting in the leakage of cellular components and disruption of essential cellular functions. Additionally, heavy metals interfere with chlorophyll biosynthesis and function, leading to a reduction in chlorophyll content. The disruption of chlorophyll pigments negatively impacts photosynthetic efficiency and overall plant metabolism [10,11]. The combined effects on cell membrane stability and chlorophyll content contribute to the overall decline in plant health and growth in metal-contaminated environments. Studies have shown that heavy metals induce an increase in hydrogen peroxide (H2O2) levels in plant tissues, representing a key marker of oxidative stress [10]. Additionally, heavy metals can stimulate lipid peroxidation, leading to the generation of malondialdehyde (MDA) [12]. The accumulation of H2O2 and MDA reflects the imbalance between ROS production and the antioxidant defense system in response to heavy metal-induced stress. Plant cells cope with excessive ROS accumulation by activating the antioxidant defense system. Antioxidant enzymes [superoxide dismutase (SOD), catalase (CAT), peroxidase (POD), ascorbate peroxidase (APX), glutathione peroxidase (GPX), glutathione reductase (GR)] participate in mitigating the harmful effects of ROS. Plants use different antioxidant mechanisms in specific organs to combat oxidative stress, depending on the type of stress and their stage of development [13].
Plants have developed specialized mechanisms for the uptake and transport of heavy metals [14]. To avoid cellular damage, heavy metals are typically bound to low molecular weight compounds and either transported to organelles or expelled into the extracellular space by specific transporters. The metal transporter genes involved in these functions are called metal ion transport proteins in plants. Cation Diffusion Facilitator (CDF) family, also known as Metal Tolerance Proteins (MTPs), is one of the metal transporter protein families that play a role in the transport of divalent metals such as Co2+, Pb2+, Ni2+, Mn2+, Cd2+, Fe2+ and Zn2+ in plants [15]. CDF transporters, demonstrating a broad phylogenetic distribution, are encountered in nearly all realms of life and can be categorized into three sub-groups: Zn-CDF, Fe/Zn-CDF, and Mn-CDF [16]. MTPs catalyze the flow of these metal ions from the cytoplasm into the subcellular compartments or out of the cell [17]. MTP transporters have an important role in increasing resistance to heavy metal stress and homeostasis is found in different species, including plants, fungi, bacteria, and mammals. Also, they provide information in studies of biological enrichment and bioremediation strategies [16].
MTP proteins have been identified in many plant species, including Arabidopsis thaliana [18], Populus trichocarpa [19], Triticum aestivum [20] Nicotiana tabacum [21], etc. Numerous Zn-CDF proteins have been the subject of investigation since the discovery of the first member, AtMTP1, in Arabidopsis. Zn-CDF genes are pivotal in augmenting plant tolerance to zinc. Notably, AtMTP1 and AtMTP3, located in the tonoplast, contribute to Zn and cobalt (Co) tolerance by facilitating the excessive transport of Zn2+ and Co2+ ions into the vacuole. Additionally, members of the Mn-CDF family, such as AtMTP8, are integral components in the intricate process of manganese (Mn) transportation [22]. However, information on MTP genes other than Arabidopsis is still limited [23]. So, there is a gap in information about the expression levels of MTP genes in Pb2+, Cd2+, As3+, and Ni2+ treated-Phaseolus vulgaris seedlings. Furthermore, the role of amino acid bio-stimulants in the activation of MTPs has not been studied in beans.
P. vulgaris is an important legume consumed worldwide in human nutrition because of rich source of protein, carbohydrates, vitamins, and minerals. P. vulgaris L. is classified as a plant sensitive to heavy metal stress and also is a hyper-accumulator of heavy metals in the root system, which can cause various types of damage to common bean growth [24,25].
Utilizing amino acid biostimulants represents an innovative approach in contemporary agriculture aimed at alleviating toxicity induced by abiotic stresses, such as heavy metal stress [26]. L-glutamic acid (L-glu) and L-aspartic acid (L-asp) are among the amino acids incorporated into the formulation of biostimulant products. L-glu plays a pivotal role in nitrogen metabolism, facilitating nitrogen assimilation in plants and participating in aminotransferase reactions. Beyond its direct function as an amino acid, it serves as a precursor to some amino acids [27]. L-asp is synthesized in plants through a transamination reaction involving glutamate and oxaloacetate. Subsequently, it undergoes metabolic processes leading to the production of many amino acids following a sequence of reactions known as the L-asp metabolic pathway. Applying these two amino acids to plants aids in their resilience against unfavorable environmental conditions like drought, salinity, and heavy metal toxicity [28]. Yet, to date, the impact of individually applying these amino acids on plant physiology and primary metabolism remains unclear, as does the potential for antagonistic or synergistic effects resulting from their mixture. For this, the objectives of the study were to investigate the effects of the application of the amino acids L-glu and L-asp as a mixture, on some physio-biochemical parameters and the expression levels of six putative PvMTPs (PvMTP7, PvMTP8, PvMTP9, PvMTP10, PvMTP11, and PvMTP13) and PvSOD, PvPOD, and PvAPX genes under Pb2+, Cd2+, As3+, and Ni2+ stress-treated Elkoca and Gina genotypes of P. vulgaris.
2.1 Plant Material, Experimental Design and Sample Collection
The seeds of P. vulgaris L. abiotic stress tolerant genotypes Elkoca (tolerant) and Gina (moderate tolerant) were cultivated at the greenhouse of Atatürk University research station. The relative humidity of the greenhouse was 40% ± 5% and the day and night temperatures were 25°C ± 2°C and 20°C ± 2°C, respectively. Seeds were first sown at a depth of 1–1.5 cm in viols filled with peat. Twelve days later, equally sized five seedlings were transferred to each 1.5 liter pots containing soil:sand:peat (2:1:1 v:v:v) (the soil is loamy with 31% sand, 36% silt and 33% clay) mixture. The experiment was conducted in five biological replicates for thirteen treatment groups for each genotypes that comprised, (1) 1 mM cadmium nitrate [Cd(NO3)2] [29] (2) Cd(NO3)2 + 1.5 mM L-glu + 1.5 mM L-asp, (3) Cd(NO3)2 + 3 mM L-glu + 3 mM L-asp, (4) 1 mM lead nitrate [Pb(NO3)2] [30] (5) Pb(NO3)2 + 1.5 mM L-glu + 1.5 mM L-asp, (6) Pb(NO3)2 + 3 mM L-glu + 3 mM L-asp, (7) 2 mg/L−1 sodium arsenite (NaAsO2) [31] (8) NaAsO2 + 1.5 mM L-glu + 1.5 mM L-asp, (9) NaAsO2 + 3 mM L-glu + 3 mM L-asp, (10) 1 mM nickel sulphate (NiSO4.6H2O) [32] (11) NiSO4.6H2O + 1.5 mM L-glu + 1.5 mM L-asp, (12) NiSO4.6H2O + 3 mM L-glu + 3 mM L-asp treatments and (13) non-treated (control). The pots were watered with irrigation water added with 1/4 hoagland nutrient solution [33] for 7 days. Once the first trifoliate leaf appeared, 200 mL of each Cd(SO4)2 ((481882, Sigma-Aldrich Chemie GmbH, Taufkırchen, Germany), Pb(NO3)2 (228621, Sigma-Aldrich Chemie GmbH, Taufkırchen, Germany), NaAsO2 (S7400, Sigma-Aldrich Chemie GmbH, Taufkirchen, Germany), NiSO4.6H2O (N4482, Sigma-Aldrich Chemie GmbH, Taufkirchen,Germany), L-glu (607851, Sigma-Aldrich Chemie GmbH, Taufkirchen, Germany) and L-asp (604852, Sigma-Aldrich Chemie GmbH, Taufkirchen, Germany) combination solutions were sprayed to the leaves every 2 days for 10 days. Also, irrigation water (for control) were sprayed to the leaves. After 10 days, the measurements were made on fresh leaves taken from the plants.
2.2 Chlorophyll Meter Measurements
The SPAD values were calculated by a SPAD 502 chlorophyll meter. The readings were measured along the widest midsection and each side of the leaflets from four plants per treatment. Four spots were marked and two readings were recorded on each spot.
2.3 Relative Water Content Measurements
Leaf discs (1 cm in diameter) were excised from fully expanded young bean leaves. The fresh weights (FW) were weighed, and then the discs were kept in distilled water for 4 h. Subsequently, turgid weights (TW) were determined. The discs were dried at 72°C to obtain dry weight (DW). The RWC was calculated according to the standard method given by [34].
2.4 Electrolyte Conductivity Measurements
Ten discs of fresh leaf (1 cm diameter) were taken from the fully expanded leaves (two plants each replication) and the samples were washed twice with distilled water, then, were soaked in closed tubes containing 20 mL of distilled water and incubated for 24 h. Afterwards, the initial electrical conductivity of the water was measured (EC1) using a conductometer (Mettler, Inlab-73X series). The samples were then kept in an autoclave at 121°C for 20 min for releasing all electrolytes, and second conductivity (EC2) was measured. The rate of electrolytes conductivity was calculated as follows [35]:
2.5 Hydrogen Peroxide and Malondialdehyde Content Measurements
The fresh leaf (0.5 g) was homogenized with 5 mL of trichloroacetic acid (TCA, 0.1%) and centrifuged at 12.000 × g for 15 min at 4°C. 0.5 mL of the supernatant was added with 0.5 mL of 10 mM potassium phosphate buffer (pH = 7.0) and 1 mL of 1 M potassium iodide. The absorbed value of the supernatant was measured using a nanodrop spectrophotometer (Thermo, Multiskan Go, Ratastie 2, Finland) at 390 nm and the H2O2 content was calculated as nmol/kg [36]. The MDA content was evaluated by the 2-thiobarbituric acid in a hot acidic medium method reported by [37] with some modifications. The samples were quantified at 532 and 600 nm, and calculated as nmol/kg.
2.6 Antioxidant Enzyme Activity Measurements
The superoxide dismutase (SOD; EC 1.15.1.1) activity was measured at 560 nm according to Giannopolitis et al. [38]. The peroxidase (POD; EC 1.11.1.7) activity was measured at 470 nm according to Shams et al. [39]. The ascorbate peroxidase (APX; EC 1.11.1.11) activity was measured at 290 nm according to Murshed et al. [40].
2.7 RNA Isolation, cDNA Synthesis and Gene Expression Analysis
Total RNA was isolated from the leaves of the bean genotypes with TRI reagent (T9424, Sigma-Aldrich Chemie GmbH, Taufkırchen, Germany) according to manufacturer’s protocol. Further, RNA isolation samples were treated with Dnase I (EN0521, Thermo Fisher Scientific, Vilnius, Lithuania) to remove DNA contamination. The RNA purity was checked by measuring the absorbance with a Nanodrop spectrophotometer (Thermo, Multiskan Go), and also its integrity and gDNA contamination was determined with 1.5% agarose gel. Then, cDNA was synthesized with 1 µg of total RNA using the Maxima first strand cDNA Synthesis Kit (K1651, Thermo Scientific™) following manual instructions. Tenfold dilution products were stored at −20°C until qPCR studies. The qPCR was carried out using a maxima sybr green/rox qPCR master mix (2X) (FE-K0221, Fermentas). The reaction was performed as follows: 94°C for 30 s, followed by 40 cycles of 94°C, 5 s, and 60°C, 34 s. All the samples were carried out in three biological replicates. The gene sequences examined were retrieved from the Phytozome v13 database (http://www.phytozome.net, accessed on 30 July 2024). Primers were designed using Primer 3 web v4.1.0 software (http://primer3.ut.ee/, accessed on 30 July 2024). The primer sequences used in this study were shown in details in Table 1.
The analyses were subjected to analysis of variance (ANOVA) using SPSS 20.0 software package (www.spss.com, accessed on 30 July 2024) (IBM, Armonk, NY, USA) and LSD (least significant difference) test at the significance level p < 0.01. Also, the data from qPCR amplification was analyzed using 2−ΔΔCT method. Heatmap analysis combined with hierarchical cluster analysis was performed using OriginPro 2024b (OriginLab Corporation Northampton, MA, USA).
3.1 Changes of Physio-Biochemical Parameters of Bean Seedlings under Different Treatments
In this study as expected, SPAD value significiantly (p < 0.05) decreased with the heavy metal treatments compared to control both Elkoca and Gina and the variation patterns were different (Fig. 1a,b). The decrease in SPAD value became much faster in As3+ treatments (17.1 and 14.2 in Elkoca and Gina, respectively). On contrary, the highest L-asp+L-glu treatment (A2) significantly alleviated the inhibitory effects of heavy metals on chlorophyll content that these values were 25.6 in Elkoca and 20.6 in Gina in Pb2++A2, 24.7 in Elkoca and 17.7 in Gina in Cd2++A2, 21.4 and 19.4 in Gina in As3++A2 and 25.6 in Elkoca and 13.6 in Gina in Ni2++A2 treatments. The maximum value of SPAD was calculated by 28.4 in Elkoca and 23.4 in Gina in A2 treatment, respectively (Fig. 1a,b). Simultaneously, RWC decreased significantly (p < 0.05) after different treatments. The highest RWC was detected in the plants of control (85.6% and 79.7% in Elkoca and Gina, respectively), while the lowest was detected after Cd2+ treatment (61.6% and 51.7% in Elkoca and Gina, respectively) (Fig. 1c,d). In turn, moderate and the highest L-asp+L-glu treatments significantly improved the RWC values under heavy metal conditions (Fig. 1c,d). Following the control conditions, the As3++A2 treatment resulted in a calculated maximum RWC value of 78.15% in Elkoca, while this value was detected as 68.3% under Ni2++A2 treatment in Gina. For the change of EC, it was showed that EC resulted in an increase (p < 0.05) in plants that after all treatments. The EC values were increased in Gina by 53.7%, 161%, 420.1% and 174.3%, while these values detected in Elkoca by 95.74%, 256.7%, 450.6% and 187.22% in Pb2+, Cd2+, As3+ and Ni2+ treatments, respectively, compared to control. While the highest L-asp+L-glu dose (A2) was more effective in reducing EC in both genotypes under As3+ stress (29.7% decrease in Elkoca and 41.94% in Gina), it was less effective under Pb2+ treatment (28.8% decrease in Elkoca and 7.87% in Gina) (Fig. 1e,f).
Figure 1: Effect of moderate (A1) and the highest (A2) L-asp+L-glu treatments on SPAD (a, b), RWC (c, d) and EC (e, f) values in Elkoca and Gina genotypes grown under Pb2+, Cd2+, As3+ and Ni2+ exposures. The vertical bars indicate the standard error of the average of treatment (n = 3). In each figure, the means with the same letter are not significantly different at the 0.01 level of probability according to the Duncan’s multiple range test
The MDA and H2O2 contents increased significiantly (p < 0.05) in all the treatments, compared to control. In both genotypes, the MDA content increased the most under As3+ treatment (136.9% in Gina and 156.3% in Elkoca), while the least increase was observed under Cd2+ treatment (66.9% in Gina and 54.3% in Elkoca) compared to control (Fig. 2a,b). The H2O2 results, however, showed some differences compared to the MDA results. The increase in H2O2 content differed between the two genotypes. In the Elkoca genotype, the highest increase was observed under Cd2+ treatment (102.5%), whereas in the Gina genotype, it was under As3+ treatment (102.6%) (Figs. 2c,d). Additionally, L-asp+L-glu treatments generally reduced H2O2 and MDA contents in a dose-dependent manner. An exception to this was that in the Gina genotype, the As3++A1 treatment was more effective in reducing MDA content compared to the As3++A2 treatment (Fig. 2b). The L-asp+L-glu treatments resulted in a decrease in MDA content, with the most significant reduction observed in the Elkoca genotype under Ni2++A2 treatment, while in the Gina genotype, it was occurred under As3++A1 treatment. Furthermore, L-asp+L-glu treatments conducted in combined with heavy metals were found to be more effective in reducing H2O2 content in the Gina genotype, with the greatest reduction observed in the As3++A2 treatment (32.6%) (Fig. 2d).
Figure 2: Effect of moderate (A1) and the highest (A2) L-asp+L-glu treatments on MDA (a, b) and H2O2 (c, d) values in Elkoca and Gina genotypes grown under Pb2+, Cd2+, As3+ and Ni2+ exposures. The vertical bars indicate the standard error of the average of treatment (n = 3). In each figure, the means with the same letter are not significantly different at the 0.01 level of probability according to the Duncan’s multiple range test
3.2 Changes of Antioxidant Enzyme Activities and Their Gene Expression Profiles of Bean Seedlings under Different Treatments
In the present study, it is also advocated response of SOD, POD and APX and also expression levels of PvSOD, PvPOD and PvAPX genes under Pb2+, Cd2+, As3+ and Ni2+ treatments with or without the increasing levels of L-asp+L-glu (Figs. 3 and 4). The activity of these enzymes and relative expression levels were enhanced in all treatments in both genotypes compared to control plants. These increase rates were higher in the Elkoca generally compared to the Gina. Among heavy metals, As3+ observed to most significantly (p < 0.05) increase SOD enzyme activity in both genotypes by 114.8% and 173.93%, respectively (Fig. 3a,b). Similarly, the expression of PvSOD increased the most with As3+ treatment in both Elkoca and Gina genotypes by 17 fold and 11 fold, respectively, compared to control (Fig. 4a). In Elkoca, following As3+, Cd2+, Ni2+ and Pb2+ sequentially increased both SOD enzyme activity and PvSOD gene expression. In turn, this pattern was variable in Gina. Besides, when the plants were treated with moderate (A1) and the highest (A2) L-asp+L-glu, both enzyme activities and their gene expression rates increased generally with increasing L-asp+L-glu doses. The highest increase in SOD activity was observed in the As3++A1 treatment in Elkoca (119.64%), while, it was observed in the As3++A2 treatment in Gina (176.52%). Concurrently, the highest increase in PvPOD expression was observed in both genotypes under the As3++A2 treatment (22.4 fold in Elkoca and 15.6 fold in Gina), while the lowest increases were found in the Pb2++A1 (8.1 fold) in Elkoca and Pb2++A2 (3.5 fold) in Gina.
Figure 3: Effect of moderate (A1) and the highest (A2) L-asp+L-glu treatments on SOD (a, b), POD (c, d) and APX (e, f) values in Elkoca and Gina genotypes grown under Pb2+, Cd2+, As3+ and Ni2+ exposures
Figure 4: Effect of moderate (A1) and the highest (A2) L-asp+L-glu treatments on PvSOD (a), PvPOD (b), PvAPX (c), PvMTP7 (d), PvMTP8 (e), PvMTP9 (f), PvMTP10 (g), PvMTP11 (h) and PvMTP13 (i) gene expression values in Elkoca and Gina genotypes grown under Pb2+, Cd2+, As3+ and Ni2+ exposures
A similar result was observed in POD enzyme activity for Elkoca, where As3+ treatment led to the highest increase (58.94%) then Pb2+, Cd2+ and Ni2+ in the order: As3+ > Pb2+ > Cd2+ > Ni2+ compared to control (Fig. 3c). Interestingly, PvPOD expression increased more with Ni2+ (12.4 fold), then As3+ (9.2 fold), Pb2+ (9.1 fold) and Cd2+ (7.4 fold) in Elkoca (Fig. 4b). In Gina, both POD enzyme activity (79.3%) and PvPOD expression showed the most significant (p < 0.05) increases with Ni2+ treatment (8.1 fold). Additionally, other heavy metals significantly increased both POD enzyme activity and PvPOD expression in the same order: As3+ > Pb2+ > Cd2+ (Figs. 3d, 4b). The elevated doses of L-asp+L-glu caused generally an increase both POD enzyme activity and PvPOD expression in two genotpes. A significant 90.5% (As3++A2 in Elkoca) and 118.7% (Ni2++A2 in Gina) increase in the POD enzyme activity was noted (Fig. 3c,d). On the other hand, Ni2++A2 treatment, resulted in the highest increase in PvPOD expression in both genotypes (14.1 fold in Elkoca and 11.3 in Gina) compared to control (Fig. 4b).
As it was shown in the Figs. 3e and 4c, APX enzyme activity and PvAPX expression rate dramatically increased most by Cd2+ treatments which were 157.2% and 9.1 fold respectively higher than that of control. This increase was followed sequentially for APX enzyme activity and PvAPX expression by Pb2+ (122.7% and 6.2 fold), As3+ (89.6% and 4.1 fold) and Ni2+ (79.7% and 3.4 fold) in Elkoca (Figs. 3e, 4c). Similarly, the highest increases in APX enzyme activity (252%) and PvAPX expression rate (12.3 fold) were observed with Cd2+ treatment in Gina, compared to control (Figs. 3f, 4c). In turn, the ranking of heavy metals following Cd2+ in terms of enhancing both APX enzyme activity and PvAPX gene expression differed from that observed in the Elkoca. This ranking was as followed: Cd2+ > As3+ > Ni2+ > Pb2+ for both parameters. Furthermore, the application of L-asp+L-glu doses caused significant increases in the value of APX enzyme activity and PvAPX expression rates. In Elkoca, the Cd2++A2 treatment resulted in the highest increase in both APX enzyme activity (175.6%) and PvAPX expression rate (11.4 fold) compared to control. In turn, this pattern varied in Gina that the highest APX enzyme activity observed under the Cd2++A2 treatment (273.6%), while the PvAPX expression showed the greatest increase under the Ni2++A2 treatment (11.5 fold) (Figs. 3e, 4c).
3.3 Changes of PvMTPs Gene Expression Profiles of Bean Seedlings under Different Treatments
The expression of PvMTPs in Elkoca and Gina genotypes were also analyzed. Among the PvMTP1-13 genes, primers PvMTP7, PvMTP8, PvMTP9, PvMTP10, PvMTP11 and PvMTP13, which gave the best results in qPCR, were used (Fig. 4d–i). The outcomes of the present research indicate a markedly different pattern in the expression of PvMTP genes in both genotypes in response to Pb2+, Cd2+, As3+ and Ni2+ under L-asp+L-glu treatments. This could be attributed to the spatial and temporal expression patterns of the PvMTPs genes involved. Some PvMTPs, including PvMTP7, PvMTP8 and PvMTP10 were highly expressed in all treatments in Elkoca, only PvMTP10 was highly expressed in all treatments in Gina (Fig. 4d,e,g). Notably, PvMTP10 exhibited particularly higher expression values from 4.5 to 25.1 fold-changes in both genotypes (Fig. 4g). Among Pb2+, Cd2+, As3+ and Ni2+ treatments, only Cd2+ increased the expression of all PvMTPs genes in all treatments for both genotypes. Besides, Ni2+ treatment exhibited relatively low or with moderately low expression levels in all PvMTP genes. Apart from these, it could be stated that the treatments of L-asp+L-glu generally increased PvMTPs expression levels in both genotypes under Pb2+, Cd2+, As3+ and Ni2+ treatments. This increase was particularly notable at the highest (A2) L-asp+L-glu treatments. This increase was most pronounced in the PvMTP10 gene (Fig. 4g). These findings collectively highlight the differential expression patterns of MTP genes in response to different treatments in both Elkoca and Gina genotypes, emphasizing the complex regulatory mechanisms involved.
3.4 Hierarchical Clustering Heatmap
The interaction between the many examined parameters of the bean-treated with heavy metals and L-asp+L-glu was evaluated using the hierarchical clustering heatmap (Fig. 5). Heatmap analysis of Elkoca and Gina genotypes based on Pb2+, Cd2+, As3+, Ni2+ and L-asp+L-glu treatments grouped the various parameters into two main clusters. SPAD and RWC of both genotypes showed in the same cluster. Other cluster consisted of all remaining parameters. On the other hand, heatmap analysis grouped the treatments into two main clusters. Control (C), moderate (A1) and the highest (A2) L-asp+L-glu treatments included in the same cluster, while remaining treatments in the other cluster. In a more detailed analysis, As3+ treatments formed a distinct cluster separate from other metal treatments, while Cd2+ and Pb2+ treatments were grouped within the same cluster, and Ni2+ treatments formed a completely separate cluster (Fig. 5).
Figure 5: Heatmap analysis combined with hierarchical cluster analysis of various attributes of Elkoca and Gina genotypes of P. vulgaris L. grown under Pb2+, Cd2+, As3+, Ni2+ and L-asp+L-glu treatments
Globally, the health, growth, and productivity of plants greatly reduced due to agricultural soils containing high levels of toxic metals [41]. This study’s findings showed that stress induced by Pb2+, Cd2+, As3+ and Ni2+ significantly damaged physio-biochemical parameters and altered the expression of the PvMTPs, PvSOD, PvPOD, and PvAPX genes in both P. vulgaris L. genotypes. In turn, L-asp+L-glu treatments improved these variables under stress conditions in various ways. In a previous study, it was determined that the combined application of L-asp and L-glu was more effective in influencing the physiological and metabolic processes of plants under stress conditions compared to their individual applications [42]. Building on this knowledge, this study also achieved more effective results with the combined application of these two amino acids. These improvements were more pronounced in the Elkoca genotype. Indeed, previous studies considered that bean genotype Gina is categorized as a moderate tolerant genotype to stress [43], while Elkoca is categorized as a tolerant genotype [44].
As observed in the study, SPAD value, is a portable device used to measure chlorophyll content and photosynthetic capacity, decreased with heavy metal treatments. This decrease was particularly notable in Gina. The toxicity of heavy metal stress on photosynthesis was documented in different kind of plant species [45–47]. Heavy metals can significantly affect photosystem I and II, electron transport chain, and chlorophyll biosynthesis in plants that inhibits the activity of chlorophyll synthase and block the chlorophyll synthesis [48]. The increase in SPAD value under L-asp+L-glu treatments might be linked to its capability to uphold the balance between chlorophyll synthesis and degradation under heavy metal stress. Also, it is known that glutamic acid is a precursor in the biosynthesis of chlorophyll. This was in line with the results of previous studies that glutamic acid [49], and aspartic acid [50] restored chlorophyll content after stresses. Amin et al. [51] applied aspartic acid and thiourea individually and in combination to faba beans, and found that the increase in chlorophyll content was higher with the combined treatment, indicating that combined applications were more effective than individual ones. Similar findings were detected by Alfosea-Simon et al. [42] that there is a synergistic effect between L-asp and L-glu on the increase in SPAD value and some other metabolic processes of plants.
Moreover, stress negatively affects RWC that associated with the disruption of cell membrane integrity and the impairment of root water uptake mechanisms. Considering the pronounced effect of heavy metals on the reduction of RWC, it can be suggested that this may be related, at least indirectly, alterations in stomatal conductance, transpiration rates, and water retention capacity [52]. Under L-asp+L-glu treatments the RWC values increased that the highest value was obtained in the A2 application (3 mM L-asp+L-glu) in both genotypes. This effect can be explained by the enhanced K+ content and decreased Na+ levels, as well as the regulation of ion transport that influences water relations. Some of the roles of amino acids have been gaining prominence in the literature as, for example, their action in the regulation of ion transport [53].
The present data indicate a similar tendency in the changes in EC, MDA and H2O2 values. EC indicates cell membrane permeability is widely used as a test for stress-induced damage in plants and as a measure of plant stress tolerance [54]. The use of EC as a reliable parameter for evaluating heavy metal toxicity in plants, emphasizing its significance in understanding the physiological responses to environmental stresses [11]. As shown in the study, individually heavy metal treatments specifically, As3+ caused a significant increase in EC values in both genotypes. EC is a rapid response to stress, occurring within minutes to hours, primarily driven by the efflux of K+ ions from plant cells. Under severe toxic heavy metal stress, the loss of K+ from plant cells, also known as K+ efflux or electrolytic leakage, is substantial. This process induces programmed cell death by enhancing the enzymatic activities of proteases and endonucleases [55]. After the treatment with L-asp+L-glu that in moderate tolerant variety decreased more, indicating that the L-asp+L-glu treatments had better effects on protecting the cell membrane. Compared to a study where aspartic acid alone reduced EC value by 20% under Cd stress [50], this study suggested that the synergistic effect of L-asp and L-glu was more effective (about 38%) in reducing EC value. These findings underscore the potential of exogenous amino acids to modulate membrane stability, as indicated by EC measurements, offering insights into the mechanisms underlying their protective effects in plants subjected to heavy metal stress.
Additionally, H2O2 and MDA levels increased with heavy metal treatments but decreased with the combined applications of L-asp+L-glu and heavy metals in both genotypes. Especially, in Gina genotype under As3+ stress, the substantial reduction in both H2O2 and MDA levels with L-asp+L-glu treatments indicated effective stress mitigation. Indeed, studies demonstrated that glutamic acid was the better treatment to enhance As3+ tolerance in rice through increasing antioxidants and osmolytes to scavenge excessive ROS [56,57]. They suggested that the role of L-glu in enhancing growth is attributed to its interaction with antioxidant metabolism, which promotes tolerance to As3+ stress. Therefore, it may be assumed that, in this case, the high SOD, POD and APX antioxidant enzyme activities in the heavy metal-exposed bean genotypes under the L-asp+L-glu treatments increased the tolerance of heavy metals. The mitigation of cadmium-induced oxidative stress by L-asp has been associated with its beneficial effects on the activities of SOD, POD, CAT, and APX in rice [49]. On the other hand, Teixeira et al. [58] showed that glutamate treatment did not increase the activity of CAT, POD, and SOD in soybean. The varying responses in enzyme activities can depend on the plant species, the intensity of the stress, and the level of ROS production [59]. Additionally, these differences may result from the strong influence of L-asp, as opposed to L-glu, on the antioxidant mechanism, or from the synergistic effects of both amino acids. This highlights L-asp+L-glu’s role as a promising avenue for developing strategies aimed at alleviating the adverse impacts of heavy metal stress on plants. In plants, glutamate and aspartate are precursors to approximately eleven amino acids. The foliar application of glutamate and aspartate together to the plant leaves do not increase the quantity of these eleven amino acids but were metabolized the increase in proline concentration [42]. Proline is known to protect plants under stress by reducing lipid peroxidation and enhancing antioxidant metabolism, among other processes [60,61]. In this study, it is likely that the combined application of L-asp and L-glu enhances the beneficial effects due to the increased levels of proline.
Antioxidant enzyme genes showed generally parallel results with antioxidant enzymes. In beans, distinct expression patterns of the PvSOD, PvPOD and PvAPX genes were observed under various abiotic stress conditions, indicating that these genes may have different roles in mitigating ROS generated by different environmental stresses [62,63]. Of note, the expression pattern of PvSOD is also interesting; in particular, moderate (A1) L-asp+L-glu pretreatment decreased PvSOD expression by Ni2+ treatment while others showed a rising trend. This may be related the insufficiency of low dose of L-asp+L-glu in reducing Ni2+ toxicity. On the other hand, the considerable variability in PvSOD, PvPOD and PvAPX expression profiles suggests the possibility that this effect might be random.
A completely different trend in the expressions of PvMTPs was detected in both genotypes. These differences encompass both heavy metals and L-asp+L-glu treatments. A notable up-regulation of the PvMTP10 gene was observed especially under Cd2+ and Ni2+ stresses in Elkoca and Gina, respectively, aligning with earlier research findings that underscore the involvement of this gene in the detoxification of Cd2+ [64]. In plants, MTP substrates and the heavy metals they are responsible for transporting are diverse. For instance, Helianthus annus MTP10 is involved in Cd2+ antiporter [64], whereas Taxus media MTP1 and MTP11 known to play a role in Cd2+ detoxification [65]. Similar to our study, the expression of the PvMTP11 gene notably upregulated in response to Cd2+ exposure, particularly in Gina. The upregulation of OsMTP11 expression was markedly observed in response to treatments involving Cd, Zn, Ni, and Mn, indicating its likely involvement in mediating cellular responses to various heavy metal exposures [9]. Additionally, in apples (Malus domestica), the induction of MdMTP11.1 expression was noted upon exposure to multiple metal ions, including Al, Cd, Cu, Fe, Mn, and Se [66]. MTPs are recognized for their established role in mitigating the lead toxicity as documented by Aslam et al. [26]. More MTP-encoding genes were upregulated in Medicago sativa under Pb stress [67]. Furthermore, the potential role of amino acid biostimulants on the activation of MTPs remains unexplored in P. vulgaris. In this study, where the expression profiles of PvMTP genes were determined for the first time through L-asp+L-glu treatments, the notable increase was detected in PvMTPs gene expressions in response to L-asp+L-glu treatments compared to stress conditions. Amino acids have a role in heavy metal transportation within cells. They form high-affinity binding sites with metal ions, facilitating their transport and regulation. Certain amino acids like asparagine, histidine, and glutamate are involved in metal complex equilibria in plant xylem fluids, influencing metal uptake and transport. Furthermore, phytochelatins and glutathione, which are synthesized from amino acids like methionine and glutamate, play a significant role in metal binding and detoxification in plants. These amino acids help in the chelation of heavy metals like Pb2+, Cd2+ and As3+, thereby contributing to the cell’s defense against heavy metal stress [8]. Therefore, this study suggests a potential contribution of L-asp+L-glu to the functionality of the MTP gene family.
Taken together, in the heatmap analysis, the clustering of As3+ treatments in a distinct group was attributed to the substantial upregulation of the PvSOD gene, while Ni2+ treatments were grouped in a different cluster due to the marked increase in PvAPX gene expression. Conversely, Cd2+ and Pb2+ treatments were placed in the same cluster as they induced similar increases or decreases in the parameters. There were evidences that As3+ stress significantly enhances SOD activity [68], while Ni2+ stress strongly increases POD activity [69]. Also, it can be suggested that L-asp+L-glu treatments, mitigated the harmful effects of heavy metals, particularly by increasing SPAD and RWC values and partially enhancing antioxidant enzyme activities, especially POD and APX.
In conclusion, the alleviative role of L-asp+L-glu treatments under heavy metal stresses demonstrated significant improvements in various physio-biochemical parameters. Notably, the distinct responses of various PvMTPs highlight the complexity of the regulatory networks orchestrating metal homeostasis. This suggests that L-asp+L-glu treatments may have enhanced the plant’s ability to sequester heavy metals into vacuoles, reducing their toxicity and improving overall plant health and also potential avenues for developing sustainable practices in agriculture through the use of combined L-asp+L-glu as a stress ameliorating agent. Furthermore, the highest expression of PvMTP10 observed through qPCR analysis under treatments with L-asp+L-glu, Pb2+, Cd2+, As3+, and Ni2+ demonstrated that it is a suitable candidate gene for developing heavy metal-resistant bean genotypes, particularly Elkoca genotype.
Acknowledgement: I am grateful for Emre Ilhan and Ataturk University Research Laboratories.
Funding Statement: The author received no specific funding for this study.
Availability of Data and Materials: All data are available in this article.
Ethics Approval: Not applicable.
Conflicts of Interest: The author declares that she has no conflicts of interest to report regarding the present study.
References
1. Aldoobie NF, Beltagi MS. Physiological, biochemical and molecular responses of common bean (Phaseolus vulgaris L.) plants to heavy metals stress. Afr J Biotechnol. 2013;12(29):4614–22. doi:10.5897/ajb2013.12387. [Google Scholar] [CrossRef]
2. Kolaj-Robin O, Russell D, Hayes KA, Pembroke JT, Soulimane T. Cation diffusion facilitator family: structure and function. FEBS Lett. 2015;589(12):1283–95. doi:10.1016/j.febslet.2015.04.007. [Google Scholar] [PubMed] [CrossRef]
3. Zhang H, Zhang LL, Li J, Chen M, An RD. Comparative study on the bioaccumulation of lead, cadmium and nickel and their toxic effects on the growth and enzyme defence strategies of a heavy metal accumulator, Hydrilla verticillata (Lf) Royle. Environ Sci Pollut Res. 2020;27:9853–5. doi:10.1007/s11356-019-06968-0. [Google Scholar] [PubMed] [CrossRef]
4. Balali-Mood M, Naseri K, Tahergorabi Z, Khazdair MR, Sadeghi M. Toxic mechanisms of five heavy metals: mercury, lead, chromium, cadmium, and arsenic. Frontiers Pharmacol. 2021;12:643972. doi:10.3389/fphar.2021.643972. [Google Scholar] [PubMed] [CrossRef]
5. Madhu PM, Sadagopan RS. Effect of heavy metals on growth and development of cultivated plants with reference to cadmium, chromium and lead—a review. J Stress Physiol Biochem. 2020;16(3):84–102. [Google Scholar]
6. Alloway BJ. Sources of heavy metals and metalloids in soils. In: Alloway BJ, editor. Heavy metals in soils: trace metals and metalloids in soils and their bioavailability. Dordrecht: Environmental Pollution; 2012. p. 11–50. [Google Scholar]
7. Capuana M. Heavy metals and woody plants-biotechnologies for phytoremediation. J Geophys Res Biogeosci. 2011;4:7–15. doi:10.3832/ifor0555-004. [Google Scholar] [CrossRef]
8. Sharma SS, Dietz KJ. The significance of amino acids and amino acid-derived molecules in plant responses and adaptation to heavy metal stress. J Exp Bot. 2006;57(4):711–26. doi:10.1093/jxb/erj073. [Google Scholar] [PubMed] [CrossRef]
9. Zhang M, Liu B. Identification of a rice metal tolerance protein OsMTP11 as a manganese transporter. PLoS One. 2017;12(4):e0174987. doi:10.1371/journal.pone.0174987. [Google Scholar] [PubMed] [CrossRef]
10. Farooq S, Siddiqui PJA. Distribution of chlorophyll in the mangrove sediments of Sonmiani bay. Pakistan Pak J Bot. 2011;43(1):405–10. [Google Scholar]
11. DalCorso G. Heavy metal toxicity in plants. In: Plants and heavy metals. Dordrecht: SpringerBriefs in Molecular Science; 2012. p. 1–25. [Google Scholar]
12. Gill M. Heavy metal stress in plants: a review. Int J Adv Res. 2014;2(6):1043–55. [Google Scholar]
13. AbdElgawad H, Zinta G, Hamed BA, Selim S, Beemster G, Hozzein WN, et al. Maize roots and shoots show distinct profiles of oxidative stress and antioxidant defense under heavy metal toxicity. Environ Pollut. 2020;258:113705. doi:10.1016/j.envpol.2019.113705. [Google Scholar] [PubMed] [CrossRef]
14. Hindt MN, Guerinot ML. Getting a sense for signals: regulation of the plant iron deficiency response. BBA Mol Cell Res. 2012;1823(9):1521–30. doi:10.1016/j.bbamcr.2012.03.010. [Google Scholar] [PubMed] [CrossRef]
15. Ricachenevsky FK, Menguer PK, Sperotto RA, Williams LE, Fett JP. Roles of plant metal tolerance proteins (MTP) in metal storage and potential use in biofortification strategies. Front Plant Sci. 2013;4:144. doi:10.3389/fpls.2013.00144. [Google Scholar] [PubMed] [CrossRef]
16. Montanini B, Blaudez D, Jeandroz S, Sanders D, Chalot M. Phylogenetic and functional analysis of the Cation Diffusion Facilitator (CDF) family: improved signature and prediction of substrate specificity. BMC Genom. 2007;8(1):1–16. doi:10.1186/1471-2164-8-107. [Google Scholar] [PubMed] [CrossRef]
17. Drager DB, Desbrosses-Fonrouge AG, Krach C, Chardonnens AN, Meyer RC, Saumitou-Laprade P, et al. Two genes encoding Arabidopsis halleri MTP1 metal transport proteins co-segregate with zinc tolerance and account for high MTP1 transcript levels. Plant J. 2004;39(3):425–39. doi:10.1111/j.1365-313X.2004.02143.x. [Google Scholar] [PubMed] [CrossRef]
18. van der Zaal BJ, Neuteboom LW, Pinas JE, Chardonnens AN, Schat H, Verkleij JA, et al. Overexpression of a novel Arabidopsis gene related to putative zinc-transporter genes from animals can lead to enhanced zinc resistance and accumulation. Plant Physiol. 1999;119(3):1047–56. doi:10.1104/pp.119.3.1047. [Google Scholar] [PubMed] [CrossRef]
19. Gao Y, Yang F, Liu J, Xie W, Zhang L, Chen Z, et al. Genome-wide identification of metal tolerance protein genes in Populus trichocarpa and their roles in response to various heavy metal stresses. Int J Mol Sci. 2020;21(15):1680. doi:10.3390/ijms21051680. [Google Scholar] [PubMed] [CrossRef]
20. Vatansever R, Filiz E, Eroglu S. Genome-wide exploration of metal tolerance protein (MTP) genes in common wheat (Triticum aestivum): insights into metal homeostasis and biofortification. Biometals. 2017;30:217–35. doi:10.1007/s10534-017-9997-x. [Google Scholar] [PubMed] [CrossRef]
21. Liu J, Gao Y, Tang Y, Wang D, Chen X, Yao Y, et al. Genome-wide identification, comprehensive gene feature, evolution, and expression analysis of plant metal tolerance proteins in tobacco under heavy metal toxicity. Front Genet. 2019;10:345. doi:10.3389/fgene.2019.00345. [Google Scholar] [PubMed] [CrossRef]
22. El-Sappah AH, Abbas M, Rather SA, Wani SH, Soaud N, Noor Z, et al. Genome-wide identification and expression analysis of metal tolerance protein (MTP) gene family in soybean (Glycine max) under heavy metal stress. Mol Biol Rep. 2023;50(4):2975–90. doi:10.1007/s11033-022-08100-x. [Google Scholar] [PubMed] [CrossRef]
23. Haque AM, Rahman MA, Das U, Rahman MM, Elseehy MM, El-Shehawi AM, et al. Changes in physiological responses and MTP (metal tolerance protein) transcripts in soybean (Glycine max) exposed to differential iron availability. Plant Physiol Biochem. 2022;179:1–9. doi:10.1016/j.plaphy.2022.03.007. [Google Scholar] [PubMed] [CrossRef]
24. Silva-Gigante M, Hinojosa-Reyes L, Rosas-Castor JM, Quero-Jiménez PC, Pino-Sandoval DA, Guzmán-Mar JL. Heavy metals and metalloids accumulation in common beans (Phaseolus vulgaris L.): a review. Chemosphere. 2023;139010. doi:10.1016/j.chemosphere.2023.139010. [Google Scholar] [PubMed] [CrossRef]
25. Eid EM, Shaltout KH, Alamri SAM, Sewelam NA, Galal TM. Evaluating the uptake of ten heavy metals by kidney bean (Phaseolus vulgaris L.) grown in a soil-sludge mixture using a regression model. Appl Ecol Env Res. 2020;18(5):7021–39. doi:10.15666/aeer/1805_70217039. [Google Scholar] [CrossRef]
26. Aslam M, Aslam A, Sheraz M, Ali B, Ulhassan Z, Najeeb U, et al. Lead toxicity in cereals: mechanistic insight into toxicity, mode of action, and management. Front Plant Sci. 2021;11:587785. doi:10.3389/fpls.2020.587785. [Google Scholar] [PubMed] [CrossRef]
27. Cao M, Song C, Jin Y, Liu L, Liu J, Xie H, et al. Synthesis of poly(γ-glutamic acid) and heterologous expression of pgsBCA genes. J Mol Catal B Enzym. 2010;67:111–6. doi:10.1016/j.molcatb.2010.07.014. [Google Scholar] [CrossRef]
28. Rai VK. Role of amino acids in plant responses to stresses. Biologia Plant. 2002;45(4):481–7. doi:10.1023/A:1022308229759. [Google Scholar] [CrossRef]
29. Leita L, De Nobili M, Mondini C, Garcia MB. Response of Leguminosae to cadmium exposure. J Plant Nutr. 1993;16(10):2001–12. doi:10.1080/01904169309364670. [Google Scholar] [CrossRef]
30. Piechalak A, Malecka A, Barałkiewicz D, Tomaszewska B. Lead uptake, toxicity and accumulation in Phaseolus vulgaris plants. Biol Plant. 2008;52:565–8. doi:10.1007/s10535-008-0112-6. [Google Scholar] [CrossRef]
31. Carbonell-Barrachina AA, Burló-Carbonell F, Mataix-Beneyto J. Arsenic uptake, distribution, and accumulation in bean plants: effect of arsenite and salinity on plant growth and yield. J Plant Nutr. 1997;20(10):1419–30. doi:10.1080/01904169709365344. [Google Scholar] [CrossRef]
32. Khalil RR, Moustafa AN, Bassuony FM, Haroun SA. Kinetin and/or calcium affect growth of Phaseolus vulgaris L. plant grown under heavy metals stress. J Environ Sci. 2017;46(2):103–20. doi:10.1016/j.jafr.2021.100182. [Google Scholar] [CrossRef]
33. Hoagland DR, Arnon DI. Growing plants without soil by the water-culture method. Berkeley, CA, USA: Davis Libraries, College of Agriculture, University of California; 1950. [Google Scholar]
34. Barrs HD, Weatherley PE. A re-examination of the relative turgidity technique for estimating water deficits in leaves. Aust J Biol Sci. 1962;15(3):413–28. doi:10.1071/bi9620413. [Google Scholar] [CrossRef]
35. Karlidag H, Esitken A, Yildirim E, Donmez MF, Turan M. Effects of plant growth promoting bacteria on yield, growth, leaf water content, membrane permeability, and ionic composition of strawberry under saline conditions. J Plant Nutr. 2010;34(1):34–45. doi:10.1080/01904167.2011.531357. [Google Scholar] [CrossRef]
36. Velikova V, Yordanov I. Edreva AJPS oxidative stress and some antioxidant systems in acid rain-treated bean plants: protective role of exogenous polyamines. Plant Sci. 2000;151(1):59–66. doi:10.1016/s0168-9452(99)00197-1. [Google Scholar] [CrossRef]
37. Heath RL, Packer L. Photoperoxidation in isolated chloroplasts: I. Kinetics and stoichiometry of fatty acid peroxidation. Arch Biochem Biophys. 1968;125(1):189–98. doi:10.1016/j.abb.2022.109248. [Google Scholar] [PubMed] [CrossRef]
38. Giannopolitis CN, Ries SK. Superoxide dismutases: I. Occurrence in higher plants. Plant Physiol. 1977;59(2):309–14. doi:10.1104/pp.59.2.309. [Google Scholar] [PubMed] [CrossRef]
39. Shams M, Ekinci M, Turan M, Dursun A, Kul R, Yildirim E. Growth, nutrient uptake and enzyme activity response of Lettuce (Lactuca sativa L.) to excess copper. Environ Sustain. 2019;2:67–73. doi:10.1007/s42398-019-00051-7. [Google Scholar] [CrossRef]
40. Murshed R, Lopez-Lauri F, Keller C, Monnet F, Sallanon H. Acclimation to drought stress enhances oxidative stress tolerance in Solanum lycopersicum L. fruits. Plant Stress. 2008;2(2):145–51. [Google Scholar]
41. Alengebawy A, Abdelkhalek ST, Qureshi SR, Wang MQ. Heavy metals and pesticides toxicity in agricultural soil and plants: ecological risks and human health implications. Toxics. 2021;9(3):42. doi:10.3390/toxics9030042. [Google Scholar] [PubMed] [CrossRef]
42. Alfosea-Simón M, Simón-Grao S, Zavala-Gonzalez EA, Cámara-Zapata JM, Simón I, Martínez-Nicolás JJ, et al. Physiological, nutritional and metabolomic responses of tomato plants after the foliar application of amino acids aspartic acid, glutamic acid and alanine. Front Plant Sci. 2021;11:581234. doi:10.3389/fpls.2020.581234. [Google Scholar] [PubMed] [CrossRef]
43. Kaymakanova M, Stoeva N. Physiological reaction of bean plants (Phaseolus vulg. L.) to salt stress. Gen Appl Plant Physiol. 2008;34:177–88. [Google Scholar]
44. Aygören AS, Güneş E, Muslu S, Kasapoğlu AG, Yiğider E, Aydın M, et al. Genome-wide analysis and characterization of SABATH gene family in Phaseolus vulgaris genotypes subject to melatonin under drought and salinity stresses. Plant Mol Biol Rep. 2023;41(2):242–59. doi:10.1007/s11105-022-01363-5. [Google Scholar] [CrossRef]
45. Niyoifasha CJ, Borena BM, Ukob IT, Minh PN, Al Azzawi TNI, Imran M, et al. Alleviation of Hg-, Cr-, Cu-, and Zn-induced heavy metals stress by exogenous sodium nitroprusside in rice plants. Plants. 2023;12(6):1299. doi:10.3390/plants12061299. [Google Scholar] [PubMed] [CrossRef]
46. Hammami H, Parsa M, Bayat H, Aminifard MH. The behavior of heavy metals in relation to their influence on the common bean (Phaseolus vulgaris) symbiosis. Environ Exp Bot. 2022;193:104670. doi:10.1016/j.envexpbot.2021.104670. [Google Scholar] [CrossRef]
47. Zubair M, Shafqat A, Jabben N, Shafiq M, Balal RM, Tahir MA, et al. Enhancing cabbage resilience against heavy metal stress through silicon amendments and melatonin: a depth investigation. Sci Hortic. 2024;324:112571. doi:10.1016/j.scienta.2023.112571. [Google Scholar] [CrossRef]
48. Qiu Y, Cai N, Wu Q. Effect of Pb on root growth and plant photosynthesis of sweetpotato. Acta Agric Zhejiangensis. 2006;18(6):429–32. doi:10.1360/yc-006-1280. [Google Scholar] [CrossRef]
49. Franzoni G, Cocetta G, Ferrante A. Effect of glutamic acid foliar applications on lettuce under water stress. Physiol Mol Biol Plants. 2021;27:1059–72. doi:10.1007/s12298-021-00984-6. [Google Scholar] [PubMed] [CrossRef]
50. Rizwan M, Ali S, Zaheer Akbar M, Shakoor MB, Mahmood A, Ishaque W, et al. Foliar application of aspartic acid lowers cadmium uptake and Cd-induced oxidative stress in rice under Cd stress. Environ Sci Pollut Res. 2017;24:21938–47. doi:10.1007/s11356-017-9860-1. [Google Scholar] [PubMed] [CrossRef]
51. Amin AA, Abouziena HF, Abdelhamid MT, Rashad ESM, Gharib AE. Improving growth and productivity of faba bean plants by foliar application of thiourea and aspartic acid. Int J Plant Soil Sci. 2014;3:724–36. doi:10.9734/IJPSS/2014/8227. [Google Scholar] [CrossRef]
52. Quilambo OA. Proline content, water retention capability and cell membrane integrity as parameters for drought tolerance in two peanut cultivars. S Afr J Bot. 2004;70(2):227–34. doi:10.1016/S0254-6299(15)30239-8. [Google Scholar] [CrossRef]
53. Rai N, Rai SP, Sarma BK. Prospects for abiotic stress tolerance in crops utilizing phyto-and bio-stimulants. Front Sustaina Food Syst. 2021;5:754853. doi:10.3389/fsufs.2021.754853. [Google Scholar] [CrossRef]
54. Demidchik V, Straltsova D, Medvedev SS, Pozhvanov GA, Sokolik A, Yurin V. Stress-induced electrolyte leakage: the role of K+-permeable channels and involvement in programmed cell death and metabolic adjustment. J Exp Bot. 2014;65(5):1259–70. doi:10.1093/jxb/eru004. [Google Scholar] [PubMed] [CrossRef]
55. Dhiman S, Sharma P, Bhardwaj T, Devi K, Khanna K, Kapoor N, et al. Role of potassium in heavy metal stress. In: Iqbal N, Umar S, editors. Role of potassium in abiotic stress. Singapore: Springer; 2022. p. 163–82. [Google Scholar]
56. Asgher M, Sehar Z, Rehaman A, Rashid S, Ahmed S, Per TS, et al. Exogenously-applied L-glutamic acid protects photosynthetic functions and enhances arsenic tolerance through increased nitrogen assimilation and antioxidant capacity in rice (Oryza sativa L.). Environ Pollut. 2022;301:119008. doi:10.1016/j.envpol.2022.119008. [Google Scholar] [PubMed] [CrossRef]
57. Huang S, Yang X, Chen G, Wang X. Application of glutamic acid improved As tolerance in aromatic rice at early growth stage. Chemosphere. 2023;322:138173. doi:10.1016/j.chemosphere.2023.138173. [Google Scholar] [PubMed] [CrossRef]
58. Teixeira WF, Fagan EB, Soares LH, Umburanas RC, Reichardt K, Neto DD. Foliar and seed application of amino acids affects the antioxidant metabolism of the soybean crop. Front Plant Sci. 2017;8:1–14. doi:10.3389/fpls.2017.00327. [Google Scholar] [PubMed] [CrossRef]
59. Bian S, Jiang Y. Reactive oxygen species, antioxidant enzyme activities and gene expression patterns in leaves and roots of Kentucky bluegrass in response to drought stress and recovery. Sci Hortic. 2009;120(2):264–70. doi:10.1016/j.scienta.2008.10.014. [Google Scholar] [CrossRef]
60. Islam MM, Hoque MA, Okuma E, Banu MNA, Shimoishi Y, Nakamura Y, et al. Exogenous proline and glycinebetaine increase antioxidant enzyme activities and confer tolerance to cadmium stress in cultured tobacco cells. J Plant Physiol. 2009;166(15):1587–97. doi:10.1016/j.jplph.2009.04.002. [Google Scholar] [PubMed] [CrossRef]
61. Hoque MA, Okuma E, Banu MNA, Nakamura Y, Shimoishi Y, Murata Y. Exogenous proline mitigates the detrimental effects of salt stress more than exogenous betaine by increasing antioxidant enzyme activities. J Plant Physiol. 2007;164(5):553–61. doi:10.1016/j.jplph.2006.03.010. [Google Scholar] [PubMed] [CrossRef]
62. Mohammadi M, Tavakoli A, Pouryousef M, Mohseni Fard E. Study the effect of 24-epibrassinolide application on the Cu/Zn-SOD expression and tolerance to drought stress in common bean. Physiol Mol Biol Plants. 2020;26(3):459–74. doi:10.1007/s12298-020-00757-7. [Google Scholar] [PubMed] [CrossRef]
63. Silva SAF, Silva FLB, Ribas AF, de Souza GH, dos Santos TB. Genome-wide in silico analysis of SOD genes in common bean (Phaseolus vulgaris L.). J Crop Sci Biotechnol. 2020;23:241–51. doi:10.1007/s12892-020-00030-z. [Google Scholar] [CrossRef]
64. Li J, Abbas M, Desoky ESM, Zafar S, Soaud SA, Syed S, et al. Analysis of metal tolerance protein (MTP) family in sunflower (Helianthus annus L.) and role of HaMTP10 as Cadmium antiporter under moringa seed extract. Ind Crops Prod. 2023;202:117023. doi:10.1016/j.indcrop.2023.117023. [Google Scholar] [CrossRef]
65. Feng S, Hou K, Zhang H, Chen C, Huang J, Wu Q, et al. Investigation of the role of tmmyb16/123 and their targets (tmmtp1/11) in the tolerance of Taxus media to cadmium. Tree Physiol. 2023;43:1009–22. doi:10.1093/treephys/tpad019. [Google Scholar] [PubMed] [CrossRef]
66. Qi D, Wang L, Liang M, Zhang Q, Tang X, Geng B, et al. Genome-wide analyses of metal tolerance protein genes in apple (Malus domestica): identification, characterization, expression and response to various metal ion stresses. Environ Exp Bot. 2022;201:104948. doi:10.1016/j.envexpbot.2022.104948. [Google Scholar] [CrossRef]
67. Wang Y, Meng Y, Mu S, Yan D, Xu X, Zhang L, et al. Changes in phenotype and gene expression under lead stress revealed key genetic responses to lead tolerance in Medicago sativa L. Gene. 2021;791:145714. doi:10.1016/j.gene.2021.145714. [Google Scholar] [PubMed] [CrossRef]
68. Srivastava S, Sinha P, Sharma YK. Status of photosynthetic pigments, lipid peroxidation and anti-oxidative enzymes in Vigna mungo in presence of arsenic. J Plant Nutr. 2017;40(3):298–306. doi:10.1080/01904167.2016.1240189. [Google Scholar] [CrossRef]
69. Gajewska E, Skłodowska M. Effect of nickel on ROS content and antioxidative enzyme activities in wheat leaves. Biometals. 2007;20(1):27–36. doi:10.1007/s10534-006-9011-5. [Google Scholar] [PubMed] [CrossRef]
Cite This Article
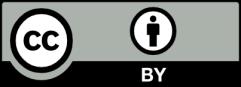
This work is licensed under a Creative Commons Attribution 4.0 International License , which permits unrestricted use, distribution, and reproduction in any medium, provided the original work is properly cited.