Open Access
ARTICLE
Comparative Effectiveness of Rhizophagus irregularis and Compost vs. Conventional Chemical Fertilizers in Managing Verticillium dahliae: A Sustainable Agriculture Approach
1 Centre d’Agrobiotechnologie et Bioingénierie, Unité de Recherche labellisée CNRST (Centre AgroBiotech-URL-CNRST-05), Université Cadi Ayyad, Marrakech, 40000, Morocco
2 Laboratory of Agro-Food, Biotechnologies and Valorization of Plant Bioresources (AGROBIOVAL), Department of Biology, Faculty of Science Semlalia, Cadi Ayyad University (UCA), Marrakech, 40000, Morocco
3 Plant, Animal Productions and Agro-Industry Laboratory, Department of Biology, Faculty of Sciences, Ibn Tofail University, B.P. 133, Kenitra, 14000, Morocco
4 Higher Institute of Nursing Professions and Health Techniques, Ministry of Health, Ouarzazate, 45000, Morocco
5 Unité de Recherche Résistance Induite et Bioprotection des Plantes, Université de Reims Champagne Ardenne, USC 1488, Reims, 51100, France
6 Department of Plant Protection, Phytopathology Unit, Ecole Nationale d’Agriculture de Meknès, Meknès, 50001, Morocco
7 African Sustainable Agriculture Research Institute (ASARI), Mohammed VI Polytechnic University (UM6P), Laayoune, 70000, Morocco
* Corresponding Authors: Rachid Lahlali. Email: ; Abdelilah Meddich. Email:
Phyton-International Journal of Experimental Botany 2024, 93(9), 2415-2438. https://doi.org/10.32604/phyton.2024.055050
Received 14 June 2024; Accepted 27 August 2024; Issue published 30 September 2024
Abstract
This study assesses the effectiveness of using arbuscular mycorrhizal fungi (M) and compost applied alone or in combination (vs. conventional chemical fertilizer application (NPK)) on tomato resistance to vascular wilt caused by Verticillium dahliae. In this study, ten treatments were applied: (1) Control (2) M: Rhizophagus irregularis (3) C: Compost (4) MC: The combination of M and compost (5) NPK: conventional chemical fertilizer (6) V: plants infected with V. dahliae (7) MV: plants infected with a conidial suspension of V. dahliae and M, (8) CV: The combination of compost and V. dahliae, (9) MCV: The combination of M, compost and V. dahliae, (10) VNPK: V. dahliae and NPK. The combination of biostimulants reduced disease severity and incidence, as well as the leaf alteration index compared to control plants (V). However, the area under the disease progress curve (AUDPC) and final mean severity (FMS) were reduced by 37% and 46% respectively by the application of the combination of M, compost and V. dahliae (MCV) compared to the V control. Additionally, the MCV treatment showed the greatest increment in protein content (152.6%), in TSS content (54.6%), and CV increased proline content by 46.6%. Furthermore, MCV also protected the photosynthetic apparatus from pathogen-induced oxidative stress and reduced the accumulation of malondialdehyde (MDA) and hydrogen peroxide (H2O2) by increasing the activity of antioxidant enzymes, such as polyphenol oxidase (PPO) (215.03%), ascorbate peroxidase (APX) (74.73%) and peroxidase activity (POX) (101.91%). MC also enhanced superoxide dismutase (SOD) activities by 166.19% compared to their controls (V). M alone or in combination with compost, remains a favourable interaction for suppressing plant diseases and improving antioxidant defense systems.Keywords
Supplementary Material
Supplementary Material FilePlant diseases are the most common reasons of reduced plant yields and quality, resulting in significant economic losses, notably in agriculture [1]. Verticillium dahliae is a highly pathogenic fungus that causes damage to more than 200 species of plants, causing significant losses in important food crops [2]. It induces Verticillium wilt, one of the most prevalent diseases affecting annual agricultural and horticultural plants, including commercially important solanaceous species like tomatoes [3]. Tomatoes (Solanum lycopersicum L.) are a widely cultivated horticultural crop enjoyed worldwide for their culinary versatility. They hold significant commercial value [4] and are rich in bioactive substances such as vitamins, phenolic compounds, and carotenoids [5]. Additionally, they contain metabolites like sucrose, hexoses, citrate, and malate, which contribute positively to human health [6]. Tomato agriculture faces various challenges globally, including diseases like Verticillium wilt. Verticillium dahliae is a soil-borne fungus that remains in the soil indefinitely and spreads rapidly, leading to blockage of vascular tissue. Symptoms include yellowing, vessel-shaped lesions, stunted growth, severe defoliation, and eventually plant mortality [7,8]. Effective management of this vascular pathogen involves understanding plant responses, starting from pathogen detection and signal transduction, to the activation of defense-related genes [9]. To combat tomato infections, various tactics and treatments have been employed, including chemical management with fungicides such as propineb and copper oxychloride [10]. However, it is widely recognised that the widespread consumption of chemical substances in agriculture in agriculture is reducing soil fertility and threatening food safety and environmental health [11,12]. Understanding pathogen virulence, host resistance, and plant-pathogen interactions could potentially aid in developing integrated disease control strategies. Recent research has provided new insights into these processes and emphasized the importance of implementing ecologically sustainable management options. In this respect, biostimulants offer a viable alternative to chemical pesticides by providing essential nutrients, promoting environmental sustainability and increasing agricultural sustainability and food safety. Over the past few decades, microbial fertilizers like M have emerged as a novel approach to the promotion of the sustainability of agriculture. M form symbiotic relationships with plants, facilitating the exchange of signaling molecules that enhance plant resistance to infections and improve tolerance to various stressors [13,14]. Mycorrhizal symbiosis is essential for managing plant diseases [15]. To explain how M confer bioprotection to crops against soil-borne diseases, several mechanisms have been suggested. These include enhancing plant nutrition, compensating for damage, modifying root anatomy and longevity, competing with pathogens for root colonization sites and host resources, activating plant defenses, and altering populations of microorganisms in the rhizosphere [16]. Mycorrhizal fungi rescue plants from stress-induced reactive oxygen species (ROS) by improving their defense system and enhancing the functioning of antioxidant enzymes [17]. They act as biostimulants, aiding plants in defending against wilt diseases [18]. Mycorrhizal fungi stimulate plant development through a variety of methods, including improved mineral supplying and water consumption by host plants [19,20]. These fungi are ecologically beneficial organisms that form a symbiotic association with plant roots, in particular improving phosphorus uptake and maintaining phytohormone balance [21]. Another promising strategy involves using compost derived from green waste, which represents an environmentally favourable method for reducing V. dahliae [22]. Compost has been recognized for its ability to enhance soil structure and suppress soil-borne diseases due to its diverse microbial communities [23,24]. Compost’s suppressive power is demonstrated by processes such as hyperparasitism, antibiosis, and trophic and spatial competition, as well as its potential to stimulate plant development and boost quality and resilience [25]. Compost inoculated with biostimulants such as Bacillus subtilis or Trichoderma hamatum has been shown to have a good effect on disease suppression in several studies, owing to its multifarious microbial consortium [26]. Compost can be added to control soil pathogens V. dahliae, Fusarium oxysporum, Rhizoctonia solani, and Botrytis cinerea through several mechanisms. These include the production of fungitoxic chemicals, direct parasitism, nutritional competition, and the promotion of a suppressive microbiome to increase disease control consistency [23–27]. According to Antón-Domínguez et al. [27], organic amendments hindered the growth of V. dahliae mycelium. Specifically, these amendments were shown to enhance the activity of antagonistic microorganisms or directly inhibit the mycelial growth through changes in nutrient availability or pH levels. This suggests that organic amendments could potentially serve as a sustainable method to manage V. dahliae in agricultural soils, offering a natural alternative to synthetic fungicides. Further research is essential to fully comprehend the chemical processes behind these inhibitory effects and optimize their usage in agricultural practices for disease prevention.
However, notwithstanding these advances, a significant knowledge gap remains regarding the efficacy of M and compost combinations vs. conventional chemical fertilizers in controlling V. dahliae, while promoting sustainable agricultural practices. Comparative studies evaluating the efficacy of conventional chemical fertilizers vs. biostimulants in enhancing tomato resistance to V. dahliae remain limited. These biostimulants, whether applied individually or in combination, are considered a strategy in agriculture aimed at producing high-yield crops while also safeguarding plants from disease [25]. Despite these advancements, few studies have directly compared the efficacy of conventional chemical fertilizers with biostimulants and compost in enhancing plant resistance against specific pathogens such as V. dahliae. The present work is intended to address this challenge by evaluating the suppressive properties of a tripartite combination involving V. dahliae, green compost, and the mycorrhizal fungus Rhizophagus irregularis, compared to conventional chemical fertilizers. Specifically, we investigate their influence on osmolyte accumulation and antioxidant defense systems in tomatoes, shedding light on their potential for integrated disease management and sustainable agriculture practices. By addressing these research gaps, this work seeks to make a contribution to the development of sustainable agricultural systems that enhance crop productivity, while also promoting environmental health, and limit the environmental damage caused by current agricultural practices. Understanding the synergistic effects of microbial fertilizers and compost could pave the way for integrated disease management strategies that foster resilient agricultural systems. Future research inspired by our study could explore several promising directions to advance sustainable agriculture. Firstly, deeper investigations into how Rhizophagus irregularis and compost interact with Verticillium dahliae could uncover specific biochemical pathways and microbial dynamics. Understanding these mechanisms could optimize their application strategies in agriculture. Secondly, more research needed to assess the long-term effects of organic alternatives on the health of the soil, including microbial diversity, nutrient retention, and carbon sequestration over multiple growing seasons.
2.1 Preparation of Biostimulants
A pure strain of Rhizophagus irregularis (isolate DAOM 197198) obtained from the Plant Biotechnology Institute of Montreal, Canada, served as the mycorrhizal fungus (M) used in the experiment. The host plant was Zea mays L., whose propagules had been enhanced by co-cultivation. To form the fungal inoculum, maize roots were chopped into 1 mm sections after colonisation with hyphae, vesicles and spores by Rhizophagus irregularis. To infect the root systems of the tomato plants, 30 g of this inoculum (including roots and spore-containing substrate) was applied during planting. This inoculum contains 748 spores/100g dry soil. Non-mycorrhizal plants received the same amount of filtered inoculum and uninoculated maize roots were added to maintain organic matter levels in the pots to reintroduce additional soil microorganisms. Mycorrhizal inoculum was filtered through Whatman paper filters containing 20 mL distilled water.
As mentioned by Meddich et al. [28], Green waste (quack grass) was the compost used in this experiment. The following physico-chemical features of the compost were present: Potassium 0.5%, Nitrogen 1.5%, Phosphorus 700 ppm, and Organic carbon 20%.
2.2 Plant Material and Experimental Design
The ‘Campbell 33’ tomato seeds were acquired from the National Institute for Agricultural Research located in Kenitra, Morocco. Tomato seeds (Lycopersicon esculentum Mill. cv. Campbell 33) have been disinfected through immersion for 10 min in a sodium hypochlorite solution (10%). The seeds were then rinsed thoroughly with distilled water five times. They were then transferred to glass Petri dishes and cultured at 28°C for a period of five days. After emergence they were placed in plastic dishes with sterile peat. The seedlings were transferred after 15 days, to plastic pots containing either 5 kg of sterilised soil heated for 3 h at 180°C or a 5% compost/sterilised soil mixture. The physical and chemical characteristics of the soil were as follows: total nitrogen: 9 mg kg−1; available phosphorus: 11 mg kg−1; organic matter: 1%; total organic carbon (TOC): 0.6%; potassium: 568 mg g−1 and calcium: 2356 g kg−1; electrical conductivity: 0.14 mS cm−1; pH: 8.6. Recommended doses of conventional chemical fertiliser (NPK) were applied as follows: 127 kg P2O5/ha as superphosphate + 332 kg K2O/ha as potassium sulfate +134 kg N/ha in the form of ammonium nitrate [29].
The experimental design consists of ten well-randomised block treatments: (1) Control: Seedling uninoculated and unamended (2) M: seedlings inoculated with 30 g of M inoculum (containing hyphae, vesicles and spores with high M infection frequency (95%) and intensity (65%)), (3) C: Compost (4) MC: The combination of M and compost (5) NPK: conventional chemical fertilizer (6) V: plants infected with a conidial suspension of V. dahliae (7) MV: plants infected with a conidial suspension of V. dahliae and M, (8) CV: The combination of compost and suspension of V. dahliae, (9) MCV: The combination of M, compost and suspension of V. dahliae, (10) VNPK: plants infected with a combination of suspension of V. dahliae and conventional chemical fertilizer. A semi-controlled greenhouse at the Faculty of Science Semlalia, Cadi Ayyad University, Marrakech, Morocco, was used to cultivate 300 tomato plants, with thirty duplicates per treatment. The greenhouse had an average temperature of 25.5°C, 16/8 day/night cycle, a light intensity of 410 μm−2 s−1, and a relative humidity of 68.5%. All treatments received regular watering, with pots kept at 75% full. After two months of mycorrhization, 150 plants were infected with a conidial solution of V. dahliae at 106 conidia/mL. To promote infection with the OMV5 isolate, the roots of these plants were carefully injured 6 cm below the stem apex with a pointed stick and 10 mL of pathogen inoculum was injected into each wound using a test tube. The remaining plants received the same treatment with 10 mL of sterile distilled water. All plants were well watered, with regular irrigation maintaining 75% of field capacity (FC).
2.3 Mycorrhization Assessments
To determine mycorrhizal colonization in roots, fresh tomato root fragments measuring 1 cm were cleansed with 10% KOH at 90°C for 2 h [30]. The segments were acidified with 5% lactic acid for 20 min, then stained with 0.05% (w/v) trypan blue for 30 min at 90°C and finally examined under a microscope.
15 randomly chosen root fragments, each measuring 1 cm in length, were assessed three times per treatment using a glass slide to measure the frequency (F%) and intensity (I%) of mycorrhization. These equations were used to determine these parameters [31].
where n is the total number of pieces, with an index of 0, 1, 2, 3, 4, or 5, and an infection rate of: 100 > n5 > 90; 90 > n4 > 50; 50 > n3 > 10; 10 > n2 > 1; 1 > n1 > 0.
“n5” signifies the number of roots with an infection level of 5 (90%–100% infection rate), while “n4” refers to the number of roots with an infection level of 4 (50%–90% infection rate), “n3” is the number of roots with an infection level of 3 (10%–50% infection rate), “n2” means the number of roots with an infection level of 2 (1%–10% infection rate) and “n1” signifies the number of roots with an infection level of 1 (0%–1% infection rate).
2.4 Evolution of Disease Symptoms
Disease symptoms were observed and assessed for three months after inoculation with the OMV5 isolate. The severity of the disease was measured on a scale of 0 to 5 according to the following Formula (1) [32]. Disease incidence, the proportion of infected plants in the total number of plants assessed, was calculated using Formula (2) [33], and the leaf alteration index was derived using [34] (Formula (3)).
where A: healthy plant or no symptoms; B: beginning dieback, no defoliation; C: moderate dieback, moderate wilting; D: severe dieback, severe wilting and E: dead plant. Notes on leaf appearance: 0: leaves appearing healthy; 1: cotyledons: wilting or yellowing; 2: cotyledons: dropping; 3: true leaves: wilting or yellowing; 4: true leaves: necrosis; 5: true leaves: abscission.
where i: leaves appearance notes 0–5, Xi: number of leaves with the note i, Nt L: total number of leaves.
The area under the disease progression curve (AUDPC), as described by Campbell et al. [35], was used to characterise disease progression. The standardised AUDPC was estimated using the following Formula (4):
where n: total number of observations; Si: final mean severity (FMS); 4: maximum disease rating
Following the experiment, V. dahliae was re-isolated from the roots and tomato epicotyls. Samples were washed with ordinary water, wiped with sterile filter paper, sectioned into pieces, and then disinfected with 70% alcohol for one minute. Sections were placed into PDA medium and cultured for one week at 25°C in darkness.
2.5 Assessment of Physiological Parameters and Photosynthetic Pigment Contents
Photosynthetic pigments, stomatal conductance (gs), and chlorophyll fluorescence (Fv/Fm) were assessed to evaluate the effects of compost, M, or V. dahliae on physiological parameters.
A handheld porometer (Leaf Porometer LP 1989, Decagon Devices Inc., Washington, DC, USA) was utilized to measure the stomatal conductance (gs). The device was calibrated with the provided calibration plate before each usage, and it was determined between 10:00 and 11:30 a.m. The sheet’s underside was used for the measurements. The units of the gs were mmol H2O m–2 s–1.
Using a portable fluorometer (OS-30p + OPTI-SCIENCES), Fv/Fm of tomato plant leaves that were still intact was measured. The youngest, entirely dilated leaves were used for measurements on the underside. The measured parameters are the initial (F0), maximum (Fm), and quantum efficiency (Fv/Fm), where Fv is the variable fluorescence. Each parameter was subjected to five measurements per treatment, which were carried out on sunny days between 10:30 and 11:30 a.m.
Concentrations of photosynthetic pigments (chlorophyll a (Chl a), chlorophyll b (Chl b), total chlorophyll and carotenoids) were quantified spectrophotometrically in 80% acetone extracts as reported by Arnon [36]. After 2 h in the dark, the collected material was centrifuged at 10,000 g for 10 min. Using a UV-vis spectrophotometer, the optical density of the supernatant was determined at 480, 645, and 663 nm (UV-3100PC spectrophotometer). As a control, a blank containing 80% acetone was employed.
Photosynthetic pigment content was calculated using the following formulas:
where OD = optical density; V = final volume of the extract and FW = fresh weight.
2.6 Determination of Lipidic Peroxidation
The method of Velikova et al. [37] was used to determine the hydrogen peroxide (H2O2) content of the leaves. To measure H2O2 concentration, 0.1 g of frozen leaf powder was mixed with 2 mL of 10% (w/v) TCA and centrifuged at 15,000× g. From the supernatant, 0.5 mL was combined with 0.5 mL of 10 mM potassium phosphate buffer (pH 7) and 1 mL of 1 M potassium iodide. After incubation for three minutes, the absorbance of the mixture was read at 390 nm and compared with a standard H2O2 curve.
The malondialdehyde (MDA) content was determined according to the method described by Dhindsa et al. [38]. A sample of 100 mg of fresh material was homogenized in 10 mL of 0.1% trichloroacetic acid (TCA) and subsequently centrifuged at 18,000
2.7 Determination of Osmolytes and Antioxidant Enzymes Concentrations in Plants
To measure total soluble sugars (TSS), A 100 mg sample of fresh tissue was homogenized in 4 mL of 80% ethanol. Subsequently, 0.25 mL of phenol and 1.25 mL of concentrated sulfuric acid were added to the resulting supernatant. Following a 10-min reaction, the absorbance at 485 nm was measured to determine the total soluble sugar (TSS) content, as per the specified procedure DuBois et al. [39].
To quantify total soluble protein in leaves, A quantity of 0.25 g of frozen material was homogenized in 0.5 mL of 0.02 M potassium phosphate buffer at pH 7.6. The mixture was then centrifuged at 10,000× g for 10 min at 4°C. The resulting supernatant was collected for the measurement of protein content using the Bradford method [40], Bovine serum albumin was used as a reference.
Free proline concentration was determined using the method of Carillo et al. [41], involving homogenization of plant material in 40% (4 mL) ethanol, reaction with a ninhydrin-containing mixture (20% ethanol, 60% acetic acid and 1% ninhydrin). At 520 nm, the optical density (OD) was calculated.
A 0.1 g sample of frozen leaves was added to a chilled mortar containing 4 mL of 1 M phosphate buffer (pH 7) with 5% polyvinylpolypyrrolidone. The mixture was homogenized and then centrifuged at 18,000× g for 15 min at 4°C. The resulting supernatant was used to assess the activity of antioxidant enzymes [42].
Peroxidase (POX) activity was measured as described by Hori et al. [43]. POX activity was assessed by its ability to convert guaiacol to tetraguaiacol (ε = 26.6 mM−1 cm−1) at 470 nm. The reaction mixture included 2 mL of 0.1 M phosphate buffer (pH 7.0), 1 mL of 20 mM guaiacol, 0.3% H2O2 (10 mM), and 0.1 mL of enzyme extract. POX activity was recorded at 470 nm, with one unit defined as a 0.01 unit min−1 change in absorbance.
Polyphenol oxidase (PPO) activity was estimated by the method described by Hori et al. [43]. The test solution consisted of 0.1 M phosphate buffer and 20 mM catechol at pH 7. PPO activity was measured in enzyme units per mg of protein, the reaction being initiated by the addition of 100 µL of the enzyme extract. One unit of PPO activity was defined as the amount of enzyme that caused a 0.001 min−1 increase in absorbance at 420 nm.
Ascorbate peroxidase (APX) was assayed using the method described by Amako et al. [44]. 2.9 mL of reaction mixture containing 50 mM of sodium phosphate buffer (pH 7.0), 0.2 mM EDTA, 0.5 mM ascorbic acid, and 100 µM H2O2 was added to the enzyme extract (100 µL). For three minutes, the decrease in absorbance was recorded at 290 nm. µmol mg−1 protein oxidized ascorbate per min was used to define an enzyme unit.
Superoxide dismutase (SOD) activity was measured by its capacity to prevent the reduction of nitro blue tetrazolium (NBT), using the method defined by Beyer et al. [45]. Briefly, 0.5 g of frozen leaf powder subsamples were homogenised in 500 μL of 0.15 M Tris-HCl buffer (pH 7.5) with 50 mg polyvinylpyrrolidone (PVP). The mixture was then centrifuged twice at 14,000× g for 10 min at 4°C. DO activity was measured in units per mg of protein, where one unit is defined as the amount of enzyme that inhibits 50% of NBT photoreduction at 560 nm.
COSTAT software (version 6.3) was used for statistical analysis, including analysis of variance and post-hoc Tukey’s honest significant difference test (p ≤ 0.05). SPSS was used to examine the data (version 23, IBM Analytics). To investigate the effects of Verticillium dahlia and to assess the significance of variations in plant responses to various treatments, multifactorial analysis of variance (MANOVA) was conducted. Significant differences among the tested factors (M; Compost; C; NPK; and Verticillium dahliae; D) and their interactions were determined with 95% confidence using Tukey’s honest significant difference test (p ≤ 0.05) via SPSS 23.0 software (MANOVA), IBM, Armonk, NY, USA. The heat map was generated using GraphPad® Prism v 8.0.1 (GraphPad Software, San Diego, CA, USA).
3.1 Effect of Biostimulants on Development of Disease Symptoms
The evaluation of Verticillium wilt symptoms was performed through disease severity and it was found that control plants did not show any symptoms (yellowing, chlorosis, necrosis, wilting, or dieback), they grow normally, whereas tomato plants inoculated with V. dahliae presented intensive yellowing and defoliation after one-month of inoculation (Fig. 1). Symptoms of Verticillium wilt of tomato plants become intensive and progressive showing chlorosis and wilting in pathogen-inoculated plants, whereas in mycorrhizal-infected plants, we observed only yellowing and some chlorosis in basal leaves after 2 months of V. dahliae inoculation, which progress over time. Moreover, the disease incidence showed an extensive wilting in inoculated tomatoes by V. dahliae (Table 1; Fig. 1b). Inoculation with M and compost, either individually or in combination, reduced disease severity by decreasing symptoms development over time. However, after 3 months of V. dahliae infection, the leaf alteration index (LAI) in MCV showed the highest percent of reduction (−50.1%) compared to untreated control, followed by MV (−45.8%), VNPK (−32.9%), CV (−30.5%), respectively. The effect of Rhizophagus irregularis on symptoms reduction was observed by estimating AUDPC and FMS. Maximum values were shown in tomato plants infected by the pathogen alone (V) and (VNPK) with 25.81% and 22.32% respectively for AUDPC, 1.25 and 1.08 for FMS compared to plants inoculated by M and amended by compost alone or combined (Table 1). In this respect, (AUDPC) and FMS levels were substantially higher in plants affected with pathogene. However, AUDPC and FMS decreased by 37% and 46%, respectively, in plants cultivated with compost and M in combination (MCV), in comparison to the V control. The interaction between C × D, M × D and NPK × D had a significant effect (p < 0.001) on leaf alteration index and AUDPC.
Figure 1: Evolution of disease severity (a) disease incidence (b) and leaf alteration index (c) of tomato plants inoculated or not with Rhizophagus irregularis for two months then infected with V. dahliae for three months or amended then infected by V. dahliae. V: Verticillium dahliae; MV: Rhizophagus irregularis + V. dahliae; CV: Compost + V. dahliae; MCV: Rhizophagus irregularis + Compost + V. dahliae
V. dahliae was re-isolated from tomato roots and epicotyls affected by pathogene (Table 1). Tomato roots had higher pathogen percentages than epicotyls. M and compost alone or in combination has greatly reduced V. dahliae re-isolation in the roots and epicotyls by 53% and 57%, respectively.
3.2 Root Mycorrhizal Colonization under V. dahliae Infection
The intensity and frequency of mycorrhization was significantly reduced by Verticillium dahliae infection in the roots of tomato seedlings (p ≤ 0.05) (Fig. 2). Our findings indicate that in the roots of the control, V, C, CV treatments and NPK treated tomato plants, no mycorrhizal structures were observed. The plants inoculated with the combination of M and Compost showed the higher root mycorrhization frequency with 40% in comparison to the plants that were treated with M alone in the absence of pathogen infection (Fig. 2a). Similarly, the plants inoculated by the combination of M and C exhibited higher mycorrhization intensity with 25% compared to the mycorrhized plants (M) (Fig. 2b). Verticillium dahliae reduced the parameters of mycorrhization, by 58% and 45% respectively for MV treatments. In addition, the presence of the pathogen significantly reduced the colonisation intensity, which was limited to 26% in the MCV treatments, in contrast to the MC combination. The interaction between M × D, and C × D had a significant effect (p < 0.001) on mycorrhization frequency and intensity.
Figure 2: Mycorrhizal frequency (a) and intensity (b) of tomatoes infected or uninfected with V. dahliae under different treatments. Control (no treatment); M: Rhizophagus irregularis; C: Compost; MC: Compost + Rhizophagus irregularis; NPK: Conventional chemical fertilizer; V: Verticillium dahliae; MV: Rhizophagus irregularis + V. dahliae; CV: Compost + V. dahliae; MCV: Rhizophagus irregularis + Compost + V. dahliae. The data reported are means ± SE. Means that share the same letters are not significantly different at p < 0.05 (Tukey’s HSD)
3.3 Biostimulants Overcome the Negative Effects of Verticillium dahliae on the Photosynthetic Efficiency
In the absence of V. dahliae, applying M and/or C and NPK resulted in a considerable improvement in the (Fv/Fm) and gs in tomato seedlings, the treatments of M, C, MC and NPK showed an improvement of Chlorophyll fluorescence by 8.7%, 11.7%, 5.6% and 3.6%, respectively, and enhance the stomatal conductance by 32.7%, 39.2%, 43.6% and 32.3% compared with untreated control plants (Fig. 3).
Figure 3: Chlorophyll fluorescence (Fv/Fm) (a) and stomatal conductance (gs) (b) of tomatoes infected or uninfected with V. dahliae under different treatments. Control (no treatment); M: Rhizophagus irregularis; C: Compost; MC: Compost + Rhizophagus irregularis; NPK: Conventional chemical fertilizer; V: Verticillium dahliae; MV: Rhizophagus irregularis + V. dahliae; CV: Compost + V. dahliae; MCV: Rhizophagus irregularis + Compost + V. dahliae. Data are mean ± SE of five biological replicates. Means that share the same letters are not significantly different at p < 0.05 (Tukey’s HSD)
In the presence of V. dahliae, the Efficiency of the Photosynthetic Machinery significantly decreased (p ≤ 0.05) (Fig. 3). Chlorophyll fluorescence (Fv/Fm) has been significantly reduced by V. dahliae. Biostimulants application improved significantly Fv/Fm in tomato seedlings. Non-inoculated plants (V) had the greatest reduction (−6.8%) compared to the plants treated with MV (−3.99%), CV (−3.72%), and MCV (−4.9%). In the presence of the pathogen, biostimulants resulted in higher gs values. Applying compost and M, either separately or together, significantly boosted stomatal conductance compared to the control. The MV, CV, MCV and NPK treatments showed a significant improvement of 50.7%, 44.6%, 42.8% and 28.4% respectively compared with untreated control plants (V) (Fig. 3). The interaction between M × C had a substantial influence had a significant effect for gs (p < 0.01) and for Fv/Fm (p < 0.001) (Table S1).
3.4 Biostimulants Mitigated the Adverse Effects of Verticillium dahliae on Photosynthetic Pigment Contents
In the absence of pathogen, chlorophyll a (Chl a), chlorophyll b (Chl b) and total chlorophyll contents (Chl T) (Table 2) were improved in M by 196.4%, 453.9%, and 300.8%, C by 239.2%, 416.2%, and 315.25%, MC by 234.6%, 322.7%, and 267.1% and NPK by 257.9%, 404.2%, and 321.1% respectively compared to the non-inoculated plants. However, the carotenoids were positively balanced by the application of M, C and MC (Table 2) contents by improvement 157.6%, 152.4% and 437.4%. In contrast, the photosynthetic pigment content was significantly affected by V. dahliae. Chlorophyll concentration decreased in the V treatment; however, the application of biostimulants attenuated the detrimental effects of V. dahliae. Chl a, Chl b and Chl T were enhanced in MV by 89.33%, 198.7%, and 121.8%, CV by 62.2%, 201.3%, and 103.4%, MC by 64.9%, 404.5% and 165.5%, in contrast NPK reduced by −59.4%, −2,1% and −42.4% respectively compared to the affected and non-inoculated plants (V). Comparatively to the affected and non-inoculated treatments (V), the application of M (M) and/or with compost positively compensated the adverse effects of V. dahliae and stimulated the concentrations of all photosynthetic pigments. The interaction between M × C and NPK × D, had a significant effect (p < 0.001) for Chl a, Chl b, Chl T and carotenoids (Table S1).
3.5 Biostimulants Compensated for the Harmful Effects of V. dahliae by Accumulating Osmolytes in Tomato Plants
The Table 3 shows that the application of biostimulants was able to maintain higher TSS content and significantly increased proline and protein contents for all the treatments compared to the controls. In the absence of V. dahliae, the highest percent of improvement in TSS content (98.5%), protein (69.1%) and proline (258.6%) were recorded in compost. Nevertheless, in the presence of V. dahliae, the protein concentration in NPK showed the greatest reduction (−47.1%) compared to NPK non affected, in contrast MV (−11.8%), CV (−14.1%), MCV (−6.1%) showed the lowest percent of reduction compared to M, C and MC, respectively. MCV showed the highest percent of improvement compared to the plants inoculated by pathogen (V). In addition, the highest level of TSS was recorded in the plants that were inoculated with MVC (54%) in comparison to the controls (V). Proline content was higher in CV treatment (46.6%) and MV (35.1%) than (diseased) control treatment (V). The interaction M × D and NPK × D had a significant effect (p < 0.001) for proline content, in addition the interaction M × C, M × D and C × D had a significant effect (p < 0.001) for protein content (Table S1).
3.6 Infection Biostimulants Reduce Oxidative Stress Levels against the Damaging Effects of V. dahliae
In order to assess the extent of the damage induced by the pathogen, the levels of malondialdehyde (MDA) and hydrogen peroxide (H2O2) were measured. In the absence of V. dahliae and compared to non-inoculated plants, applying single or combined biostimulants resulted in lower MDA and H2O2 concentration (Fig. 4). However, the application of M, C and MC separately reduced the MDA by −32.4%, −10.9% and −39.06% compared to the control in the absence of V. dahliae (Fig. 4a). Similarly, our findings demonstrated a maximal increase in H2O2 values in the NPK and non-inoculated treatments. The application of M, C and MC was showed significantly reduction in H2O2 content by −40.3%, −23.82% and −54.8% in comparison with control plants (Fig. 4b). However, the V and NPK treatments had the highest concentrations of MDA and H2O2 compared to the other treatments due to the presence of V. dahliae (Fig. 4). Moreover, in the presence of V. dahliae, the H2O2 content significantly decreased in MCV treatment by 42.1%, followed by MV (39.2%) and CV (32.5%) compared to plants inoculated solely with V. dahliae. Similarly, our findings demonstrated a maximal increase in H2O2 values in the NPK and V, while the lowest values for the MDA content were recorded in CV (−47.3%), MCV (−39.7%) and MV (−33.3%) treatments compared to V. The interaction M × C, C × D, NPK × D, Drought had a significant effect for MDA (p < 0.001) and H2O2 (p < 0.001) content (Table S1).
Figure 4: MDA: malondialdehyde content (a) and H2O2: hydrogen peroxide content (b) of tomatoes infected or uninfected with V. dahliae under different treatments. Control (no treatment); M: Rhizophagus irregularis; C: Compost; MC: Compost + Rhizophagus irregularis; NPK: Conventional chemical fertilizer; V: Verticillium dahliae; MV: Rhizophagus irregularis + V. dahliae; CV: Compost + V. dahliae; MCV: Rhizophagus irregularis + Compost + V. dahliae. Data are mean ± SE of five biological replicates. Means that share the same letters are not significantly different at p < 0.05 (Tukey’s HSD)
3.7 Biostimulants Reinforced the Antioxidant Defense Systems
Moreover, the application of biostimulants markedly enhanced polyphenoloxidase (PPO) and peroxidase (POX) activities, as well as superoxide dismutase (SOD) and ascorbate peroxidase (APX) levels in comparison to the control. Notably, in the absence of V. dahliae, plants treated with M exhibited the highest increase in SOD levels (292.0%), followed by those treated with C (222.1%), MC (149.1%), and NPK (119.0%) relative to the control (Fig. 5a).
Figure 5: SOD: superoxide dismutase (a), APX: ascorbate peroxidase activity (b), POX: peroxidase activity (c) and PPO: polyphenol oxidase activity (d) of tomatoes infected or uninfected with V. dahliae under different treatments. Control (no treatment); M: Rhizophagus irregularis; C: Compost; MC: Compost + Rhizophagus irregularis; NPK: Conventional chemical fertilizer; V: Verticillium dahliae; MV: Rhizophagus irregularis + V. dahliae; CV: Compost + V. dahliae; MCV: Rhizophagus irregularis + Compost + V. dahliae. Data are mean ± SE of five biological replicates. Means that share the same letters are not significantly different at p < 0.05 (Tukey’s HSD)
Likewise, APX activity was significantly boosted by MC, showing an increase of 197.2%, M (197.2%), C (159.4%) and NPK (125.6%) (Fig. 5b). However, the activity of POX (Fig. 5c) was enhanced by 66.3%, 55.9%, 28.1%, 8.6% and 167.1% in M, MC, C and NPK respectively compared to the control plants. Additionally, the biostimulants significantly increased PPO enzyme activity by 69.6%, 66.2%, 50.6% and 21.6% in tomato plants inoculated with MC, M, NPK and C respectively, compared to the controls (Fig. 5d). Furthermore, in the presence of V. dahliae, the SOD, APX, POX and PPO activity were significantly increased in tomato plants affected. The biostimulants’ application significantly enhanced these parameters compared to inoculated plants with V. dahliae alone. Our findings demonstrated a maximal increase in SOD values in the MV treatment by 25.8%, followed by CV (22.6%) and MCV by (7.2%), Our results showed a maximum increase in SOD values in the MV treatment of 25.8%, followed by CV (22.6%) and MCV (7.2%). In addition, the highest APX values were recorded in MCV treatments (75.1%) compared with V controls. However, POX activity was highest in the MCV treatment (101.8%), followed by the CV (48.4%) and MV (49.2%) treatments compared with the V controls. In addition, MCV treatment showed a significant improvement in PPO activity of 215.1% compared with V controls (Fig. 5d). The interaction M × D and C × D had a significant effect (p < 0.001) for peroxidase activity and polyphenol oxidase activity (Table S1).
3.8 Heat Map for Biochemical Traits of Tomato
The heat map illustrates the comparative performance of plants treated in the absence and presence of V. dahliae. The color scale indicates that the darkest red represents the highest values, whereas the darkest blue denotes the lowest values for tomatoes (Fig. 6). Of the different treatments tested, compared to uninoculated or unmodified plants, MCV treatment was found to be the most beneficial in increasing TSS and protein in the presence of the pathogen. The organic (total soluble sugars and proline) osmolytes increased following the pathogen infection. However, the application of CV increased the values of proline compared to the control plants. MCV and CV treatments registered the highest levels of peroxidase, superoxide dismutase, polyphenoloxidase, and ascorbate peroxidase activities in the presence of V. dahliae demonstrating their effective role in the V dahlia resistance mechanisms. In control (V) and VNPK plants, markers of stress (MDA and H2O2) showed an opposite effect in the presence of pathogens, having higher values than the rest of the biostimulants MC, CV and MCV. However, MCV showed the highest values of the frequency and intensity of tomato root colonization compared to the other treatments. Physiological parameters and photosynthetic pigments, such as gs, Fv/Fm, Chl a, b, T, and carotenoids, exhibited significant variation between tomato seedlings grown under normal conditions and those infected with a pathogen. MCV and VNPK displayed the highest values for these parameters in the absence of infection, whereas V. dahliae infection resulted in a reduction of these parameters. In addition, the heat map analysis indicates that the highest values of disease symptoms (DI, FMS, AUPDC and LAI) were found in the non-inoculated and non-amended control (V treatment) compared to the plants treated by biostimulants. The lowest values of disease symptoms (DI, FMS, AUPDC and LAI) were found in MC, CV and MCV treatment.
Figure 6: Heat map analysis of tomato plants subjected to various treatments in absence or presence of V.dahliae. The variables were (Disease symptoms, mycorrhization assessment, physiological and biochemical parameters). Protein; TSS: total soluble sugar; Proline; SOD: superoxide dismutase; POX: peroxidase activity; PPO: polyphenoloxidase activity; APX: Ascorbate peroxidase; Chl T: total chlorophyll; Chl a: chlorophyll a; Chl b: chlorophyll b; Car: carotenoids; MDA: malonydialdehyde; H2O2: hydrogen peroxide; Fv/Fm: chlorophyll fluorescence; gs: stomatal conductance; % F and % I: frequency and intensity of mycorrhization; DI: disease incidence; FMS: final mean severity; LAI: Leaf alteration index; AUDPC: area under the disease progress curve; R Roots and R Epicotyls: the re-isolation of the V. dahliae from tomato roots and epicotyls. The heat map’s lowest value is dark blue, brilliant red represents highest value, and the middle values are represented by the white color. There is a corresponding transition (or gradient) between these extremes in the color gradient
Plants are affected by vasa phytopathogenic fungus Verticillium dahliae that infects plants through their roots, causing a range of crop failures and significant economic losses. This fungus causes monocyclic disease, characterized by a single cycle of infection and inoculum formation each growing season. In order to combat infection by V. dahliae, plants have evolved a wide range of defence strategies, including specific resistance genes and inducible defence signalling pathways. Several research have investigated the impact of compost and M on Verticillium-infected crop performance, showing proportional benefits for different crops under pathogen attack [46,47]. Our data revealed that the pathogen reduced mycorrhization characteristics. In contrast, using M in conjunction with compost resulted in higher intensity and frequency of mycorrhization in tomatoes. This increase in mycorrhization parameters is attributed to the availability of phosphate and humic acid in the compost, which promote the elongation of M hyphae [48] and improve hyphae development and spore germination [49]. Zeng et al. [50] demonstrated that compost addition increased the hyphal development and sporulation of M fungus, while having no effect on soil community composition. Medina et al. [51] found that compost-enriched soil exhibited increased hyphal growth and enhanced mycorrhizal activity. Further investigation into molecular interactions and plant defense responses is necessary to better understand the mechanisms behind suppressing common tomato phytopathogen attacks [52]. In the presence of V. dahliae, the mycorrhizal consortium likely induced significant genetic changes, enhancing the signaling systems of tomato plants’ defense against phytopathogens [53,54], exhibited higher mycorrhization frequency and intensity than mycorrhized plants. This supports the basic premise that inoculation with M and adding compost would provide significant synergistic effects. These measures will encourage and promote the developpement of a mycorrhizal symbiosis in tomato crops, while at the same time inhibiting V. dahliae [55].
The study found that the combination of biostimulants was more efficacious in managing disease than when compost and M applied individually. These findings suggest that combining compost and M could effectively suppress the disease. In fact, our findings revealed that the pathogen was less aggressive in plants treated with combined or individual M treatments than infected control plants. Similarly to the our finding, previous research has demonstrated that mycorrhized plants are less susceptible to pathogenic fungus, Early inoculation of the Rhizolive consortium has been shown to reduce symptoms of Verticillium wilt in olive trees and stimulate lignification, total polyphenol levels and phenylalanine ammonia lyase [33]. The processes of mycorrhization reinforce the mechanisms of antioxidant potential of the plants colonised by M [53]. The protection against pathogens offered by M might involve several mechanisms: its ability to competitively colonize host plant roots, enhance nutrient uptake, activate plant defense responses, and produce fungal molecules that suppress pathogenic fungi [56]. In the presence of pathogens, inoculation with M results in a heightened accumulation of various antioxidant enzymes, hydrolases, and hormones in plants. This includes increases in phenylalanine ammonia-lyase, superoxide dismutase, ethylene, abscisic acid, as well as β-1,3-glucanase and chitinase [57]. Pre-emptive mycorrhization may be beneficial as it allows the mycorrhizal fungi to colonise and to protect the root cortex from the invasion of the pathogen, thereby reducing vascular colonisation by V. dahliae. Similarly, In mycorrhized bean roots, Fusarium solani growth was limited to the epidermis and cortical tissue, whereas the pathogen penetrated the vascular stele in non-mycorrhizal roots, causing more severe damage than in mycorrhizal roots [58]. Furthermore, M colonisation significantly increased the upregulation of genes associated with disease resistance and lignin synthesis [59]. On the other hand, our results align with previous research indicating that compost can prevent diseases caused by pathogens [60]. In addition, M has been shown to minimize Alternaria alternata damage to tomato and bean plants [61,62]. Furthermore, it appears that plants control the rhizosphere microbiome by producing chemicals that selectively stimulate bacteria that support plant development and health while suppressing pathogens that damage plants [63].
Our findings showed that the capacity of the biostimulants utilized, particularly the tripartite combination, to boost photosynthetic activity and CO2 absorption in tomato may account for the enhancement in physiological parameters under normal conditions in the absence of pathogen [64,65]. Application of V. dahliae treatment reduced gs and Fv/Fm, this finding is consistent with results in tomato plants [24]. Tomato plants treated with M and compost, whether applied individually or in combination, exhibit enhanced gs and Fv/Fm in response to V. dahliae infection. This improvement in photosynthetic traits is likely to be due to the increased availability and uptake of key minerals, including magnesium, potassium, nitrogen, and phosphorus, facilitated by both compost and M mycelium. These minerals are essential for photosynthesis as they are integral to the formation of key molecules such as enzymes and chlorophyll pigments [66]. These favorable benefits can be attributed to improved supply of water due to compost, which is a lightweight humus-rich soil amendment that improves water retention, and to the greater resistance of tomato xylem vessels to pathogen colonisation in soils containing compost [67].
Furthermore, the results demonstrated that M and/or compost boosted the chlorophyll content of tomato leaves, particularly when M spores were inoculated without pathogen infection. Previous research described by Othman et al. [68] confirmed similar findings, which discovered higher chlorophyll content in basil plants infected with G intraradices.
Plants possess a range of defense strategies to counteract the deleterious impact of biotic stress, including the accumulation of specific osmolytes in the cytosol [69]. Additionally, inoculation with M and/or the amendment of compost positively influenced the accumulation of key osmoprotectants, including glycine betaine, soluble sugars, and proline. These compounds help safeguard photosynthesis by enhancing the integrity of chloroplast membranes [70]. In the current investigation, the use of biostimulants in conjunction with V. dahliae resulted in increased protein content in tomato plants compared to those infected with the pathogen alone. This elevated protein content is likely a result of heightened biosynthesis in response to biotic stress, with the aim of preserving cellular homeostasis. The accumulation of osmoprotectants like proteins could induce physicochemical alterations in stressed plants, contributing to the production of defensive compounds [71]. Increasing cellular osmolytes is widely acknowledged to help plants maintain cellular function, thereby ensuring physiological stability under stress [72]. Plants treated with compost and/or M can collect more osmolytes, potentially preserving and increasing photosynthetic activity in severe conditions [73]. In our study, proline content was higher in MV than (diseased) control treatment (V). These results align with those obtained by Yao et al. [74], who discovered that applying M led to increased proline accumulation in tobacco plants, in comparison to the treatment of Phytophthora nicotianae alone. According to Ashraf et al. [75], proline plays crucial roles in osmotic adjustment, stabilizing cellular redox balance, preserving membrane and protein integrity, and scavenging free radicals under stressful conditions. Additionally, it serves as a pH regulator and prevents macromolecules from denaturing. Consequently, proline enhances plant tolerance by bolstering the antioxidant system rather than solely through osmotic adjustment [76]. In addition, inoculation with V. dahliae caused TSS to accumulate. The increased levels of total soluble sugar (TSS) in V. dahliae infection can be ascribed to the swift synthesis of molecules responsive to stress, which aid in preserving cellular homeostasis. Plants inoculated with M and/or compost could potentially accumulate greater amounts of osmoprotectants under stress conditions, thereby protecting and enhancing their photosynthetic activity [77]. These biostimulants are effective in improving tomato resistance to V. dahliae due to the significant accumulation of osmolytes.
However, ROS have potent oxidizing properties that can cause the degradation of DNA proteins, and lipids, ultimately causing cellular damage and death. The generation of ROS serves as an initial response in plants, acting as a vital signal to adapt to environmental stressors and activate defence mechanisms [78,79]. In our results, Plants infected with V. dahliae showed elevated levels of MDA and H2O2. These elevated levels reflect significant membrane lipid peroxidation due to the presence of the pathogen, which compromises membrane integrity and function by reacting with unsaturated lipids, leading to extensive cellular damage [80]. Maldonado et al. [81] discovered that ROS generation might be caused by toxins generated by pathogenic fungus that disrupt host plant metabolism. The significant increase in MDA levels in plants exposed to V. dahliae may induce overproduction of antioxidant enzymes, which serve as biochemical markers of plant disease resistance [82]. Conversely, application of compost and M, either individually or together, significantly reduced root MDA levels in tomato plants exposed to V. dahliae. The results are consistent with those reported by Nafady et al. [83] and Xiang et al. [84], who demonstrated that mycorrhizal plants exhibit reduced MDA levels following pathogen infection. High levels of H2O2 at infection sites act as an initial defence against pathogen invasion. This accumulation may be related to the rapid senescence observed in V. dahliae infected plants, particularly when inoculated with strain M [85]. The observed reduction in MDA levels following biostimulants administration, even in the presence of pathogens, may be due to improved antioxidant enzyme activity, as demonstrated in the current experiment and prior investigations [33]. The ability of compost and M to improve the antioxidant system has been shown in many previous studies [64–86]. Plants infected with the pathogen may create and store both non-enzymatic and enzymatic antioxidants to defend themselves from oxidative stress damage. Our research indicated a marked elevation in the activity of antioxidant enzymes (APX, SOD, POX, and PPO) upon exposure to the pathogen, particularly in tomatoes inoculated with M. Plants inoculated with biostimulants have an enhanced antioxidant system compared to unmodified and non-mycorrhizal plants. SOD is the first antioxidant enzyme, converting superoxide radicals to hydrogen peroxide and oxygen [87]. PPO is considered to be a key enzyme in the defence against pathogens and plays an important role in the lignification of the cells of the host plant that are affected [88].
Thus, the effectiveness of controlling wilt in tomatoes for sustainable agriculture may be due to combining compost and M [46]. In general, plant nutrition influences development and defense systems against pathogens [89]. Specifically, regarding Verticillium wilt, employing low doses of nitrogen, along with decreasing calcium levels in the soil, or increasing potassium and magnesium, seems to be associated with a decrease in both the incidence and severity of the disease [90]. Mineral nutrition has already been shown to have an influence on Verticillium wilt infections in several crops, which may result in a reduction or an increase in wilt symptoms [47]. In our investigation, the administration of NPK impacts the severity and incidence of V. dahliae disease. These results are in line with those previously described by López-Escudero et al. [91], indicating that the use of fertilizer combined with regular irrigation in soils naturally infested with pathogens increases the occurrence of Verticillium wilt in olive trees. Farmers and agronomists worry that excessive nitrogen fertilization worsens disease in olive trees. Nitrogen significantly impacts a wide range of pathogens and their hosts, affecting disease development regardless of the amount applied. Nitrogen’s influence on plant diseases arises from direct effects, like changing pathogen growth or virulence, and indirect effects, such as altering plant physiology and the soil environment, particularly in the rhizosphere where soil-borne pathogens thrive [92]. The impact of N on the infection of V. dahliae has been demonstrated in a vast range of herbaceous hosts, however the literature contains inconsistent data. Excess N is thought to promote disease incidence, whereas NO3− and NH4+ have been shown to decrease the number of V. dahliae propagules in the soil, thereby helping to control the disease [93]. Regarding the impact of potassium (K) on plant diseases, Fontana et al. [94] noted that potassium, either alone or combined with nitrogen (N) or other nutrients, affects the severity of diseases caused by various soil-borne pathogens, such as Verticillium wilt. Indeed, applying potassium to potassium-deficient soils enhances the plant’s resistance to diseases, including Verticillium wilt in cotton [95]; Nonetheless, the comparison between biostimulants and conventional chemical fertilizers has received less attention in the context of sustainable agriculture. The efficacy of Rhizophagus irregularis and compost against V. dahliae compared with conventional chemical fertilizers offers interesting prospects for sustainable agriculture. Studying how Rhizophagus irregularis enhances plant resistance to pathogenic fungi could inspire new biocontrol methods, while long-term studies should prioritize sustainability measures such as soil health indicators, carbon sequestration rates and improved biodiversity in agricultural ecosystems. In addition, the integration of economic analyses to assess profitability and commercial viability is crucial for the practical implementation and large-scale adoption of sustainable agriculture.
This study combines physiological and biochemical features to give fresh light on the impact of M and/or C compared to NPK application on tomato resistance to V. dahliae infection. Particularly, under pathogen infection, M colonization and compost amendment enhanced tolerance in tomato plants due to better photosynthetic apparatus, osmoprotectants and antioxidant potential in conjunction with lower H2O2 and MDA content. Additionally, M, and/or C treatments reduced the excess production of ROS, resulting in structural and functional stability, which mitigated the oxidative consequences caused by V. dahliae. The application of compost and/or M is potentially vital for improving the growth, yield, and V. dahliae resistance in tomatoes. Tomatoes inoculated with M and treated with compost have shown a marked reduction in the negative impacts of V. dahliae, as demonstrated by enhanced tomato physiology and lowered MDA and H2O2 levels when exposed to V. dahliae. Significantly, MCV presented the highest activities of POX, PPO, and APX, whereas CV had the most substantial increase in SOD activity. Future research should focus on the extended effects of M and compost on soil health, microbial community dynamics, and crop productivity in diverse agricultural settings. The integration of advanced molecular techniques and the exploration of synergies with other biocontrol agents could optimize the effectiveness of these treatments in agricultural environments. In conclusion, the use of compost and microbial communities represents a promising strategy for improving tomato resistance to V. dahlia. This strategy provides viable solutions for agricultural sustainability and ensures food security amidst evolving environmental challenges.
Acknowledgement: The current work was funded by Plant, Animal Productions and Agro-Industry Laboratory, Department of Biology, Faculty of Sciences, Ibn Tofail University.
Funding Statement: The study did not receive any designated funding.
Author Contributions: Allal Douira, Rachid Benkirane, Rachid Lahlali and Abdelilah Meddich designed and supervised the research. Fatima-Ezzahra Soussani conducted the experiments, analyzed the results, and interpreted the data. Ait Rahou Youssef and Ikan Chayma contributed to monitoring of the manipulation and the analytical tools. Ait Barka Essaid contributed to the editing of the manuscript, and was involved in funding acquisition and investigation. Fatima-Ezzahra Soussani drafted the manuscript, which was then reviewed and corrected by Allal Douira, Rachid Benkirane, Rachid Lahlali and Abdelilah Meddich. All authors reviewed the results and approved the final version of the manuscript.
Availability of Data and Materials: All data produced or examined in the course of this study are contained within this published article.
Ethics Approval: Not applicable.
Conflicts of Interest: The authors declare that they have no conflicts of interest to report regarding the present study.
Supplementary Materials: The supplementary material is available online at https://doi.org/10.32604/phyton.2024.055050.
References
1. Witzel K, Buhtz A, Grosch R. Temporal impact of the vascular wilt pathogen Verticillium dahliae on tomato root proteome. J Proteomics. 2017;169:215–24. doi:10.1016/J.JPROT.2017.04.008. [Google Scholar] [PubMed] [CrossRef]
2. Inderbitzin P, Subbarao KV. Verticillium systematics and evolution: how confusion impedes verticillium wilt management and how to resolve it. AM Phytopat Soc. 2014;104(6):564–74. doi:10.1094/PHYTO-11-13-0315-IA. [Google Scholar] [PubMed] [CrossRef]
3. Ma M, Taylor PWJ, Chen D, Vaghefi N, He JZ. Major soilborne pathogens of field processing tomatoes and management strategies. Microorg. 2023;11(2):263. doi:10.3390/MICROORGANISMS11020263. [Google Scholar] [PubMed] [CrossRef]
4. Ali MY, Sina AAI, Khandker SS, Neesa L, Tanvir EM, Kabir A, et al. Nutritional composition and bioactive compounds in tomatoes and their impact on human health and disease: a review. Foods. 2021;10(1):45. doi:10.3390/FOODS10010045. [Google Scholar] [PubMed] [CrossRef]
5. Szabo K, Cătoi AF, Vodnar DC. Bioactive compounds extracted from tomato processing by-products as a source of valuable nutrients. Plant Foods Hum Nutr. 2018;73:268–77. doi:10.1007/S11130-018-0691-0/METRICS. [Google Scholar] [CrossRef]
6. Wang S, Qiang Q, Xiang L, Fernie AR, Yang J. Targeted approaches to improve tomato fruit taste. Hortic Res. 2023;10(1):815. doi:10.1093/HR/UHAC229. [Google Scholar] [PubMed] [CrossRef]
7. Liu K, McInroy JA, Hu CH, Kloepper JW. Mixtures of plant-growth-promoting rhizobacteria enhance biological control of multiple plant diseases and plant-growth promotion in the presence of pathogens. Plant Dis. 2018;102(1):67–72. doi:10.1094/PDIS-04-17-0478-RE/ASSET/IMAGES/LARGE/PDIS-04-17-0478-RE_T3.JPEG. [Google Scholar] [CrossRef]
8. Wang H, Tang C, Deng C, Li W, Klosterman SJ, Wang Y. Septins regulate virulence in Verticillium dahliae and differentially contribute to microsclerotial formation and stress responses. Phytopathol Res. 2022;4(1):1–14. doi:10.1186/s42483-022-00145-x. [Google Scholar] [CrossRef]
9. Akbar MU, Aqeel M, Shah MS, Jeelani G, Iqbal N, Latif A, et al. Molecular regulation of antioxidants and secondary metabolites act in conjunction to defend plants against pathogenic infection. S Afr J Bot. 2023;161(8):247–57. doi:10.1016/J.SAJB.2023.08.028. [Google Scholar] [CrossRef]
10. Sarkar M, Upreti R, Kumar S. Current status of tomato (Solanum lycopersicum L.) diseases and their management. Dis Hortic Crop. 2022;465–521. doi:10.1201/9781003160427-18. [Google Scholar] [CrossRef]
11. Chavan S, Nadanathangam V. Effects of nanoparticles on plant growth-promoting bacteria in Indian agricultural soil. Agronomy. 2019;9(3):140. doi:10.3390/AGRONOMY9030140. [Google Scholar] [CrossRef]
12. Chojnacka K. Sustainable chemistry in adaptive agriculture: a review. Curr Opin Green Sustain Chem. 2024;46:100898. doi:10.1016/J.COGSC.2024.100898. [Google Scholar] [CrossRef]
13. Kumar A, Dutta J, Chandrawanshi NK, Ekka A, Sethi SK. Arbuscular mycorrhizal fungi in alleviation of biotic stress tolerance in plants: a new direction in sustainable agriculture. Entrep with Microorg. 2024;2024(1):355–69. doi:10.1016/B978-0-443-19049-0.00006-2. [Google Scholar] [CrossRef]
14. Dowarah B, Gill SS, Agarwala N. Arbuscular mycorrhizal fungi in conferring tolerance to biotic stresses in plants. J Plant Growth Regul. 2021;41(4):1429–44. doi:10.1007/S00344-021-10392-5. [Google Scholar] [CrossRef]
15. Bello TT, Fabiyi OA. Advancement in mycorrhizal fungi-based sustainable plant disease management. Mycorrhizal Symbiosis Agroecosystem Restor. 2024;109(2):125–45. doi:10.1007/978-981-99-5030-0_6. [Google Scholar] [CrossRef]
16. Vishwakarma SK, Ilyas T, Malviya D, Shafi Z, Shahid M, Yadav B, et al. Arbuscular mycorrhizal fungi (AMF) as potential biocontrol agents. Rhizosphere Microb: Biotic Stress Manag. 2022;40(9):197–222. doi:10.1007/978-981-19-5872-4_10. [Google Scholar] [CrossRef]
17. Chandrasekaran M, Paramasivan M. Arbuscular mycorrhizal fungi and antioxidant enzymes in ameliorating drought stress: a meta-analysis. Plant Soil. 2022;480(1):295–303. doi:10.1007/S11104-022-05582-3/METRICS. [Google Scholar] [CrossRef]
18. Abarca C, Fernandez Bidondo L, Bompadre J, Velázquez MS. Arbuscular mycorrhizal fungi in tomato tolerance to pathogens and nematodes: a comprehensive review. Sci Hortic. 2024;329(16):112969. doi:10.1016/J.SCIENTA.2024.112969. [Google Scholar] [CrossRef]
19. El Kinany S, Achbani E, Faggroud M, Ouahmane L, El Hilali R, Haggoud A, et al. Effect of organic fertilizer and commercial arbuscular mycorrhizal fungi on the growth of micropropagated date palm cv. Feggouss. J Saudi Soc Agric Sci. 2019;18(4):411–7. doi:10.1016/j.jssas.2018.01.004. [Google Scholar] [CrossRef]
20. Begum N, Qin C, Ahanger MA, Raza S, Khan MI, Ashraf M, et al. Role of arbuscular mycorrhizal fungi in plant growth regulation: implications in abiotic stress tolerance. Front Plant Sci. 2019;10:1068. doi:10.3389/FPLS.2019.01068/BIBTEX. [Google Scholar] [CrossRef]
21. Kullu B, Patra DK, Acharya S, Pradhan C, Patra HK. AM fungi mediated bioaccumulation of hexavalent chromium in brachiaria mutica–a mycorrhizal phytoremediation approach. Chemosphere. 2020;258(1):127337. doi:10.1016/J.CHEMOSPHERE.2020.127337. [Google Scholar] [PubMed] [CrossRef]
22. De Corato U. Agricultural waste recycling in horticultural intensive farming systems by on-farm composting and compost-based tea application improves soil quality and plant health: a review under the perspective of a circular economy. Sci Total Environ. 2020;738(3):139840. doi:10.1016/J.SCITOTENV.2020.139840. [Google Scholar] [PubMed] [CrossRef]
23. Tubeileh AM, Stephenson GT. Soil amendment by composted plant wastes reduces the Verticillium dahliae abundance and changes soil chemical properties in a bell pepper cropping system. Curr Plant Biol. 2020;22:100148. doi:10.1016/j.cpb.2020.100148. [Google Scholar] [CrossRef]
24. Ait Rahou Y, Ait-El-Mokhtar M, Anli M, Boutasknit A, Ben-Laouane R, Douira A, et al. Use of mycorrhizal fungi and compost for improving the growth and yield of tomato and its resistance to Verticillium dahliae. Arch Phytopathol Plant Prot. 2020;54(13–14):665–90. doi:10.1080/03235408.2020.1854938. [Google Scholar] [CrossRef]
25. Voss M, Valle C, Gaudino EC, Tabasso S, Forte C, Cravotto G. Unlocking the potential of agrifood waste for sustainable innovation in agriculture. Recycling. 2024;9(2):25. doi:10.3390/RECYCLING9020025. [Google Scholar] [CrossRef]
26. Huda N, Rasel Rana M, Mizanur Rahman M, Amdadul Huq M, Tasbir Rahman S, Rahman F, et al. Vermicompost and organic manure interactions: effects on heavy metal concentrations, nitrification activity, comammox Nitrospira inopinata, and archaea/bacteria. Res Sqaure. 2023;8(2):175. doi:10.21203/RS.3.RS-2993924/V1. [Google Scholar] [CrossRef]
27. Antón-Domínguez BI, Díaz-Díaz M, Acedo-Antequera FA, Trapero C, Agustí-Brisach C. Use of natural-based commercial products as an alternative for providing bioprotection against verticillium wilt of olive. J Sci Food Agric. 2024;104(10):6311–21. doi:10.1002/JSFA.13461. [Google Scholar] [PubMed] [CrossRef]
28. Meddich A, Elouaqoudi FZ, Khadra A, Bourzik W. Valorization of plant and industrial waste through composting. Rev Des Compos Des Mater Av. 2016;26(3–4):451–69 (In French). doi:10.3166/RCMA.26.451-469. [Google Scholar] [CrossRef]
29. Elalaoui AC. Mineral fertilization of crops: major fertilizing elements (N, P, K). Transf Technol En Agric. 2007;4 (In French). [Google Scholar]
30. Phillips JM, Hayman DS. Improved procedures for clearing roots and staining parasitic and vesicular-arbuscular mycorrhizal fungi for rapid assessment of infection. Trans Br Mycol Soc. 1970;55(1):158–IN18. doi:10.1016/S0007-1536(70)80110-3. [Google Scholar] [CrossRef]
31. Trouvelot A, Kough JL, Gianinazzi-Pearson V. Measurement of VA mycorrhization rate in a root system: seeking estimation methods with functional significance. Physiol Genet Asp Mycorrhizae. 1986;217–21 (In French). [Google Scholar]
32. El Said S, Hegazi A, Allatif A. Resistance of some olive cultivars to verticillium wilt. J Appl Sci Res. 2012;8:2758–65. [Google Scholar]
33. Boutaj H, Chakhchar A, Meddich A, Wahbi S, El Alaoui-Talibi Z, Douira A, et al. Bioprotection of olive tree from verticillium wilt by autochthonous endomycorrhizal fungi. J Plant Dis Prot. 2020;127:349–57. doi:10.1007/S41348-020-00323-Z/METRICS. [Google Scholar] [CrossRef]
34. Douira A, Ben Kirane R, Ouazzani Touhami A, Okeke B, Elhaloui NE. Verticillium wilt of pepper (Capsicum annuum) in Morocco. J Phytopathol. 1995;143(8):467–70. doi:10.1111/J.1439-0434.1995.TB04556.X. [Google Scholar] [CrossRef]
35. Campbell CL, Madden LV. Introduction to plant disease epidemiology. New York, USA: John Wiley & Sons; 1990. p. 532. [Google Scholar]
36. Arnon DI. Copper enzymes in isolated chloroplasts. Polyphenoloxidase in beta vulgaris. Plant Physiol. 1949;24(1):1–15. doi:10.1104/PP.24.1.1. [Google Scholar] [PubMed] [CrossRef]
37. Velikova V, Yordanov I, Edreva A. Oxidative Stress and some antioxidant systems in acid rain-treated bean plants protective role of exogenous polyamines. Plant Sci. 2000;151(1):59–66. doi:10.1016/S0168-9452(99)00197-1. [Google Scholar] [CrossRef]
38. Dhindsa RS, Plumb-dhindsa P, Thorpe TA. Leaf senescence: correlated with increased levels of membrane permeability and lipid peroxidation, and decreased levels of superoxide dismutase and catalase. J Exp Bot. 1981;32(1):93–101. doi:10.1093/JXB/32.1.93. [Google Scholar] [CrossRef]
39. DuBois M, Gilles KA, Hamilton JK, Rebers PA, Smith F. Colorimetric method for determination of sugars and related substances. Microb Cell Fact. 2002;8(3):59–356. doi:10.1021/AC60111A017. [Google Scholar] [CrossRef]
40. Bradford M. A rapid and sensitive method for the quantitation of microgram quantities of protein utilizing the principle of protein-dye binding. Anal Biochem. 1976;72(1–2):248–54. doi:10.1006/ABIO.1976.9999. [Google Scholar] [PubMed] [CrossRef]
41. Carillo P, Mastrolonardo G, Nacca F, Parisi D, Verlotta A, Fuggi A. Nitrogen metabolism in durum wheat under salinity: accumulation of proline and glycine betaine. Funct Plant Biol. 2008;35(5):412–26. doi:10.1071/FP08108. [Google Scholar] [PubMed] [CrossRef]
42. Tejera García NA, Olivera M, Iribarne C, Lluch C. Partial purification and characterization of a non-specific acid phosphatase in leaves and root nodules of Phaseolus vulgaris. Plant Physiol Biochem. 2004;42(7–8):585–91. doi:10.1016/J.PLAPHY.2004.04.004. [Google Scholar] [PubMed] [CrossRef]
43. Hori K, Ayako WST. NII-electronic library service. Chem Pharm Bull. 1997;57. [Google Scholar]
44. Amako K, Chen GX, Asada K. Separate assays specific for ascorbate peroxidase and guaiacol peroxidase and for the chloroplastic and cytosolic isozymes of ascorbate peroxidase in plants. Plant Cell Physiol. 1994;35(3):497–504. doi:10.1093/OXFORDJOURNALS.PCP.A078621. [Google Scholar] [CrossRef]
45. Beyer WF, Fridovich I. Assaying for superoxide dismutase activity: some large consequences of minor changes in conditions. Anal Biochem. 1987;161(2):559–66. doi:10.1016/0003-2697(87)90489-1. [Google Scholar] [PubMed] [CrossRef]
46. Bilgili A, Bilgili AV. Comparison of compost, PGPR, and AMF in the biological control of tomato Fusarium wilt disease. Eur J Plant Pathol. 2023;167(4):771–86. doi:10.1007/S10658-023-02710-2/METRICS. [Google Scholar] [CrossRef]
47. Kowalska B. Management of the soil-borne fungal pathogen–Verticillium dahliae Kleb. causing vascular wilt diseases. J Plant Pathol. 2021;103(4):1185–94. doi:10.1007/s42161-021-00937-8. [Google Scholar] [CrossRef]
48. Zhao YY, Cartabia A, Lalaymia I, Declerck S. Arbuscular mycorrhizal fungi and production of secondary metabolites in medicinal plants. Mycorrhiza. 2022;32(3):221–56. doi:10.1007/S00572-022-01079-0. [Google Scholar] [PubMed] [CrossRef]
49. Ortuño MF, Lorente B, Hernández JA, Sánchez-Blanco MJ. Mycorrhizal inoculation on compost substrate affects nutritional balance, water uptake and photosynthetic efficiency in Cistus albidus plants submitted to water stress. Rev Bras Bot. 2018;41(2):299–310. doi:10.1007/S40415-018-0457-9. [Google Scholar] [CrossRef]
50. Zeng ZW, Tan XF, Liu YG, Tian SR, Zeng GM, Jiang LH, et al. Comprehensive adsorption studies of doxycycline and ciprofloxacin antibiotics by biochars prepared at different temperatures. Front Chem. 2018;6:339755. doi:10.3389/FCHEM.2018.00080/BIBTEX. [Google Scholar] [CrossRef]
51. Medina A, Vassilev N, Alguacil MM, Roldán A, Azcón R. Increased plant growth, nutrient uptake, and soil enzymatic activities in a desertified mediterranean soil amended with treated residues and inoculated with native mycorrhizal fungi and a plant growth-promoting yeast. Soil Sci. 2004;169(4):260–70. doi:10.1097/01.ss.0000126840.65846.02. [Google Scholar] [CrossRef]
52. Sharma A, Sharma A, Sharma A, Kumar Y, Sharma P, Bhardwaj R, et al. Polyphenol phytoalexins as the determinants of plant disease resistance. Plant Phenolics Biot Stress Manag. 2024;2024(1):243–74. doi:10.1007/978-981-99-3334-1_10. [Google Scholar] [CrossRef]
53. Ghosh UK, Islam MN, Siddiqui MN, Cao X, Khan MAR. Proline, A multifaceted signalling molecule in plant responses to abiotic stress: understanding the physiological mechanisms. Plant Biol. 2022;24(2):227–39. doi:10.1111/PLB.13363. [Google Scholar] [PubMed] [CrossRef]
54. Zhang X, Li M, Yang H, Li X, Cui Z. Physiological responses of Suaeda glauca and Arabidopsis thaliana in phytoremediation of heavy metals. J Environ Manag. 2018;223:132–9. doi:10.1016/J.JENVMAN.2018.06.025. [Google Scholar] [PubMed] [CrossRef]
55. Mbogning S, Okiobe ST, Theuerl S, Nwaga D. Synergistic interplay between arbuscular mycorrhizal fungi and fern manure compost tea suppresses common tomato phytopathogens and pest attacks on-farm. Front Hortic. 2024;3:1253616. doi:10.3389/FHORT.2024.1253616. [Google Scholar] [CrossRef]
56. Abo-Elyousr KAM, Seleim MEA, Abd-El-Moneem KMH, Saead FA. Integrated effect of Glomus mosseae and selected plant oils on the control of bacterial wilt disease of tomato. Crop Prot. 2014;66:67–71. doi:10.1016/J.CROPRO.2014.07.022. [Google Scholar] [CrossRef]
57. Wang C, Linderholm HW, Song Y, Wang F, Liu Y, Tian J, et al. Impacts of drought on maize and soybean production in northeast China during the past five decades. Int J Environ Res Public Health. 2020;17(7):2459. doi:10.3390/ijerph17072459. [Google Scholar] [PubMed] [CrossRef]
58. Eke P, Chatue Chatue G, Wakam LN, Kouipou RMT, Fokou PVT, Boyom FF. Mycorrhiza consortia suppress the fusarium root rot (Fusarium solani f. sp. Phaseoli) in common bean (Phaseolus vulgaris L.). Biol Control. 2016;103:240–50. doi:10.1016/J.BIOCONTROL.2016.10.001. [Google Scholar] [CrossRef]
59. Zhang F, Zou Y, Wu Q. Quantitative estimation of water uptake by mycorrhizal extraradical hyphae in citrus under drought stress. Sci Hortic. 2018;229:132–6. doi:10.1016/j.scienta.2017.10.038. [Google Scholar] [CrossRef]
60. Shen T, Lei Y, Pu X, Zhang S, Du Y. Identification and application of Streptomyces microflavus G33 in compost to suppress tomato bacterial wilt disease. Appl Soil Ecol. 2021;157:103724. doi:10.1016/J.APSOIL.2020.103724. [Google Scholar] [CrossRef]
61. Matrood AAA, Khrieba MI, Okon OG. Synergistic interaction of Glomus mosseae T. and Trichoderma harzianum R. in the induction of systemic resistance of Cucumis sativus L. to Alternaria alternata (Fr.) K. Plant Sci Today. 2020;7(1):101–8. doi:10.14719/PST.2020.7.1.629. [Google Scholar] [CrossRef]
62. Prakash J, Egamberdieva D, Arora NK. A novel Bacillus safensis-based formulation along with mycorrhiza inoculation for controlling Alternaria alternata and simultaneously improving growth, nutrient uptake, and steviol glycosides in Stevia rebaudiana under field conditions. Plants. 2022;11(14):1857. doi:10.3390/PLANTS11141857/S1. [Google Scholar] [CrossRef]
63. Abdalla M, Ahmed MA. Arbuscular mycorrhiza symbiosis enhances water status and soil-plant hydraulic conductance under drought. Front Plant Sci. 2021;12:722954. doi:10.3389/FPLS.2021.722954/BIBTEX. [Google Scholar] [CrossRef]
64. Soussani FE, Boutasknit A, Ben-Laouane R, Benkirane R, Baslam M, Meddich A. Arbuscular mycorrhizal fungi and compost-based biostimulants enhance fitness, physiological responses, yield, and quality traits of drought-stressed tomato plants. Plants. 2023;12(9):1856. doi:10.3390/PLANTS12091856/S1. [Google Scholar] [CrossRef]
65. Tahiri AI, Meddich A, Raklami A, Alahmad A, Bechtaoui N, Anli M, et al. Assessing the potential role of compost, PGPR, and AMF in improving tomato plant growth, yield, fruit quality, and water stress tolerance. J Soil Sci Plant Nutr. 2021;22(1):743–64. doi:10.1007/S42729-021-00684-W. [Google Scholar] [CrossRef]
66. Shankar V, Tayang A, Evelin H. Mechanisms of arbuscular mycorrhizal fungi-induced drought stress amelioration in plants. Arbuscular Mycorrhizal Fungi High Plants. 2024;2024(1):149–75. doi:10.1007/978-981-99-8220-2_7. [Google Scholar] [CrossRef]
67. Islam T, Haque MA, Barai HR, Istiaq A, Kim JJ. Antibiotic Resistance in Plant Pathogenic Bacteria: recent data and environmental impact of unchecked use and the potential of biocontrol agents as an eco-friendly alternative. Plants. 2024;13(8):1135. doi:10.3390/PLANTS13081135. [Google Scholar] [PubMed] [CrossRef]
68. Othman YA, Alananbeh KM, Tahat MM. Can arbuscular mycorrhizal fungi enhance crop productivity and quality in hydroponics? A meta-analysis. Sustainability. 2024;16(9):3662. doi:10.3390/SU16093662. [Google Scholar] [CrossRef]
69. Shrivastav A, Tripathi MK, Tiwari PN, Singh P. Biochemical changes: their potential role against fungal disease resistance development in mustard. J Adv Biol Biotechnol. 2024;27(5):13–31. doi:10.9734/JABB/2024/V27I5758. [Google Scholar] [CrossRef]
70. Channab BE, EL Idrissi A, Ammar A, Dardari O, Marrane SE, el Gharrak A, et al. Recent advances in nano-fertilizers: synthesis, crop yield impact, and economic analysis. Nanoscale. 2024;16(9):4484–513. doi:10.1039/D3NR05012B. [Google Scholar] [PubMed] [CrossRef]
71. Mascellani Bergo A, Leiss K, Havlik J. Twenty years of 1H NMR plant metabolomics: a way forward toward assessment of plant metabolites for constitutive and inducible defenses to biotic stress. J Agric Food Chem. 2023;72(15):8332–46. doi:10.1021/ACS.JAFC.3C09362/SUPPL_FILE/JF3C09362_SI_003.XLSX. [Google Scholar] [CrossRef]
72. Das D, Abbhishek K, Banik P, Swain DK. Comparative evaluation of changes in soil bio-chemical properties after application of traditional and enriched Vermicompost. Environ Technol Innov. 2022;28(1–2):102956. doi:10.1016/J.ETI.2022.102956. [Google Scholar] [CrossRef]
73. McNeece BT, Sharma K, Lawrence GW, Lawrence KS, Klink VP. The mitogen activated protein kinase (MAPK) gene family functions as a cohort during the glycine max defense response to heterodera glycines. Plant Physiol Biochem. 2019;137(2):25–41. doi:10.1016/J.PLAPHY.2019.01.018. [Google Scholar] [PubMed] [CrossRef]
74. Yao B, Li Y, Zeng W, Yang G, Zeng J, Nie J, et al. Synergistic adsorption and oxidation of trivalent antimony from groundwater using biochar supported magnesium ferrite: performances and mechanisms. Environ Pollut. 2023;323:121318. doi:10.1016/J.ENVPOL.2023.121318. [Google Scholar] [PubMed] [CrossRef]
75. Ashraf M, Foolad MR. Roles of glycine betaine and proline in improving plant abiotic stress resistance. Environ Exp Bot. 2007;59(2):206–16. doi:10.1016/J.ENVEXPBOT.2005.12.006. [Google Scholar] [CrossRef]
76. Rogan CJ, Pang Y-Y, Mathews SD, Turner SE, Weisberg AJ, Lehmann S, et al. Transporter-mediated depletion of extracellular proline directly contributes to plant pattern-triggered immunity against a bacterial pathogen. bioRxiv. 2023;15(1):7048.10.18.562815. doi:10.1101/2023.10.18.562815. [Google Scholar] [CrossRef]
77. Pisuttu C, Lo Piccolo E, Paoli L, Cotrozzi L, Nali C, Pellegrini E, et al. Physiochemical responses of Ailanthus altissima under the challenge of Verticillium dahliae: elucidating the decline of one of the world’s worst invasive alien plant species. Biol Invasions. 2023;25(1):61–78. doi:10.1007/S10530-022-02891-7/FIGURES/9. [Google Scholar] [CrossRef]
78. Huang Z, Zhao F, Wang M, Qi K, Wu J, Zhang S. Soil chemical properties and geographical distance exerted effects on arbuscular mycorrhizal fungal community composition in pear orchards in Jiangsu province. China Appl Soil Ecol. 2019;142:18–24. doi:10.1016/J.APSOIL.2019.05.017. [Google Scholar] [CrossRef]
79. Phull AR, Nasir B, Haq IUL, Kim SJ. Oxidative stress, consequences and ROS mediated cellular signaling in Rheumatoid arthritis. Chem Biol Interact. 2018;281:121–36. doi:10.1016/J.CBI.2017.12.024. [Google Scholar] [PubMed] [CrossRef]
80. Nadarajah KK. Defensive strategies of ROS in plant-pathogen interactions. Plant Pathog Interact. 2023;162(3):163–83. doi:10.1007/978-981-99-4890-1_6. [Google Scholar] [CrossRef]
81. Maldonado E, Rojas DA, Morales S, Miralles V, Solari A. Dual and opposite roles of reactive oxygen species (ROS) in chagas disease: beneficial on the pathogen and harmful on the host. Oxid Med Cell Longev. 2020;2020(5):1–17. doi:10.1155/2020/8867701. [Google Scholar] [PubMed] [CrossRef]
82. Kaur A, Kaur M, Tak Y. Insights into biotic stress management by plants using phenolic compounds. Plant Phenolics Biot Stress Manag. 2024;2024(2):75–93. doi:10.1007/978-981-99-3334-1_3. [Google Scholar] [CrossRef]
83. Nafady NA, Hashem M, Hassan EA, Ahmed HAM, Alamri SA. The combined effect of arbuscular mycorrhizae and plant-growth-promoting yeast improves sunflower defense against Macrophomina phaseolina diseases. Biol Control. 2019;138:104049. doi:10.1016/J.BIOCONTROL.2019.104049. [Google Scholar] [CrossRef]
84. Xiang D, Huang Y, Li L, Zhao M, Liang B. Effects of combinations of arbuscular mycorrhizal fungal species on tomato growth and Fusarium wilt control. Biol Control. 2023;186(20):105353. doi:10.1016/J.BIOCONTROL.2023.105353. [Google Scholar] [CrossRef]
85. Boutaj H, Meddich A, Wahbi S, Moukhli A, Alaoui-Talibi Z, Douira A, et al. Effect of Arbuscular Mycorrhizal Fungi on Verticillium wilt development of olive trees caused by Verticillium dahliae. Res J Biotechnol. 2019;14(8). [Google Scholar]
86. Anli M, Symanczik S, El Abbassi A, Ait-El-Mokhtar M, Boutasknit A, Ben-Laouane R, et al. Use of arbuscular mycorrhizal fungus Rhizoglomus irregulare and compost to improve growth and physiological responses of Phoenix dactylifera ‘Boufgouss’. Plant Biosyst. 2020;155(4):763–71. doi:10.1080/11263504.2020.1779848. [Google Scholar] [CrossRef]
87. Roy Z, Bansal R, Siddiqui L, Chaudhary N. Understanding the role of free radicals and antioxidant enzymes in human diseases. Curr Pharm Biotechnol. 2022;24(10):1265–76. doi:10.2174/1389201024666221121160822. [Google Scholar] [PubMed] [CrossRef]
88. Li R, Tao R, Ling N, Chemical Chu G. Organic and bio-fertilizer management practices effect on soil physicochemical property and antagonistic bacteria abundance of a cotton field: implications for soil biological quality. Soil Tillage Res. 2017;167(1–3):30–8. doi:10.1016/J.STILL.2016.11.001. [Google Scholar] [CrossRef]
89. Sun X, Atiyeh HK, Li M, Chen Y. Biochar facilitated bioprocessing and biorefinery for productions of biofuel and chemicals: a review. Bioresour Technol. 2020;295:122252. doi:10.1016/J.BIORTECH.2019.122252. [Google Scholar] [PubMed] [CrossRef]
90. Fernández-López J, Viuda-Martos M, Sayas-Barberá ME, de Vera CNR, Lucas-González R, Roldán-Verdú A, et al. Chia, quinoa, and their coproducts as potential antioxidants for the meat industry. Plants. 2020;9(10):1–21. doi:10.3390/plants9101359. [Google Scholar] [PubMed] [CrossRef]
91. López-Escudero FJ, Mercado-Blanco J. Verticillium wilt of olive: a case study to implement an integrated strategy to control a soil-borne pathogen. Plant Soil. 2011;344:1–50. doi:10.1007/S11104-010-0629-2/FIGURES/10. [Google Scholar] [CrossRef]
92. Shreejana KC, Poudel A, Oli D, Ghimire S, Angon PB, Islam MS. A comprehensive assessment of verticillium wilt of potato: present status and future prospective. Int J Phytopathol. 2023;12(2):207–19. doi:10.33687/phytopath.012.02.4647. [Google Scholar] [CrossRef]
93. Elkelish A, Ibrahim MFM, Ashour H, Bondok A, Mukherjee S, Aftab T, et al. Exogenous application of nitric oxide mitigates water stress and reduces natural viral disease incidence of tomato plants subjected to deficit irrigation. Agron. 2021;11(1):87. doi:10.3390/AGRONOMY11010087. [Google Scholar] [CrossRef]
94. Fontana JE, Wang G, Sun R, Xue H, Li Q, Liu J, et al. Impact of potassium deficiency on cotton growth, development and potential microrna-mediated mechanism. Plant Physiol Biochem. 2020;153:72–80. doi:10.1016/J.PLAPHY.2020.05.006. [Google Scholar] [PubMed] [CrossRef]
95. Sharma J, Dua VK, Sharma S, Choudhary AK, Kumar P, Sharma A. Role of plant nutrition in disease development and management. Sustain Manag Potato Pests Dis. 2022;10:83–110. doi:10.1007/978-981-16-7695-6_4. [Google Scholar] [CrossRef]
Cite This Article
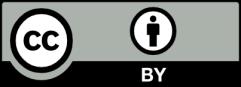
This work is licensed under a Creative Commons Attribution 4.0 International License , which permits unrestricted use, distribution, and reproduction in any medium, provided the original work is properly cited.