Open Access
ARTICLE
Arbuscular Mycorrhizal Fungi Improve Drought Tolerance of Quinoa Grown in Compost-Amended Soils by Altering Primary and Secondary Metabolite Levels
1 Centre d’Agrobiotechnologie et Bioingénierie, Unité de Recherche labellisée CNRST (Centre AgroBiotech-URL-CNRST-05), Université Cadi Ayyad, Marrakech, 40000, Morocco
2 Laboratory of Agro-Food, Biotechnologies and Valorization of Plant Bioresources (AGROBIOVAL), Department of Biology, Faculty of Science Semlalia, Cadi Ayyad University (UCA), Marrakech, 40000, Morocco
3 Tunisian-Moroccan laboratories (LMTM) of Plant Physiology and Biotechnology and Climate Change LPBV2C, Tunis, 1000, Tunisia
4 Faculty of Sciences, Laboratory of Plant, Animal, and Agro-Industry Productions, University Ibn Toufail, Kenitra, 14000, Morocco
5 Department of Biology, Pluridisciplinary Faculty of Nador, Mohammed First University, B.P. 300, Seloune, Nador, 62700, Morocco
6 Sustainable Agriculture Research Institute (ASARI), Mohammed VI Polytechnic University (UM6P), Laayoune, 70000, Morocco
7 Department of Plant Protection, Phytopathology Unit, Ecole Nationale d’Agriculture de Meknès, Km10, Rte Haj Kaddour, BPS/40, Meknès, 50001, Morocco
* Corresponding Authors: Rachid Lahlali. Email: ; Abdelilah Meddich. Email:
Phyton-International Journal of Experimental Botany 2024, 93(9), 2285-2302. https://doi.org/10.32604/phyton.2024.055052
Received 14 June 2024; Accepted 22 August 2024; Issue published 30 September 2024
Abstract
Quinoa (Chenopodium quinoa) has recently gained popularity as a pseudo-cereal cultivated in various countries due to the nutritional and antioxidant benefits of its seeds, and its capacity to persist in water-stressed environments. Our study aimed to assess the effects of native arbuscular mycorrhizal fungi (AMF) and local organic amendments on the metabolic responses and antioxidant activity of quinoa seeds under water-stressed conditions. To this end, quinoa plants were grown in soils inoculated with an indigenous mycorrhizal consortium AMF and amended with two types of compost from horse manure (HM) and green waste (GW) under two water regimes: well-watered (WW) 75% and 25% field capacity (FC). The primary metabolite contents (sugars and total protein) of quinoa seeds were measured. Additionally, the study involved identifying and quantifying secondary metabolites, particularly phenolic compounds (quercetin, vanillic acid, rutin, coumaric acid, kaempferol, and tetraterpenoids carotenoids) in quinoa seeds were determined using high-performance liquid chromatography (HPLC). The individual application of AMF and HM increased the total protein content in quinoa seeds by 8% and 6%, respectively, in contrast to the water-stressed control plants (WS). Conversely, the combined application of AMF, GW, and HM led to a 21% increase in sugar content compared to the control seeds under water-stressed conditions. Additionally, HPLC analysis identified five phenolic compounds, namely quercetin, kaempferol, vanillic acid, coumaric acid, and rutin. Under WS conditions, the application of biostimulants, whether used individually or in combination, brought about a rise in the identified phenolic compounds, except rutin, compared to controls. Consequently, these findings suggest that using AMF, either alone or in combination with HM and/or GW, can enhance the total protein content of quinoa seeds, help sustain higher levels of both primary and secondary metabolites under water stress conditions, thereby enhancing tolerance and reducing the detrimental impact of water stress on quinoa plants.Keywords
Quinoa (Chenopodium quinoa), which was first cultivated in the Andean region of South America, belongs to the amaranth family and the Chenopodiaceae subfamily [1–4]. Owing to its wide genetic diversity and resilience to the severe conditions of the Andean highlands, quinoa is thought to be resistant to temperature variations between day and night, as well as Abiotic factors, such as salinity, and drought [5,6]. Quinoa’s secondary metabolites, including tannins and flavonol glycosides [7,8], are pivotal in enhancing its tolerance to drought stress conditions [9]. These compounds act as antioxidants and protectants, playing a vital role in quinoa’s capacity to preserve cellular integrity and function under drought and other environmental stresses [10,11]. By scavenging reactive oxygen species and stabilizing cellular membranes, these metabolites contribute significantly to quinoa’s resilience and adaptability in diverse climatic conditions [12]. These secondary metabolites contribute to quinoa’s ability to combat oxidative stress and support overall health. In addition to its antioxidant properties, quinoa’s protein content is notably higher compared to many other cereals. It is regarded as a high-quality protein source due to its substantial levels of lysine and methionine [10–13]. Quinoa not only contains tannins and flavonol glycosides crucial for its resilience to drought stress but also boasts an array of antioxidants like ascorbic acid, phenolic compounds, and phytosterols. These antioxidants are valued for their ability to reduce inflammation and fight fungal infections, contributing to quinoa’s overall health benefits [14,15]. Furthermore, these antioxidants serve as immunological enhancers, potentially reducing the incidence of diabetes and cardiovascular disease [6–8]. It has been termed ‘one of the grains of the 21st century’ due to its resilience in adverse environmental circumstances and its outstanding functional and nutritional qualities [6]. Its antioxidant capacity is linked to its content of phenolic compounds, including kaempferol, quercetin, and α-tocopherols. These compounds interrupt the autoxidation chain reaction of fatty acids by interacting with fatty acid peroxyl radicals, leading to the production of stable, non-radical products [14–17].
Drought is recognized globally as a critical stress factor significantly limiting plant productivity across various regions of the world [18,19]. Drought stress happens when soil available water is reduced and the atmospheric conditions lead to continuous water loss through evaporation or transpiration [18,19]. This can result in oxidative stress if the reducing power produced by light-dependent reactions surpasses the rate at which it is used in the Calvin cycle [20]. Reactive oxygen species (ROS) can be overproduced due to an excess of reductants in the photosynthetic electron transport chain., which can cause additional damaging changes within the cell [21,22]. The mechanisms that plants have developed to avoid the negative effects of reactive oxygen species are crucial to their ability to withstand stressful conditions [23–25]. They built a range of complicated defense systems, including ROS scavenging mechanisms that consist of antioxidant enzymes and secondary metabolites [23–25]. In addition, alongside defensive strategies, several new biotechnologies have emerged to improve plant performance in drought environments. Lately, biostimulants have become widely recognized as natural and environmentally friendly methods for enhancing soil health and boosting crop resistance to environmental stresses like drought [26,27]. Applying arbuscular mycorrhizal fungi (AMF) is recommended as they improve nutrient uptake, soil porosity, structure, and water availability and efficiency [28]. AMF increases levels of metabolites and polyphenols through significant changes in physiological processes and enzymatic activity [29–31]. These fungi form symbiotic associations with plant roots to improve resistance to water stress and enhance nutrient uptake. This is achieved through mechanisms such as hormone regulation and alteration of gene expression pathways [32–34]. The synthesis of secondary compounds and polyphenols in plants is stimulated by this symbiotic interaction [35], which is crucial for their defense against environmental stressors and enhancement of nutritional quality [36,37]. Moreover, as a useful alternative to the over-reliance on chemical fertilizers for crops, organic compost is gaining popularity in today’s farming industry [38,39]. It is used to increase water retention, improve plant health and long-term soil fertility, minimize nutrient loss, and improve carbon content [27–40].
Extensive research on quinoa has primarily concentrated on its seed nutritional profile, protein quality, starch properties, and its application in food products derived from cereal flours [41,42]. However, few have evaluated the antioxidant activity and metabolite levels of quinoa seeds under stress and treated with biostimulants/biofertilizers. The effect of native AMF and local organic amendments on the metabolic responses and antioxidant activity of quinoa seeds under water-stressed conditions was assessed in this investigation.
The research aims to examine the effect of the AMF, either individually or in conjunction with organic amendments from green waste and/or horse manure, on the biochemical and metabolic characteristics of quinoa seeds under water-stressed conditions. We hypothesized that these biostimulants, used individually or in combination, might boost the synthesis of secondary metabolites lessen the detrimental consequences of water stress, and enhance the antioxidant capacity of water-stressed quinoa plants.
2.1 Protocols and Experimental Design
Quinoa seeds (Variety: pinu) were obtained from the National Institute for Agricultural Research (Marrakesh, Morocco). They were first immersed in a dilution of 5% sodium hypochlorite for 10 min. Then, they were immersed in distilled water for 10 min and rinsed five times to remove any remaining sodium hypochlorite. After disinfection, the seeds were placed in Petri dishes and incubated at 25°C for seven days. One plant per plastic bag containing three kilograms of soil previously sterilized for four hours at 180°C was used to transplant the evenly formed, pre-germinated seedlings. Soil physiochemical parameters are presented in the Table 1.
It was an experiment under controlled conditions in a greenhouse in Marrakech, Morocco, at Cadi Ayyad University. Two distinct water regimes were used: (1) well-watered (WW), which needed continuous irrigation at 75% of field capacity (FC), and (2) water-stressed (WS), which required decreased irrigation at 25% of FC. Each water regime included five treatments: C: non-inoculated and non-amended seedlings (Control), AMF: Seedlings inoculated with 60 g AMF inoculum containing about 100 spores and 2 g mycorrhizal root fragments (high AMF frequency (96%) and intensity (60%)) containing hyphae, vesicles and spores, GW: seedling treated with 5% of green waste compost, HM: seedling treated with 5% of compost from horse manure and AMFGWHM: the tripartite combination of AMF, GW and HM The study had fifteen duplicates of each treatment in a randomized experimental design. Plants were sustained for 4 months under controlled conditions with light (photon flux density ranged from the plants were cultivated under controlled conditions for 4 months, with a light photon flux density ranging from 330 µmol m2 s−1 and an average temperature of 25.5°C, and average relative humidity 65%). The TDR meter (Delta UK Ltd., Clacton-on-Sea, UK) was used to monitor soil moisture in all pots daily in the morning and evening. The amount of water required under the various water conditions was derived using the measured soil bulk density, field capacity, soil weight, moisture content and maximum soil moisture.
The isolation of the AMF consortium, known as Aoufous, was carried out in the Tafilalet region of Morocco., includes a mixture of 15 indigenous species: Rhizophagus intraradices, Claroideoglomus claroideum, Pacispora boliviana, Acaulospor, Acaulospora delicata, Acaulospora sp, Glomus macrocarpum, Glomus deserticola, Glomus claroides, Glomus aggregatum, Glomus clarum, Glomus versiforme, Glomus heterosporum, Glomus microcarpum, Glomus sp.
Zea mays L. was used as the host plant for a three-month period while AMF consortium was cultivated in a controlled greenhouse environment using trap culture in pots. The 5% sodium hypochlorite solution was used for the sterilisation of maize seeds by immersion in it for five minutes. Any remaining contamination was then carefully removed with sterile distilled water. To increase the density of the inoculum, the seeds were co-cultivated with the host plant in sterilized sand within a greenhouse environment. Once the roots were colonized by hyphae, vesicles, and spores, they were chopped into small segments and utilized as the inoculum. For the control plants, an equivalent amount of autoclaved mycorrhizal inoculum was applied [43].
2.3 Measurements of Protein Content and Total Soluble Sugar
The Bradford method [44] was utilized to quantify soluble protein content. One gram of seeds was homogenized in 4 mL of 1 M phosphate buffer (pH 7.2), then centrifuged at 18,000 × g for 15 min at 4°C. The supernatant was mixed with dye and transferred to spectrophotometer cuvettes, where the absorbance was measured at 595 nm.
Samples of seeds (0.1 g) were homogenized in 80% ethanol and centrifuged at 5000 rpm for 10 min. 0.25 mL phenol and 1.25 mL sulphuric acid were added to the resulting supernatant. The absorbance of the solution was measured at 485 nm for the determination of the total soluble sugar (TSS) content [45].
2.4 Determination of Total Carotenoid
Cold acetone and petroleum ether were used to determine total carotenoid content. At 450 nm, absorbance was measured. An extinction coefficient of 2500 was used to calculate the amount of carotenoids [46].
2.5 Preparation of Extract and Polyphenols Quantification
The preparation and extraction procedures were conducted according to the methods specified by Bahmanzadegan et al. [47], utilizing HPLC with an ultra-high-performance liquid chromatography (UHPLC) system equipped with a photodiode array detector. Elution was monitored at wavelengths of 279.5 and 280 nm. These techniques have been specifically designed to recognize and measure the phenolic compounds in quinoa seeds. Among the phenolic acids identified by HPLC in quinoa are Quercetine, Kamepferol, vanillic acid, coumaric acid and Rutin.
The statistical software SPSS version 23.0 (IBM, Armonk, NY, USA) was used to analyze the data. An analysis of variance (ANOVA) was performed on the data to look at changes over treatment. The mean separation was assessed using Tukey’s honest significant difference test at a significance level of 5% (p < 0.05).
3.1 Effect of Water Stress and Biostimulants on Carotenoid Content in Quinoa Seeds
Carotenoid content showed an increase under water stress (Fig. 1). Similarly, the use of biostimulants, either individually or in combination, notably enhanced the carotenoid content in quinoa seeds under (WW). Specifically, compared to untreated plants that received horse manure treatment (HM) and arbuscular mycorrhizal fungi combined with green waste and horse manure (AMFGWHM) showed a 34% and 26% a significant enhancement in carotenoid levels respectively. Similarly, they increased under WS in treated plants, particularly in HM with an improvement of 7% compared to the control plants.
Figure 1: The impact of two water regimes (WW: 75% FC, WS: 25% FC) on carotenoids content in seeds of control and treated quinoa plants. Means (±SD) within the same graph followed by different letters are significant at p < 0.05
3.2 Effect of Water Stress and Biostimulants on Osmolytes Accumulation in Quinoa Seeds
In all treatments, exposure to water stress raised total soluble sugars (TSS) and decreased protein content (see Table 2). The reduction in protein content was more pronounced in uninoculated and unmodified plants. However, biostimulants significantly increased both TSS and protein content in quinoa seeds under (WS) in comparison to the control. Specifically, protein content in quinoa plants treated with GW and AMF-GW-HM increased by 20% compared to untreated plants under well-watered (WW) conditions. The AMF-HM-GW combination also increased protein content in WW compared to the control. Table 2 shows that infected and treated plants had significantly higher TSS contents (p < 0.05) than untreated plants under both WW and water-stressed (WS) conditions. Under WS conditions, this accumulation reached higher values in AMF quinoa plants, with 8% improvement, and under WW conditions, in HM plants, with an improvement of 46% compared to their controls.
3.3 Effect of Water Stress and Biostimulants on Quercetin Content in Quinoa Seeds
Fig. 2 illustrates a notable rise in quercetin concentrations in response to water stress. The quantity of quercetin markedly increased in plants treated with biostimulants in comparison to the control. Under water stress (WS), plants treated with (GW) had the highest quercetin concentrations (114.6%), followed by plants treated with AMF alone (80.31%) and plants treated with AMF in combination with green water and humic substances (AMF-GWHM) (36.4%) in comparison with the control. In both well-watered (WW) and water-stressed conditions, GW-treated plants had the highest quercetin concentrations.
Figure 2: The impact of two water regimes (WW: 75% FC, WS: 25% FC) on quercetin content in seeds of control and treated quinoa plants. Means (±SD) within the same graph followed by different letters are significant at p < 0.05
3.4 Effect of Water Stress and Biostimulants on Kaempferol Content in Quinoa Seeds
Under water stress, the concentration of kaempferol in quinoa seeds has increased considerably (Fig. 3). Indeed, plants exposed to water stress and treated with biostimulants had the highest kaempferol concentration. The use of biostimulants, either individually or in combination, significantly enhanced kaempferol levels compared to untreated plants. Under (WS) conditions, the greatest kaempferol concentration was found in plants inoculated with the tripartite AMFGWHM combination, with an improvement by 298%, GW by 101% and AMF by 63% compared to the control.
Figure 3: The impact of two water regimes (WW: 75% FC, WS: 25% FC) on Kampferol content in seeds of control and treated quinoa plants. Means (±SD) within the same graph followed by different letters are significant at p < 0.05
3.5 Effect of Water Stress and Biostimulants on Vanillic Acid Content in Quinoa Seeds
Our finding showed that application of water stress results in Vanillic acid concentration rising., as shown in Fig. 4. Compared to the control plants, the use of biostimulants significantly improved this parameter. Under (WS), the highest vanillin acid concentrations were observed in the AMFGWHM-treated plants, which improved by 187% over the control. However, under both well-watered (WW) and water-stressed (WS) conditions, the application of AMF alone resulted in lower vanillic acid concentrations compared to other treatments.
Figure 4: The impact of two water regimes (WW: 75% FC, WS: 25% FC) on vanillic acid content in seeds of control and treated quinoa plants. Means (±SD) within the same graph followed by different letters are significant at p < 0.05
3.6 Effect of Water Stress and Biostimulants on Coumaric Acid Content in Quinoa Seeds
Fig. 5 shows that coumaric acid levels were affected by water stress. Applying biostimulants, alone or combined, significantly raised this parameter under water-stressed conditions compared to the control. The use of (GW) and (HM) resulted in the highest coumaric acid concentrations, with increases of 559.2% and 341.5%, respectively, compared to the water-stressed control plants.
Figure 5: The impact of two water regimes (WW: 75% FC, WS: 25% FC) on coumaric acid content in seeds of control and treated quinoa plants. Means (±SD) within the same graph followed by different letters are significant at p < 0.05
3.7 Effect of Water Stress and Biostimulants on Rutin Content in Quinoa Seeds
Water stress significantly lowered rutin concentrations in quinoa seeds. Green waste (GW) and horse manure (HM) resulted in a significant decrease in rutin content of 87.8% and 83.3%, respectively, as compared to control plants, as shown in Fig. 6.
Figure 6: The impact of two water regimes (WW: 75% FC, WS: 25% FC) on rutin content in seeds of control and treated quinoa plants. Means (±SD) within the same graph followed by different letters are significant at p < 0.05
Quinoa has recently been included in the list of healthy pseudo-cereals, and is acknowledged as one of the best sources of protein derived from plants [48,49]. In addition to containing high quality protein, they are a primary source of energy due to their starch content [47–50]. Quinoa carbohydrates can be regarded as a nutraceutical food due to their effects on lowering cholesterol, providing beneficial hypoglycemic effects, and reducing free fatty acids [41–51]. The bioactive substances in quinoa are mostly present in the seed’s outer layers, where they operate as a chemical barrier to keep out insects and microbes [41–50]. In quinoa seeds, more than 20 free and conjugated phenolic compounds have been identified. Major ones include flavonoids like kaempferol, quercetin and their glycosides, vanillic acid, ferulic acid and their derivatives [52,53]. Research has shown that the antioxidant potential of quinoa seeds correlates with their increased phenolic content [54,55]. The most common dietary phenolic components are phenolic acids and flavonoids, which are particularly abundant in quinoa seeds. Quinoa leaves and seeds contain significant bioactive substances that are beneficial to health. Free, esterified, or attached to elements of the cell wall [56], they can neutralise free radicals, block oxidases, stabilise free radicals, chelate metal ions and inhibit radical chain reactions [56–58]. They are commonly found in plants and, consequently, in plant-based foods. Consuming phenolic compounds can promote health and well-being by regulating glucose and lipid metabolism, boosting pancreatic β-cell activity, and increasing insulin secretion [59,60]. Quinoa seeds contain an extensive amount of flavonoids, including kaempferol and quercetin glycosides. The antioxidant activity of quinoa is likely due to non-phenolic substances, such as carotenoids [61]. Carotenoids are natural pigments that range in hue from yellow to reddish-orange, and give plant tissues their distinctive flavor. Besides from their structural functions, they are highly recognized for their antioxidant properties that prevent lipid peroxidation and maintain membrane stability. They are necessary for the non-radiative dissipation of surplus energy and for the assembly of the light-harvesting complex [62–68].
Our findings revealed that carotenoid levels in stressed quinoa increased under drought conditions. Tissue carotenoïdes concentrations can be changed by drought stress [69–71]. Although a decrease in leaf area may be associated with the drought-induced rise in carotenoid content, it may also be a defensive strategy meant to lessen the damaging impact of water stress [72,73]. The accumulation of carotenoids for osmotic regulation of drought-stressed plants in many crops has been reported [74–77]. Carotenoids serve a crucial function as photoprotective agents by quenching singlet oxygen generated from excessive light energy, thus preventing membrane damage. This aspect of carotenoid activity has been thoroughly examined in various other publications [78,79]. Additionally, the accumulation of carotenoids in stressed plants had a beneficial impact on their relative water content (RWC). This elevated RWC may stem from osmoregulation by osmoprotectants, since drought-stressed plants frequently accumulate carotenoids or sugars [80–83]. Whatever the water circumstances, biostimulants resulted in much higher carotenoid levels than untreated plants. The availability of nutrients is likely key to the synergistic effect of biostimulants in boosting total carotenoid concentrations. The significant release of minerals by compost and the improved mineral uptake facilitated by AMF are likely to contribute to increased levels of carotenoids in plants [84,85]. This is consistent with the above results. The combined application of AMF and compost is critical for reducing the negative effects of water stress in plants by raising carotenoid concentrations [70–86].
The leaves and grains of quinoa have been shown to have a higher nutritional value than other types of cereals. The protein in quinoa is a vital nutritional component and could make a significant contribution to reducing malnutrition in a wide range of populations [7–64]. Variations in protein content may be associated with cultivation under water stress, with the plant producing less protein than one grown under normal conditions [87–89]. Water stress negatively affects the protein content of quinoa seeds. However, the application of both biofertilizers improved this parameter. Proteins play a role in numerous processes by altering plant metabolism under stress and activating signaling in the plant defense network [87–90]. In our study, quinoa showed the maximum total soluble protein in treated plants, while the minimum was observed in untreated plants. Boutasknit et al. [91] discovered that the soluble protein content of Ceratonia siliqua decreased significantly under drought stress, while AMF and compost led to a significant increase in its protein content. In addition, Sheteiwy et al. [92] and Elsherpiny [93] suggested that AMF and compost enhance the accumulation of various proteins essential for plant metabolism. This variation in protein levels may clarify how the combination of AMF and compost improves the non-enzymatic antioxidant defense system under water stress [57]. Still, by boosting antioxidant enzyme activity in drought-stressed host plants, AMF in combination with compost can lessen oxidative stress [94]. Plants react to drought stress by increasing the concentration of osmoregulatory substances inside their cells [95]. These substances, such as soluble sugars, help regulate osmotic potential, maintain cell turgor pressure, scavenge free radicals, provide energy and carbon reserves, and offer protection to membrane structures and enzymes involved in metabolism [96–98]. In this experiment, water stress led to an increased accumulation of soluble carbohydrates in quinoa seeds. However, the level of these metabolites was more pronounced in quinoa treated by biofertilizers. Indeed, the mycorrhizal consortium and compost used enhance content of TSS in quinoa plants under both water regimes. The results suggested that the accumulations of carbohydrates and sugars are implicated in plant tolerance due to the greater photosynthetic capacity under water stress conditions. Ye et al. [99] demonstrated that mycorrhizal plants had increased levels of soluble sugars after drought, suggesting improved maintenance of photosynthetic capacity. The observation that AMF-plants sustain higher nitrogen metabolism and display reduced stress-induced accumulation of soluble proteins and amino acids compared to non-mycorrhizal plants supports this interpretation [100–102]. The accumulation of sugar suggests that AMF colonization improves enhances the host plant’s drought tolerance [103]. Ben-Laouane et al. [104] furthermore noted that, in water-stressed situations, AMF inoculation and compost amendment considerably raised chemical constituents like proline concentration, chlorophyll a, b, and total chlorophyll content. This enhancement improved osmoregulation in water-stressed alfalfa plants. Our findings show that combining AMF and compost before exposing quinoa seedlings to water stress affects the total soluble solids (TSS) content.
In crops, the synthesis and accumulation of polyphenols are generally triggered by abiotic stresses, such as water stress. Plants experiencing water stress can become significant sources of polyphenols by boosting the concentration of these compounds in their tissues, although this often results in reduced biomass production [105]. This increase in the phenolic content of quinoa seeds following the addition of biostimulants could be attributed to the positive effect of biostimulants in enhancing the accessibility of nutrients required by plants, and subsequently their uptake, transport and accumulation in plant tissues in full concentration, particularly phosphorus [106]. In the present study, Quercetin increases under stress conditions, particularly in plants inoculated with AMF and amended with compost/vermicompost, as previously reported [107,108]. Indeed, Mohammadi et al. [109] confirmed a greater increase in quercetin in stressed buckwheat compared to the unstressed plants, indicating that the synthesis of phenolic antioxidants such as quercetin can take place under stress conditions. This increase shows that mycorrhiza and compost (green waste and horse manure) act as moderators, encouraging the production of secondary metabolites under unfavorable conditions. In the same vein, under conditions of water stress, plants accumulated kaempferol content. The kaempferol content in the seeds of stressed plants was higher than in normal plants. The results of this investigation are in harmony with the study of Mahdi et al. [110] on Cichorium intybus L. Exposure to drought stress resulted in an increase in Kaempferol content. This flavonoid subclass has been shown to be essential for controlling plant-environment interactions, particularly when it comes to helping plants adapt to different abiotic stresses. ROS produced by adverse circumstances can generally be scavenged by flavonoids [111]. Quercetin derivatives are very helpful for buffering fluctuations in ROS homeostasis because of their chemical structure [112–115]. In addition to their antioxidant properties, they also act on α-glucosidase and prevent pancreatic lipase from functioning [51]. According to Sekhavatizadeh et al. [64], quinoa contains many derivatives of quercetin and kaempferol that are not found in regularly eaten grains. Vanillic acid and Coumaric acids present a similar result. It was clear from the data that water stress increased their content. Additionally, under stress conditions, rutin, which is one of the main phenols in quinoa, showed a significant decrease, especially in treated plants compared to the untreated ones. However, water stress significantly decreased the rutin content of quinoa seeds. These findings are consistent with those of Gharibi et al. [116]. Rutin content in quinoa seeds in this study exceeded 20 mg/100 g FW. Gharibi et al. [116] showed that variation in rutin content in quinoa seeds is related to stress conditions. Meanwhile, the amounts of quercetin, vanillic acid and coumaric acid also increased significantly in the treatments. These results are in line with what has been observed. The application of biostimulants has significantly increased the quantities of several phenols, particularly under water stress, giving quinoa plants the ability to resist better, especially under unfavorable conditions [117,118]. The objective of current study sought to assess the influence of indigenous biostimulants on the polyphenolic content of quinoa seeds, namely phenolic acids and flavonols, under both normal and water stressed circumstances.
The positive role of the biostimulants used on the quality of quinoa seeds under water stress was demonstrated. Both organic amendments and AMF improved the nutritional value of the seeds, as well as their antioxidant capacity. Interestingly, the quinoa plant’s response to water stress was more pronounced in plants treated with these biostimulants. and can be a key tool for quinoa’s tolerance to water stress via metabolic and biochemical mechanisms.
Acknowledgement: The current work was funded by the Tuniso-Moroccan Mixed Laboratories (LMTM) of Plant Physiology and Biotechnology and Climate Change LPBV2C (LR11ES09) and the FOSC Project (Sus-Agri-CC) from the European Union’s Horizon 2020 Research and Innovation Program under grant agreement No. 862555.
Funding Statement: The authors received no specific funding for this study.
Author Contributions: The authors confirm contribution to the paper as follows: study conception and design: Wissal Benaffari, Fatima-Ezzahra Soussani, Abir Ben Hassine, Hala Ben Ahmed; data collection: Wissal Benaffari; analysis and interpretation of results: Abderrahim Boutasknit, Fatima-Ezzahra Soussani, Salma Toubali; draft manuscript preparation: Wissal Benaffari, Abdelilah Meddich, Rachid Lahlali; Supervision: Abdelilah Meddich, Rachid Lahlali. All authors reviewed the results and approved the final version of the manuscript.
Availability of Data and Materials: All data generated or analyzed during this study are included in this published article.
Ethics Approval: None.
Conflicts of Interest: The authors declare that they have no conflicts of interest to report regarding the present study.
References
1. Bazile D, Jacobsen SE, Verniau A. The global expansion of quinoa: trends and limits. Front Plant Sci. 2016;7(608):622. doi:10.3389/fpls.2016.00622. [Google Scholar] [PubMed] [CrossRef]
2. Bruno MC. Archaeobotanical insights into Kañawa (Chenopodium pallidicaule Aellen) domestication: a rustic seed crop of the Andean Altiplano. Agronomy. 2023;13(8):2085. doi:10.3390/agronomy13082085. [Google Scholar] [CrossRef]
3. Maughan PJ, Jarvis DE, De E, Torres C, Jaggi KE, Warner HC, et al. North American pitseed goosefoot is a genetic resource to improve Andean quinoa (C. quinoa). Sci Rep. 2024;14(1):1–13. doi:10.1038/s41598-024-63106-8. [Google Scholar] [PubMed] [CrossRef]
4. Bazile D. Global trends in the worldwide expansion of. Biol Life Sci Forum. 2023;25:13. doi:10.3390/blsf2023025013. [Google Scholar] [CrossRef]
5. Maamri K, Zidane OD, Chaabena A, Fiene G, Bazile D. Adaptation of some quinoa genotypes (Chenopodium quinoa Willd.), grown in a Saharan climate in Algeria. Life. 2022;12(11):1854. doi:10.3390/life12111854. [Google Scholar] [PubMed] [CrossRef]
6. Sharma B, Sarvesh T, Ghaswa R, Kantwa CR. Quinoa 21st century golden grain for nutrition security and health benefits: a review. Int J Curr Microbiol Appl Sci. 2021;10(2):1076–83. doi:10.20546/ijcmas.2021.1002.127. [Google Scholar] [PubMed] [CrossRef]
7. Villacrés E, Quelal M, Galarza S, Iza D, Silva E. Nutritional value and bioactive compounds of leaves and grains from quinoa (Chenopodium quinoa Willd.). Plants. 2022;11(2):213. doi:10.3390/plants11020213. [Google Scholar] [PubMed] [CrossRef]
8. Ren G, Teng C, Fan X, Guo S, Zhao G, Zhang L, et al. Nutrient composition, functional activity and industrial applications of quinoa (Chenopodium quinoa Willd.). Food Chem. 2023;410(4):135290. doi:10.1016/j.foodchem.2022.135290. [Google Scholar] [PubMed] [CrossRef]
9. Samadi M, Kazemeini SA, Razzaghi F, Edalat M, Andersen MN, Jacobsen SE, et al. Melatonin priming manipulates antioxidant regulation and secondary metabolites production in favor of drought tolerance in Chenopodium quinoa Willd. S Afr J Bot. 2024;166(2):272–86. doi:10.1016/j.sajb.2024.01.044. [Google Scholar] [CrossRef]
10. Lin PH, Chao YY. Different drought-tolerant mechanisms in quinoa (Chenopodium quinoa Willd.) and djulis (Chenopodium formosanum Koidz.) based on physiological analysis. Plants. 2021;10(11):2279. doi:10.3390/plants10112279. [Google Scholar] [PubMed] [CrossRef]
11. Manaa A, Goussi R, Derbali W, Cantamessa S, Essemine J, Barbato R. Photosynthetic performance of quinoa (Chenopodium quinoa Willd.) after exposure to a gradual drought stress followed by a recovery period. Biochimica et Biophysica Acta (BBA)-Bioenergetics. 2021;1862(5):148383. doi:10.1016/j.bbabio.2021.148383. [Google Scholar] [PubMed] [CrossRef]
12. Kuktaite R, Repo-Carrasco-Valencia R, de Mendoza CC, Plivelic TS, Hall S, Johansson E. Innovatively processed quinoa (Chenopodium quinoa Willd.) food: chemistry, structure and end-use characteristics. J Sci Food Agric. 2022;102(12):5065–76. doi:10.1002/jsfa.11214. [Google Scholar] [PubMed] [CrossRef]
13. Mohamed Ahmed IA, Al Juhaimi F, Özcan MM. Insights into the nutritional value and bioactive properties of quinoa (Chenopodium quinoa): past, present and future prospective. Int J Food Sci Technol. 2021;56(8):3726–41. doi:10.1111/ijfs.15011. [Google Scholar] [CrossRef]
14. Jan N, Hussain SZ, Naseer B, Bhat TA. Amaranth and quinoa as potential nutraceuticals: a review of anti-nutritional factors, health benefits and their applications in food, medicinal and cosmetic sectors. Food Chem: X. 2023;18(3):100687. doi:10.1016/j.fochx.2023.100687. [Google Scholar] [PubMed] [CrossRef]
15. Shah S, Khan Y, Yan H. The phytochemical, pharmacological and medicina l evaluation of quinoa (Chenopodium quinoa Willd.). Pakistan J Weed Sci Res. 2022;28(2):138–58. doi:10.28941/pjwsr.v28i2.1049. [Google Scholar] [CrossRef]
16. Pereira E, Encina-Zelada C, Barros L, Gonzales-Barron U, Cadavez V, Ferreira CFR, et al. Chemical and nutritional characterization of Chenopodium quinoa Willd (quinoa) grains: a good alternative to nutritious food. Food Chem. 2019;280:110–4. doi:10.1016/j.foodchem.2018.12.068. [Google Scholar] [PubMed] [CrossRef]
17. Stikić RI, Milinčić DD, Kostić A, Jovanović ZB, Gašić UM, Tešić ŽL, et al. Polyphenolic profiles, antioxidant, and in vitro anticancer activities of the seeds of Puno and Titicaca quinoa cultivars. Cereal Chem. 2020;97(3):626–33. doi:10.1002/cche.10278. [Google Scholar] [CrossRef]
18. Ahluwalia O, Singh PC, Bhatia R. A review on drought stress in plants: implications, mitigation and the role of plant growth promoting rhizobacteria. Resour Environ Sustain. 2021;5(16):100032. doi:10.1016/J.RESENV.2021.100032. [Google Scholar] [CrossRef]
19. Chaudhry S, Sidhu GPS. Climate change regulated abiotic stress mechanisms in plants: a comprehensive review. Plant Cell Rep. 2022;41(1):1–31. doi:10.1007/s00299-021-02759-5. [Google Scholar] [PubMed] [CrossRef]
20. Savchenko T, Tikhonov K. Oxidative stress-induced alteration of plant central metabolism. Life. 2021;11(4):304. doi:10.3390/life11040304. [Google Scholar] [PubMed] [CrossRef]
21. Kesawat MS, Satheesh N, Kherawat BS, Kumar A, Kim H, Chung S, et al. Regulation of reactive oxygen species during salt stress in plants and their crosstalk with other signaling molecules—current perspectives and future directions. Plants. 2023;12(4):864. doi:10.3390/plants12040864. [Google Scholar] [PubMed] [CrossRef]
22. Suzuki N. Fine tuning of ROS, redox and energy regulatory systems associated with the functions of chloroplasts and mitochondria in plants under heat stress. Int J Mol Sci. 2023;24(2):1356. doi:10.3390/ijms24021356. [Google Scholar] [PubMed] [CrossRef]
23. Wu J, Wang J, Hui W, Zhao F, Wang P, Su C, et al. Physiology of plant responses to water stress and related genes: a review. Forests. 2022;13(2):324. doi:10.3390/f13020324. [Google Scholar] [CrossRef]
24. Zhang Y, Xu J, Li R, Ge Y, Li Y, Li R. Plants’ response to abiotic stress: mechanisms and strategies. Int J Mol Sci. 2023;24(13):1–17. doi:10.3390/ijms241310915. [Google Scholar] [PubMed] [CrossRef]
25. Salsinha YCF, Indradewa D, Purwestri YA, Rachmawati D. Physiological and oxidative defense responses of local rice cultivars Nusa Tenggara Timur-Indonesia during vegetative drought stress. Aust J Crop Sci. 2021;15(3):394–400. doi:10.21475/ajcs.21.15.03.p2851. [Google Scholar] [CrossRef]
26. Gujre N, Soni A, Rangan L, Tsang DCW, Mitra S. Sustainable improvement of soil health utilizing biochar and arbuscular mycorrhizal fungi: a review. Environ Pollut. 2021;268(4):115549. doi:10.1016/j.envpol.2020.115549. [Google Scholar] [PubMed] [CrossRef]
27. Tahat MM, Alananbeh KM, Othman YA, Leskovar DI. Soil health and sustainable agriculture. Sustain. 2020;12(12):4859. doi:10.3390/SU12124859. [Google Scholar] [CrossRef]
28. Bamdad H, Papari S, Lazarovits G, Berruti F. Soil amendments for sustainable agriculture: microbial organic fertilizers. Soil Use Manag. 2022;38(1):94–120. doi:10.1111/sum.12762. [Google Scholar] [CrossRef]
29. Fritz V, Tereucán G, Santander C, Contreras B, Cornejo P, Ferreira PAA, et al. Effect of inoculation with arbuscular mycorrhizal fungi and fungicide application on the secondary metabolism of solanum tuberosum leaves. Plants. 2022;11(3):278. doi:10.3390/plants11030278. [Google Scholar] [PubMed] [CrossRef]
30. Duc NH, Vo AT, Haddidi I, Daood H, Posta K. Arbuscular mycorrhizal fungi improve tolerance of the medicinal plant Eclipta prostrata (L.) and induce major changes in polyphenol profiles under salt stresses. Front Plant Sci. 2021;11:2209. doi:10.3389/FPLS.2020.612299/BIBTEX. [Google Scholar] [CrossRef]
31. de Assis RMA, Carneiro JJ, Medeiros APR, de Carvalho AA, da Cunha Honorato A, Carneiro MAC, et al. Arbuscular mycorrhizal fungi and organic manure enhance growth and accumulation of citral, total phenols, and flavonoids in Melissa officinalis L. Ind Crops Prod. 2020;158:112981. doi:10.1016/j.indcrop.2020.112981. [Google Scholar] [CrossRef]
32. Boutasknit A, Baslam M, Ait-El-Mokhtar M, Anli M, Ben-Laouane R, Ait-Rahou Y, et al. Assemblage of indigenous arbuscular mycorrhizal fungi and green waste compost enhance drought stress tolerance in carob (Ceratonia siliqua L.) trees. Sci Rep. 2021;11(1):22835. doi:10.1038/s41598-021-02018-3. [Google Scholar] [PubMed] [CrossRef]
33. Huang D, Ma M, Wang Q, Zhang M, Jing G, Li C, et al. Arbuscular mycorrhizal fungi enhanced drought resistance in apple by regulating genes in the MAPK pathway. Plant Physiol Biochem. 2020;149(2):245–55. doi:10.1016/j.plaphy.2020.02.020. [Google Scholar] [PubMed] [CrossRef]
34. Wang Y, Zou YN, Shu B, Wu QS. Deciphering molecular mechanisms regarding enhanced drought tolerance in plants by arbuscular mycorrhizal fungi. Sci Hortic. 2023;308:111591. doi:10.1016/j.scienta.2022.111591. [Google Scholar] [CrossRef]
35. Zhao Y, Cartabia A, Lalaymia I, Declerck S. Arbuscular mycorrhizal fungi and production of secondary metabolites in medicinal plants. Mycorrhiza. 2022;32(3):221–56. doi:10.1007/s00572-022-01079-0. [Google Scholar] [PubMed] [CrossRef]
36. Qaderi MM, Martel AB, Strugnell CA. Environmental factors regulate plant secondary metabolites. Plants. 2023;12(3):447. doi:10.3390/plants12030447. [Google Scholar] [PubMed] [CrossRef]
37. Robe K, Izquierdo E, Vignols F, Rouached H, Dubos C. The coumarins: secondary metabolites playing a primary role in plant nutrition and health. Trends Plant Sci. 2021;26(3):248–59. doi:10.1016/j.tplants.2020.10.008. [Google Scholar] [PubMed] [CrossRef]
38. Durán-Lara EF, Valderrama A, Marican A. Natural organic compounds for application in organic farming. Agric. 2020;10(2):1–22. doi:10.3390/agriculture10020041. [Google Scholar] [CrossRef]
39. Kang SW, Yun JJ, Park JH, Cho JS. Exploring suitable biochar application rates with compost to improve upland field environment. Agronomy. 2021;11(6):1136. doi:10.3390/agronomy11061136. [Google Scholar] [CrossRef]
40. Rivier PA, Jamniczky D, Nemes A, Makó A, Barna G, Uzinger N, et al. Short-term effects of compost amendments to soil on soil structure, hydraulic properties, and water regime. J Hydrol Hydromechanics. 2022;70(1):74–88. doi:10.2478/johh-2022-0004. [Google Scholar] [CrossRef]
41. Pathan S, Siddiqui RA. Nutritional composition and bioactive components in quinoa (Chenopodium quinoa Willd) greens: a review. Nutrients. 2022;14(3):1–12. doi:10.3390/nu14030558. [Google Scholar] [PubMed] [CrossRef]
42. Li L, Lietz G, Seal CJ. Phenolic, apparent antioxidant and nutritional composition of quinoa (Chenopodium quinoa Willd) seeds. Int J Food Sci Technol. 2021;56(7):3245–54. doi:10.1111/IJFS.14962. [Google Scholar] [CrossRef]
43. Meddich A, Jaiti F, Bourzik W, Asli AEl, Hafidi M. Use of mycorrhizal fungi as a strategy for improving the drought tolerance in date palm (Phoenix dactylifera). Sci Hortic. 2015;192(21):468–74. doi:10.1016/J.SCIENTA.2015.06.024. [Google Scholar] [CrossRef]
44. Bradford M. A rapid and sensitive method for the quantitation of microgram quantities of protein utilizing the principle of protein-dye binding. Anal Biochem. 1976;72(1–2):248–54. doi:10.1006/ABIO.1976.9999. [Google Scholar] [PubMed] [CrossRef]
45. DuBois M, Gilles KA, Hamilton JK, Rebers PA, Smith F. Colorimetric method for determination of sugars and related substances. Microb Cell Fact. 2002;8(3):59. doi:10.1021/AC60111A017. [Google Scholar] [CrossRef]
46. Rodriguez-amaya DB, Kimura M. Harvest plus handbook for carotenoid analysis. Washington, DC, Cali, Colombia: International Food Policy Research Institute (lFPRl) International Center for Tropical Agriculture (ClAT); 2004. vol. 2, no. 1, p. 63–77. doi:10.3141/2068-08. [Google Scholar] [PubMed] [CrossRef]
47. Bahmanzadegan A, Rowshan V, Zareiyan F, Hatami A. Lagoecia cuminoides L., its antioxidant activity and polyphenolic constituents from Iran. Nat Prod Res. 2019;33(16):2376–8. doi:10.1080/14786419.2018.1440232. [Google Scholar] [PubMed] [CrossRef]
48. Khan Y, Shah S, Tian H. The roles of arbuscular mycorrhizal fungi in influencing plant nutrients, photosynthesis, and metabolites of cereal crops—a review. Agronomy. 2022;12(9):2191. doi:10.3390/agronomy12092191. [Google Scholar] [CrossRef]
49. Afzal I, Haq MZU, Ahmed S, Hirich A, Bazile D. Challenges and perspectives for integrating quinoa into the agri-food system. Plants. 2023;12(19):1–23. doi:10.3390/plants12193361. [Google Scholar] [PubMed] [CrossRef]
50. Hussain MI, Farooq M, Syed QA, Ishaq A, Al-Ghamdi AA, Hatamleh AA. Botany, nutritional value, phytochemical composition and biological activities of quinoa. Plants. 2021;10(11):2258. doi:10.3390/plants10112258. [Google Scholar] [PubMed] [CrossRef]
51. Fischer S, Wilckens R, Jara J, Aranda M, Valdivia W, Bustamante L, et al. Protein and antioxidant composition of quinoa (Chenopodium quinoa Willd) sprout from seeds submitted to water stress, salinity and light conditions. Ind Crops Prod. 2017;107(2):558–64. doi:10.1016/j.indcrop.2017.04.035. [Google Scholar] [CrossRef]
52. Tang Y, Zhang B, Li X, Chen PX, Zhang H, Liu R, et al. Bound phenolics of quinoa seeds released by acid, alkaline, and enzymatic treatments and their antioxidant and α-Glucosidase and pancreatic lipase inhibitory effects. J Agric Food Chem. 2016;64(8):1712–9. doi:10.1021/acs.jafc.5b05761. [Google Scholar] [PubMed] [CrossRef]
53. Hernández-Ledesma B. Quinoa (Chenopodium quinoa Willd.) as a source of nutrients and bioactive compounds: a review. Bioact Compd Heal Dis. 2019;2(3):27–47. doi:10.31989/bchd.v2i3.556. [Google Scholar] [CrossRef]
54. Benaffari W, Meddich A. Inoculation of arbuscular mycorrhizal fungi and plant growth promoting rhizobacteria mediated water stress tolerance in quinoa seeds by modulating the phenolics compounds, stress markers, osmolytes accumulation and antioxidant defense. Gesunde Pflanz. 2023;75(5):2121–32. doi:10.1007/s10343-023-00849-w. [Google Scholar] [CrossRef]
55. Ramos-Pacheco BS, Choque-Quispe D, Ligarda-Samanez CA, Solano-Reynoso AM, Palomino-Rincón H, Choque-Quispe Y, et al. Effect of germination on the physicochemical properties, functional groups, content of bioactive compounds, and antioxidant capacity of different varieties of quinoa (Chenopodium quinoa Willd.) grown in the high Andean Zone of Peru. Foods. 2024;13(3):1–14. doi:10.3390/foods13030417. [Google Scholar] [PubMed] [CrossRef]
56. Vuolo MM, Lima VS, Maróstica Junior MR. Phenolic compounds: structure, classification, and antioxidant power. ‘Bioactive compounds’. Woodhead Publishing. Elsevier Inc.; 2019. p. 30–50. doi:10.1016/B978-0-12-814774-0.00002-5. [Google Scholar] [CrossRef]
57. Ivanova A, Gerasimova E, Gazizullina E. Study of antioxidant properties of agents from the perspective of their action mechanisms. Molecules. 2020;25(18):4251. doi:10.3390/molecules25184251. [Google Scholar] [PubMed] [CrossRef]
58. Rahman M, Rahaman S, Islam R, Rahman F, Mithi FM, Alqahtani T, et al. Role of phenolic compounds in human disease: current. Molecules. 2022;27(1):233. doi:10.3390/molecules27010233. [Google Scholar] [PubMed] [CrossRef]
59. Liu S, Yu J, Fu M, Wang X, Chang X. Regulatory effects of hawthorn polyphenols on hyperglycemic, inflammatory, insulin resistance responses, and alleviation of aortic injury in type 2 diabetic rats. Food Res Int. 2021;142(13):110239. doi:10.1016/j.foodres.2021.110239. [Google Scholar] [PubMed] [CrossRef]
60. Sun C, Zhao C, Guven EC, Paoli P, Simal-Gandara J, Ramkumar KM, et al. Dietary polyphenols as antidiabetic agents: advances and opportunities. Food Front. 2020;1(1):18–44. doi:10.1002/fft2.15. [Google Scholar] [CrossRef]
61. Ocampo M, Fischer S, Folch-cano C, Pinto A, Figueroa I. Content and antioxidant capacity of phenolic compounds in quinoa seed: a review. Chil J Agric Res. 2023;1(6):754–67. doi:10.4067/S0718-58392023000600754. [Google Scholar] [PubMed] [CrossRef]
62. Sharma G, Prakash D, Gupta C, Prakash D, Sharma G. Phytochemicals of nutraceutical importance: do they defend against diseases. Phytochem Nutraceutical. 2014:1. doi:10.1079/9781780643632.0000. [Google Scholar] [PubMed] [CrossRef]
63. Metibemu DS, Ogungbe IV. Carotenoids in drug discovery and medicine: pathways and molecular targets implicated in human diseases. Molecules. 2022;27(18):6005. doi:10.3390/molecules27186005. [Google Scholar] [PubMed] [CrossRef]
64. Sekhavatizadeh SS, Hosseinzadeh S, Mohebbi G. Nutritional, antioxidant properties and polyphenol content of quinoa (Chenopodium quinoa Willd.) cultivated in Iran. Futur Food J Food, Agric Soc. 2021;9:1–12. doi:10.17170/KOBRA-202102163256. [Google Scholar] [CrossRef]
65. Melini V, Melini F. Functional components and anti-nutritional factors in gluten-free grains: a focus on quinoa seeds. Foods. 2021;10(2):351. doi:10.3390/foods10020351. [Google Scholar] [PubMed] [CrossRef]
66. Hinojosa L, González JA, Barrios-Masias FH, Fuentes F, Murphy KM. Quinoa abiotic stress responses: a review. Plants. 2018;7(4):106. doi:10.3390/plants7040106. [Google Scholar] [PubMed] [CrossRef]
67. Demmig-Adams B, Adams WW. Photoprotection and other responses of plants to high light stress. Annu Rev Plant Physiol Plant Mol Biol. 1992;43(1):599–626. doi:10.1146/annurev.pp.43.060192.003123. [Google Scholar] [CrossRef]
68. González-Peña MA, Ortega-Regules AE, Anaya de Parrodi C, Lozada-Ramírez JD. Chemistry, occurrence, properties, applications, and encapsulation of carotenoids—a review. Plants. 2023;12(2):313. doi:10.3390/plants12020313. [Google Scholar] [PubMed] [CrossRef]
69. Benaffari W, Boutasknit A, Anli M, Ait-el-mokhtar M, Ait-Rahou Y, Ben-Laouane R, et al. The native arbuscular mycorrhizal fungi and vermicompost-based organic amendments enhance soil fertility, growth performance, and the drought stress tolerance of quinoa. Plants. 2022;11(3):393. doi:10.3390/plants11030393. [Google Scholar] [PubMed] [CrossRef]
70. Soussani FE, Boutasknit A, Ben-Laouane R, Benkirane R, Baslam M, Meddich A. Arbuscular mycorrhizal fungi and compost-based biostimulants enhance fitness, physiological responses, yield, and quality traits of drought-stressed tomato plants. Plants. 2023;12(9):1856. doi:10.3390/plants12091856. [Google Scholar] [PubMed] [CrossRef]
71. Ouhaddou R, Ben-Laouane R, Slimani A, Boutasknit A, Anli M, Oufdou K, et al. Autochthonous biostimulants as a promising biological tool to promote lettuce growth and development under salinity conditions. Environ Sci Proc. 2022;16(1):41. doi:10.3390/environsciproc2022016041. [Google Scholar] [CrossRef]
72. Munchinger I, Hajek P, Akdogan B, Caicoya AT, Kunert N. Leaf thermal tolerance and sensitivity of temperate tree species are correlated with leaf physiological and functional drought resistance traits. J For Res. 2023;34(1):63–76. doi:10.1007/s11676-022-01594-y. [Google Scholar] [CrossRef]
73. Akhter Ansari W, Atri N, Pandey M, Kumar Singh A, Singh B, Pandey S. Influence of drought stress on morphological, physiological and biochemical attributes of plants: a review. Biosci Biotechnol Res Asia. 2019;16(4):697–709. doi:10.13005/bbra/2785. [Google Scholar] [CrossRef]
74. Ali Q, Javed MT, Haider MZ, Habib N, Rizwan M, Perveen R, et al. α-tocopherol foliar spray and translocation mediates growth, photosynthetic pigments, nutrient uptake, and oxidative defense in Maize (Zea mays L.) under drought stress. Agronomy. 2020;10(9):1–27. doi:10.3390/agronomy10091235. [Google Scholar] [CrossRef]
75. Zafari M, Ebadi A, Jahanbakhsh S, Sedghi M. Safflower (Carthamus tinctorius) biochemical properties, yield, and oil content affected by 24-epibrassinosteroid and genotype under drought stress. J Agric Food Chem. 2020;68(22):6040–7. doi:10.1021/acs.jafc.9b06860. [Google Scholar] [PubMed] [CrossRef]
76. Kusvuran S. Microalgae (Chlorella vulgaris Beijerinck) alleviates drought stress of broccoli plants by improving nutrient uptake, secondary metabolites, and antioxidative defense system. Hortic Plant J. 2021;7(3):221–31. doi:10.1016/j.hpj.2021.03.007. [Google Scholar] [CrossRef]
77. Šola I, Stić P, Rusak G. Effect of flooding and drought on the content of phenolics, sugars, photosynthetic pigments and vitamin C, and antioxidant potential of young Chinese cabbage. Eur Food Res Technol. 2021;247(8):1913–20. doi:10.1007/s00217-021-03759-1. [Google Scholar] [CrossRef]
78. Swapnil P, Meena M, Singh SK, Dhuldhaj UP, Harish, Marwal A. Vital roles of carotenoids in plants and humans to deteriorate stress with its structure, biosynthesis, metabolic engineering and functional aspects. Curr Plant Biol. 2021;26(3):100203. doi:10.1016/j.cpb.2021.100203. [Google Scholar] [CrossRef]
79. Black HS, Boehm F, Edge R, Truscott TG. The benefits and risks of certain dietary carotenoids that exhibit both anti-and pro-oxidative mechanisms—a comprehensive review. Antioxidants. 2020;9(3):264. doi:10.3390/antiox9030264. [Google Scholar] [PubMed] [CrossRef]
80. Bouzouina M, Kouadria R, Lotmani B. Fungal endophytes alleviate salt stress in wheat in terms of growth, ion homeostasis and osmoregulation. J Appl Microbiol. 2021;130(3):913–25. doi:10.1111/jam.14804. [Google Scholar] [PubMed] [CrossRef]
81. Farouk S, Al-Ghamdi AAM. Sodium nitroprusside application enhances drought tolerance in marjoram herb by promoting chlorophyll biosynthesis and enhancing osmotic adjustment capacity. Arab J Geosci. 2021;14(6):1–13. doi:10.1007/s12517-021-06846-5. [Google Scholar] [CrossRef]
82. da Oliveira APS, Melo YL, de Alencar RS, Viégas PRA, Dias GF, de Ferraz RLS, et al. Osmoregulatory and antioxidants modulation by salicylic acid and methionine in cowpea plants under the water restriction. Plants. 2023;12(6):1341. doi:10.3390/plants12061341. [Google Scholar] [PubMed] [CrossRef]
83. Alghamdi SA, Alharby HF, Bamagoos AA, naz Zaki SS, Abu El-Hassan AMA, Desoky ESM, et al. Rebalancing nutrients, reinforcing antioxidant and osmoregulatory capacity, and improving yield quality in drought-stressed Phaseolus vulgaris by foliar application of a bee-honey solution. Plants. 2023;12(1):1–25. doi:10.3390/plants12010063. [Google Scholar] [PubMed] [CrossRef]
84. Schütz L, Gattinger A, Meier M, Müller A, Boller T, Mäder P, et al. Improving crop yield and nutrient use efficiency via biofertilization—a global meta-analysis. Front Plant Sci. 2018;8:2204. doi:10.3389/fpls.2017.02204. [Google Scholar] [PubMed] [CrossRef]
85. Basu S, Rabara RC, Negi S. AMF: the future prospect for sustainable agriculture. Physiol Mol Plant Pathol. 2018;102(5):36–45. doi:10.1016/j.pmpp.2017.11.007. [Google Scholar] [CrossRef]
86. Salma T, Mohamed A, Abderrahim B, Raja BL, Wissal B, Hela BA, et al. Combined use of mycorrhizae and green compost for reducing the deleterious effects of salt stress in two genotypes of quinoa (Chenopodium quinoa). J Soil Sci Plant Nutr. 2023;23(1):1254–71. doi:10.1007/s42729-022-01118-x. [Google Scholar] [CrossRef]
87. Michaletti A, Naghavi MR, Toorchi M, Zolla L, Rinalducci S. Metabolomics and proteomics reveal drought-stress responses of leaf tissues from spring-wheat. Sci Rep. 2018;8(1):1–18. doi:10.1038/s41598-018-24012-y. [Google Scholar] [PubMed] [CrossRef]
88. Wang X, Liu B, Ma J, Zhang Y, Hu T, Zhang H, et al. Soil aluminum oxides determine biological nitrogen fixation and diazotrophic communities across major types of paddy soils in China. Soil Biol Biochem. 2019;131:81–9. doi:10.1016/J.SOILBIO.2018.12.028. [Google Scholar] [CrossRef]
89. Ozturk M, Turkyilmaz Unal B, García-Caparrós P, Khursheed A, Gul A, Hasanuzzaman M. Osmoregulation and its actions during the drought stress in plants. Physiol Plant. 2021;172(2):1321–35. doi:10.1111/ppl.13297. [Google Scholar] [PubMed] [CrossRef]
90. Zandalinas SI, Balfagón D, Arbona V, Gómez-Cadenas A. Modulation of antioxidant defense system is associated with combined drought and heat stress tolerance in citrus. Front Plant Sci. 2017;8:1–10. doi:10.3389/fpls.2017.00953. [Google Scholar] [PubMed] [CrossRef]
91. Boutasknit A, Baslam M, Anli M, Ait-El-Mokhtar M, Ben-Laouane R, Ait-Rahou Y, et al. Impact of arbuscular mycorrhizal fungi and compost on the growth, water status, and photosynthesis of carob (Ceratonia siliqua) under drought stress and recovery. Plant Biosyst. 2021;156(4):994–1010. doi:10.1080/11263504.2021.1985006. [Google Scholar] [CrossRef]
92. Sheteiwy MS, Ali DFI, Xiong YC, Brestic M, Skalicky M, Hamoud YA, et al. Physiological and biochemical responses of soybean plants inoculated with arbuscular mycorrhizal fungi and Bradyrhizobium under drought stress. BMC Plant Biol. 2021;21(1):1–21. doi:10.1186/s12870-021-02949-z. [Google Scholar] [PubMed] [CrossRef]
93. Elsherpiny M. Role of compost, biochar and sugar alcohols in raising the maize tolerance to water deficit conditions. Egypt J Soil Sci. 2023;63(1):67–81. doi:10.21608/ejss.2022.178322.1556. [Google Scholar] [CrossRef]
94. Liu CY, Wang YJ, Wu QS, Yang TY, Kuča K. Arbuscular mycorrhizal fungi improve the antioxidant capacity of tea (Camellia sinensis) seedlings under drought stress. Not Bot Horti Agrobot Cluj-Napoca. 2020;48:1993–2005. doi:10.15835/48412066. [Google Scholar] [CrossRef]
95. Boutasknit A, Ait-el-mokhtar M, Fassih B, Ben-laouane R. Effect of arbuscular mycorrhizal fungi and rock phosphate on growth, physiology, and biochemistry of carob under water stress and after rehydration in vermicompost-amended soil. Metabolites. 2024;14(4):202. doi:10.3390/metabo14040202. [Google Scholar] [PubMed] [CrossRef]
96. Dien DC, Mochizuki T, Yamakawa T. Effect of various drought stresses and subsequent recovery on proline, total soluble sugar and starch metabolisms in Rice (Oryza sativa L.) varieties. Plant Prod Sci. 2019;22(4):530–45. doi:10.1080/1343943X.2019.1647787. [Google Scholar] [CrossRef]
97. Rahimi A, Mohammadi MM, Siavash Moghaddam S, Heydarzadeh S, Gitari H. Effects of stress modifier biostimulants on vegetative growth, nutrients, and antioxidants contents of garden thyme (Thymus vulgaris L.) under water deficit conditions. J Plant Growth Regul. 2022;41(5):2059–72. doi:10.1007/s00344-022-10604-6. [Google Scholar] [CrossRef]
98. Tao H, Sun H, Wang Y, Wang X, Guo Y. Effects of water stress on quality and sugar metabolism in ‘Gala’ apple fruit. Hortic Plant J. 2023;9(1):60–72. doi:10.1016/j.hpj.2022.03.008. [Google Scholar] [CrossRef]
99. Ye Q, Wang H, Li H. Arbuscular mycorrhizal fungi improve growth, photosynthetic activity, and chlorophyll fluorescence of Vitis vinifera L. cv. Ecolly under drought stress. Agronomy. 2022;12:1563. doi:10.3390/agronomy12071563. [Google Scholar] [CrossRef]
100. Hu Y, Xie W, Chen B. Arbuscular mycorrhiza improved drought tolerance of maize seedlings by altering photosystem II efficiency and the levels of key metabolites. Chem Biol Technol Agric. 2020;7(1):1–14. doi:10.1186/s40538-020-00186-4. [Google Scholar] [CrossRef]
101. Abdi N, van Biljon A, Steyn C, Labuschagne MT. Bread wheat (Triticum aestivum) responses to arbuscular mycorrhizae inoculation under drought stress conditions. Plants. 2021;10(9):1756. doi:10.3390/plants10091756. [Google Scholar] [PubMed] [CrossRef]
102. Wu QS. Arbuscular mycorrhizas and stress tolerance of plants. Singapore: Springer Singapore; 2017. doi:10.1007/978-981-10-4115-0 [Google Scholar] [CrossRef]
103. Wu J, Zhao Y, Qi H, Zhao X, Yang T, Du Y, et al. Identifying the key factors that affect the formation of humic substance during different materials composting. Bioresour Technol. 2017;244(283):1193–6. doi:10.1016/J.BIORTECH.2017.08.100. [Google Scholar] [PubMed] [CrossRef]
104. Ben-Laouane R, Ait-El-Mokhtar M, Anli M, Boutasknit A, Ait Rahou Y, Raklami A, et al. Green compost combined with mycorrhizae and rhizobia: a strategy for improving alfalfa growth and yield under field conditions. Gesunde Pflanz. 2021;73(2):193–207. doi:10.1007/s10343-020-00537-z. [Google Scholar] [CrossRef]
105. Zagoskina NV, Zubova MY, Nechaeva TL, Kazantseva VV, Goncharuk EA, Katanskaya VM, et al. Polyphenols in plants: structure, biosynthesis, abiotic stress regulation, and practical applications (review). Int J Mol Sci. 2023;24(18):13874. doi:10.3390/ijms241813874. [Google Scholar] [PubMed] [CrossRef]
106. Rouphael Y, Colla G. Editorial: biostimulants in agriculture. Front Plant Sci. 2020;11:40. doi:10.3389/fpls.2020.00040. [Google Scholar] [PubMed] [CrossRef]
107. Alizadeh S, Fallahi Gharagoz S, Pourakbar L, Siavash Moghaddam S, Jamalomidi M. Arbuscular mycorrhizal fungi alleviate salinity stress and alter phenolic compounds of Moldavian balm. Rhizosphere. 2021;19(2):100417. doi:10.1016/j.rhisph.2021.100417. [Google Scholar] [CrossRef]
108. Celikcan F, Kocak MZ, Kulak M. Vermicompost applications on growth, nutrition uptake and secondary metabolites of Ocimum basilicum L. under water stress: a comprehensive analysis. Ind Crops Prod. 2021;171(1):113973. doi:10.1016/j.indcrop.2021.113973. [Google Scholar] [CrossRef]
109. Mohammadi E, Fattahi M, Barin M, Ashrafi-Saeidlou S. Arbuscular mycorrhiza and vermicompost alleviate drought stress and enhance yield, total flavonoid, rutin content, and antioxidant activity of buckwheat (Fagopyrum esculentum Moench). South African J Bot. 2022;148:588–600. doi:10.1016/j.sajb.2022.05.020. [Google Scholar] [CrossRef]
110. Mahdi TA, Hossein AF. Changes in Kaempferol content of Chicory (Cichorium intybus L.) under water deficit stresses and planting densities. J Med Plants Res. 2014;8(1):30–5. doi:10.5897/jmpr10.732. [Google Scholar] [PubMed] [CrossRef]
111. Laoué J, Fernandez C, Ormeño E. Plant flavonoids in Mediterranean species: a focus on flavonols as protective metabolites under climate stress. Plants. 2022;11(2):172. doi:10.3390/plants11020172. [Google Scholar] [PubMed] [CrossRef]
112. Agati G, Azzarello E, Pollastri S, Tattini M. Flavonoids as antioxidants in plants: location and functional significance. Plant Sci. 2012;196:67–76. doi:10.1016/j.plantsci.2012.07.014. [Google Scholar] [PubMed] [CrossRef]
113. Singh P, Arif Y, Bajguz A, Hayat S. The role of quercetin in plants. Plant Physio and Biochem. 2021;166:10–9. doi:10.1016/j.plaphy.2021.05.023. [Google Scholar] [PubMed] [CrossRef]
114. Alizadeh Yeloojeh K, Saeidi G, Sabzalian MR. Drought stress improves the composition of secondary metabolites in safflower flower at the expense of reduction in seed yield and oil content. Ind Crops Prod. 2020;154:112496. doi:10.1016/j.indcrop.2020.112496. [Google Scholar] [CrossRef]
115. Sarker U, Oba S. Polyphenol and flavonoid profiles and radical scavenging activity in leafy vegetable Amaranthus gangeticus. BMC Plant Biol. 2020;20(1):1–12. doi:10.1186/s12870-020-02700-0. [Google Scholar] [PubMed] [CrossRef]
116. Gharibi S, Sayed Tabatabaei BE, Saeidi G, Talebi M, Matkowski A. The effect of drought stress on polyphenolic compounds and expression of flavonoid biosynthesis related genes in Achillea pachycephala Rech.f. Phytochemistr. 2019;162(1):90–8. doi:10.1016/J.PHYTOCHEM.2019.03.004. [Google Scholar] [PubMed] [CrossRef]
117. Karimi G, Pourakbar L, Moghaddam SS, Popović-Djordjević J. Integrated effects of bacteria and fungi biofertilizers on morphological traits, antioxidants indices, and polyphenol compounds of quinoa (Chenopodium quinoa Willd.) under salinity condition. Res Sq. 2020:1–32. doi:10.21203/rs.3.rs-123080/v1. [Google Scholar] [CrossRef]
118. Cataldo E, Fucile M, Manzi D, Peruzzi E, Mattii GB. Effects of zeowine and compost on leaf functionality and berry composition in Sangiovese grapevines. J Agric Sci. 2023;161(3):412–27. doi:10.1017/S002185962300028X. [Google Scholar] [CrossRef]
Cite This Article
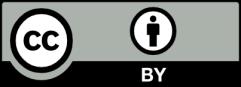
This work is licensed under a Creative Commons Attribution 4.0 International License , which permits unrestricted use, distribution, and reproduction in any medium, provided the original work is properly cited.