Open Access
ARTICLE
Drought Stress Alleviation in Chenopodium quinoa through Synergistic Effect of Silicon and Molybdenum via Triggering of SNF1-Associated Protein Kinase 2 Signaling Mechanism
1 Department of Botany, Abdul Wali Khan University Mardan, Mardan, 23200, Pakistan
2 Department of Biotechnology, Abdul Wali Khan University Mardan, Mardan, 23200, Pakistan
3 Department of Food Science and Nutrition, Dong-A University, Pusan, 602760, Republic of Korea
4 Department of Horticulture and Life Science, Yeungnam University, Gyeongsan, 38541, Republic of Korea
5 Department of Zoology, College of Science, King Saud University, Riyadh, 11451, Saudi Arabia
6 Department of Botany, Government College Women University, Sialkot, 51310, Pakistan
7 Korean Institute of Science and Technology, Gangneung, 25451, Republic of Korea
* Corresponding Authors: Sajid Ali. Email: ; Muhammad Hamayun. Email:
# Two authors equally contributed to this work
(This article belongs to the Special Issue: Abiotic Stress Impacts on Plant Physiology and Their Alleviation)
Phyton-International Journal of Experimental Botany 2024, 93(9), 2455-2478. https://doi.org/10.32604/phyton.2024.054508
Received 30 May 2024; Accepted 06 August 2024; Issue published 30 September 2024
Abstract
Drought stress negatively impacts agricultural crop yields. By using mineral fertilizers and chemical regulators to encourage plant development and growth, its impact can be mitigated. The current study revealed that exogenous silicon (Si) (potassium silicate; K2Si2O5 at 1000 ppm) and molybdenum (Mo) (ammonium molybdate; (NH4)6Mo7O24•4H2O at 100 ppm) improved drought tolerance in quinoa (Chenopodium quinoa Willd). The research was conducted in a randomized complete block design with three biological replicates. The treatments comprised T0 (control, water spray), T4 (drought stress), and T1, T2, T3, T5, T6, and T7, i.e., foliar applications of silicon and molybdenum solutions individually and in combination. Results revealed that drought stress predominantly affected the quinoa yield by decreasing the growth, physiological, biochemical, metabolic, hormonal, antioxidant, and ionic attributes. On the contrary, the supplementation of Si and Mo enhanced the growth attributes (shoot, panicle, and root length, No. of leaves per plant, shoot and panicle fresh/dry weight, root fresh/dry weight, No. of seeds and seeds fresh weight per plant), physiological traits (relative water content, chlorophyll, and carotenoids content), biochemical characteristics (total soluble sugars, protein and lipid content), metabolic attributes (total phenolic, flavonoids, tannins, lycopene, carotene), hormonal contents (indoleacetic acid (IAA), gibberellic acid (GA), salicylic acid (SA)), enzymatic and non-enzymatic antioxidants (catalase, peroxidase and ascorbic acid), and ionic content (potassium (K), (calcium) Ca, (magnesium) Mg, Si and Mo). Under drought stress, Si and Mo reduced electrolyte leakage, abscisic acid (ABA) content, H2O2 production, and sodium uptake. In addition, combined Si and Mo supplementation elevated the expression of the sucrose non-fermenting 1 (SNF1)-associated protein kinase 2 (SnRK2) (CqSNRK2.10) gene in quinoa under drought stress vs. control, signifying an essential regulatory function for Si and Mo-induced drought stress tolerance. These results imply that the exogenous administration of Si and Mo in combination might be an efficient method to alleviate drought stress on quinoa.Keywords
Being sessile organisms, plants are susceptible to several environmental stressors, including low temperatures, salinity, as well as drought [1]. Stress-tolerant plant varieties adapt physiologically, morphologically, biochemically, and molecularly to control growth and performance during drought, affecting 21% of the world’s land area [2,3]. Drought impacts agricultural output and plant growth, necessitating stress-reduction strategies like hyperactivating Reactive Oxygen Species (ROS) scavenging machinery, increasing antioxidant enzyme activities, and activating stress-tolerant genotypes to prevent cell damage [4].
The xerophytic plants can withstand drought by using strategies like (i) osmolyte biosynthesis and accumulation to regulate turgor pressure and prevent structural membrane damage, (ii) regularizing ionic equilibrium and balancing, (iii) modulating water use efficacy and uptake, (iv) elevated photosynthetic activity, (v) biosynthesis and signaling of phytohormones, (vi) metabolic production related to the growth-promotion and stress-mitigation, (vii) genetic adaptation via modulating the expression of genes that drive stress perception and cell signaling, as well as initiate the expression of genes for metabolite and hormone accumulation, for mitigation of drought stress. Drought stress negatively impacts quinoa growth, development, and yield due to insufficient adaptations in stress-prone plants, indicating that not all plant species can fully handle drought [5].
Quinoa, a genetically diverse crop with strong nutritional capacity, is recognized by the FAO for its potential to ensure human food security in the 21st century. Quinoa is a staple in the human diet due to its high nutritional value, often used as a substitute for rice in various foods [6,7]. As reported earlier [8], drought stress (8% VWC) elevated H2O2 and MDA levels in quinoa while significantly reducing plant growth and relative water content, it is still superior to other environmental factors. Researchers are focusing on exploring the key regulatory genes controlling morpho-physiological changes in quinoa to improve drought resistance. High-quality genome sequencing may help explore molecular underpinnings and enhance plant growth features, despite the lack of research on drought-responsive processes in other crops. For example, LOC110738152 and LOC110713661 have been revealed as master genes controlling the drought tolerance responses in quinoa [9]. Another study found that CqZF-HD14, a crucial gene regulating drought tolerance, interacted with CqNAC79 or CqHIPP34 to ameliorate the drought stress resistance of quinoa seedlings [10]. Furthermore, the sucrose non-fermenting 1 (SNF1)-associated protein kinase 2 (SnRK2) gene plays essential roles in biotic and abiotic stresses, which is a member of a small family of plant-specific serine/threonine (Ser/Thr) protein kinases. CqSnRK2.12 overexpression in A. thaliana exhibited drought resistance in comparison to the controls [11].
Scientists are striving to develop various biological and non-biological strategies to enhance drought-stress resistance in quinoa. Recently, the induction of drought tolerance response was reported by rhizobacterial inoculation [12], and biochar application in quinoa [13]. Concerning the micronutrients, sodium silicate application for seed priming was reported [14] in improving wheat growth under drought stress. However, there is a lack of comprehensive studies on the impact of micronutrients on drought stress responses in quinoa. Silicon in soil enhances crop quality, production, growth, photosynthesis, nitrogen fixation, and resistance to drought stress in Si-accumulating plants like rice, wheat, maize, sorghum, oilseed rape, lentil, mango, and tomato [15,16]. However, the bioavailable form of Si (silicic acid) is frequently constrained, as plants absorb silicic acid as a sole silicon source. The exogenous foliar Si supplementation has been shown to reduce drought effects on wheat, pearl millet, and chestnut [15].
Molybdenum is another favorable element that has a key role in increasing tolerance to environmental problems such as drought, cold, and salt stress, according to previous reports [17,18]. Foliar molybdenum application elevated the yield and agronomic characteristics of wheat [19], supported the defense system in Ricinus communis by upregulating the expression of enzymes involved in metabolic processes [20,21], and stimulated the growth, yield, and defense system mechanisms of the mung bean [22].
Therefore, the current study was rationalized to explore the potential of silicon and molybdenum as foliar fertilizers to alleviate drought stress in quinoa, its capacity to withstand stress, and its effects on metabolic, antioxidant, phytohormonal, and mineral status rebalancing.
2.1 Seed Collection and Plant Growth Experiment
Mature seeds of quinoa (Chenopodium quinoa Willd.) variety Q9, were collected from Agriculture University Faisalabad and stored at 5°C. The experiment in the field was done at the Botanical Garden of Abdul Wali Khan University Mardan. Laboratory work was done at the Molecular Biology and Plant Physiology lab, Abdul Wali Khan University Mardan. Soil for plant growth was collected from the Mardan district of Khyber Pakhtunkhwa (KPK) Pakistan, for physicochemical analysis. The soil composition was comprised of sand content (72% to 75%), silt content (10% to 12%), clay (12%–15%), soil pH (7.2 to 7.9), electrical conductivity (0.5% to 5%), organic carbon (4.17%), organic matter (1.3%), carbonates (1.29 meq/L), bicarbonates (2.9 meq/L), and Cl− (1.16 meq/L).
In present work, the silicon (potassium silicate; K2Si2O5, CAS No. 1312-76-1, Brenntag GmbH, Messeallee 11, 45131, Essen, Germany) at the concentration of 1000 ppm, and molybdenum (ammonium molybdate; (NH4)6Mo7O24•4H2O, ammonium molybdate (CAS No. 12054-85-2, Sigma Aldrich, Milwaukee, WI, USA) at the concentration of 100 ppm, was supplemented as foliar fertilization.
The healthy seeds of quinoa were surface sterilized by soaking them in 70% ethanol for 1 minute, followed by three washes with distilled water. After 15 days of germination, the evenly developing seedlings were picked and trimmed out to five per container. Every day, the plants were watered with tap water. Sandy loam soil was placed into each earthen pot, each measuring 30 cm × 12 cm × 12 cm (height, length, breadth). The experimental design followed a completely randomized design (CRD), with 48 pots divided into 8 groups representing different treatments, with at least ten technical replicates and three biological replicates each.
The seedlings were subjected to drought stress after germination for three weeks. After the onset of the first signs of wilting, watering and foliar applications of silicon and molybdenum were performed for each plant in the 4th, 6th, and 10th weeks after seed germination. To ensure leaf absorption, the foliar spray was applied in the morning to both sides of the leaves.
Samples were taken after the development phase for biochemical examination. Merck (Darmstadt, Germany), Sigma-Aldrich (Taufkirchen, Germany), Brenntag GmbH, and Fluka (Buchs, Switzerland) provided the chemicals and reagents for the experiment.
The experimental setup included the following treatments:
Treatment 0: Control (Distilled water).
Treatment 1: Silicon (Potassium silicate 1000 ppm) foliar spray.
Treatment 2: Molybdenum (Ammonium molybdate 100 ppm) foliar spray.
Treatment 3: Silicon + Molybdenum foliar spray.
Treatment 4: Drought stress.
Treatment 5: Drought + Silicon (Potassium silicate 1000 ppm) foliar spray.
Treatment 6: Drought + Molybdenum (Ammonium molybdate 100 ppm) foliar spray.
Treatment 7: Drought + Silicon + Molybdenum foliar spray.
2.2 Monitoring Growth Parameters
For monitoring growth parameters, various agronomic characteristics were studied. The growth parameters included vegetative measurements such as plant root and shoot length, total number of intact leaves, and dry and fresh weight of roots, stems, and leaves. Reproductive attributes like panicle length, fresh and dry mass of the panicle, number of seeds per plant, weight of seeds per plant, and 1000 seeds weight were also measured.
Electrolyte leakage (EL) was measured according to the method described earlier [23] with some modifications. The relative water content (RWC) of fresh leaf samples was quantified following the method described earlier [24]. Leaf water loss (LWL) was measured according to the method mentioned previously [25].
Biochemical analysis was conducted to measure various parameters using standard protocols.
2.4.1 Extraction and Estimation of Photosynthetic Pigments
The estimation of chlorophyll a, b, total chlorophyll, and carotenoid content in fresh leaf samples (1.0 g) from the 15th leaf (counted from the base) of each plant was performed following the process described earlier [26].
2.4.2 Analysis of Metabolites and Phytohormones
Metabolic evaluation was carried out using fresh leaf samples from the 15th leaf (counted from the base) of each plant. Total soluble sugars were quantified by the method indicated earlier [27]. The leaves’ total protein content was quantified according to the method mentioned earlier [28]. Lipid content was quantified as described earlier [29]. The proline quantification was conducted as described earlier [30]. Total phenolics were assessed as explained previously [31]. Lycopene and β-carotene were assessed using the method described earlier [32]. Tannin was measured using the method described earlier [33]. The total flavonoid content was calculated using the AlCl3 technique reported earlier [34]. The estimation of auxin content was carried out using the Salkowski reagent [35], as described previously [36]. Salicylic acid (SA) content was evaluated as mentioned earlier [30]. Endogenous ascorbic acid (AsA) was quantified by the method mentioned earlier [37]. The content of GA3 and ABA content was investigated by the method mentioned previously [38].
2.4.3 Quantification of Enzymatic and Non-Enzymatic Antioxidants
The total antioxidant capacity was quantified using the method devised earlier [39]. Catalase activity was quantified as described [40]. Guaiacol peroxidase activity was quantified according to the procedure described [41]. Ascorbate peroxidase activity was quantified following the method described earlier [42]. The determination of H2O2 was performed according to the method mentioned earlier [31]. The detection of ROS was done using the procedure outlined earlier [43].
2.4.4 Detection of Reactive Oxygen Species (ROS) Accumulation through DAB Activity Assay
Detection of ROS through 3,3-diaminobenidine (DAB) staining was performed as described earlier [44].
Samples of dried ground plants were measured for the concentrations of several elements (Ca2+, Mg2+, K+, and Na+). A small amount of 0.5 g of pulverized, dry plant material was powdered and put into digesting vials. Each sample received 6.5 mL of a 5:1:0.5 ratio acid solution (HNO3, H2SO4, HClO4) for the digestion procedure. For dilution, after adding distilled water, the sample was filtered using No. 1 Whatman filter paper, as described earlier [45]. Following the steps outlined previously [46]. The modified molybdenum blue technique was used to measure silicon uptake. The extraction-photometric approach utilizing Tetrazolium Violet (TV) was used to determine the molybdenum content, as mentioned earlier [47].
2.6 RT-qPCR Analysis for Expression of Drought Stress Marker Gene CqSnRK2.10
Total RNA was isolated from quinoa leaves and root tissues, followed by DNase treatment to get purified stable RNA that was subsequently used for cDNA synthesis, following the procedure outlined previously [44]. The gene expression analysis of drought stress-related marker gene, CqSnRK2.10, reported earlier [11], was performed using qPCR primers designed by Primer3 [48], with oligonucleotide sequences of CqSnRK2.10 gene as F-AAGCCCTCGTTCGGATACTTAATGC, and R-GCCTCTTGTTCCACCACTAATCTTCTC.
Due to the stable expression under drought stress in quinoa, the internal control CqTUB-9 was used with primer sequences; F-GAGATGTTCCGTCGTGTGAGTGAG, and R-ATCGGCAGTTGCATCCTGGTATTG, as reported earlier [11]. Oligonucleotides for primers were synthesized by Bio Basic (Seoul, Republic of Korea). Gene expression analysis and data normalization procedure was followed as previously mentioned earlier [44].
Biological triplicates were used in the studies. Data analysis was carried out using a two-way ANOVA. The SPSS 20 (SPSS Inc., Chicago, IL, USA) was utilized to conduct the Duncan multiple range test (DMRT) for the differential comparison of mean values. Different statistical bars marked with significant letters at p < 0.05 were used to display significant differences. Fold changes were calculated as described earlier [29].
3.1 Effect of Silicon and Molybdenum on Vegetative Growth Attributes of Quinoa under Drought Stress
The findings of this study demonstrate that drought stress exerted a negative impact on the growth of quinoa. The quantitative data for the growth potential of quinoa under drought stress exhibited a statistically significant decrease in shoot length cm [42/57 cm (control)], root length [8.3/17 cm (control)], number of leaves [26/40 (control)], comparable to the respective control plants. Nevertheless, under drought stress the silicon and molybdenum supplementation promoted growth comparable to the respective control plants.
Silicon and molybdenum combined supplementation exhibited statistically significant promotion in shoot length, [54/42 cm (drought control)], root length [(15/8.3 cm (DC), number of leaves [37/26 (drought control)], in quinoa under drought stress, comparable to the respective control plants (Fig. 1A–D).
Figure 1: Foliar application of silicon and molybdenum on growth phenotype of quinoa under drought stress. Phenotypic analysis (A) shoot length (B) root length (C) and No. of leaves/plant (D). Numeric data is represented by means and standard errors of three biological replicates with at least four technical replicates, marked with letters denoting significant differences at p ≤ 0.05
The quantitative data for the growth performance of quinoa under drought stress showed statistically significant reduction in shoot fresh weight [5.4/10 g (control)], shoot dry weight [1.5/2.9 g (control)], leaves fresh weight per plants [1.6/2.9 g (control)], leaves dry weight per plants [0.29/0.7 g (control)], root fresh weight [1.37/2.35 g (control)], root dry weight [0.37/0.87 g (control)], total fresh weight [8.4/15 g (control)] and total dry weight [2.08/4.5 g (control)], comparable to the respective control plants.
Silicon and molybdenum combined supplementation exhibited statistically significant promotion in shoot fresh weight [8.5/5.4 g (drought control)], shoot dry weight [2.7/1.5 g (drought control)], leaves fresh weight per plant [2.68/1.6 g (drought control)] leaves dry weight per plant [0.58/0.29 g (drought control)], root fresh weight [0.75/0.34 g (DC), root dry weight [0.79/0.37 g (drought control)], total fresh weight [13/8.4 g (drought control)] and total dry weight [4/2.08 g (drought control)], in quinoa plants under drought stress, comparable to the respective control plants (Fig. 2A–H).
Figure 2: The impact of foliar application of silicon and molybdenum on vegetative growth attributes of quinoa under drought stress. Shoot fresh weight (A) shoot dry weight (B) leaves fresh weight (C) leaves dry weight (D) root fresh weight (E) root dry weight (F) total fresh weight (G) and total dry weight (H). Numeric data is represented by means and standard errors of three biological replicates with at least four technical replicates, marked with letters denoting significant differences at p ≤ 0.05
3.2 Effect of Silicon and Molybdenum on Reproductive Growth Attributes of Quinoa under Drought Stress
The findings of the present research exposed that drought stress negatively affects the reproductive growth of quinoa. Parameters such as panicle length, fresh and dry biomass of the panicle, number of seeds per plant, seed weight per plant, and 1000 seeds weight were negatively affected.
Quantitative results for the reproductive growth attributes in quinoa under drought stress showed a statistically significant reduction in panicle length [11.3/23.5 cm (control)], fresh biomass of the panicle [6/17 g (control)], dry biomass of panicle [1.6/3.6 g (control)], number of seeds per plant [620/1001 (control)], total seed weight per plant [1.7/3.8 g (control)], and 1000 seeds weight [2.6/3.8 g (control)], as compared to control (C) without stress.
Silicon and molybdenum combined supplementation exhibited statistically significant promotion in panicle length [22/11.3 cm (drought control)], fresh biomass of the panicle [12/6 g (drought control)], dry biomass of panicle [3/1.6 g (drought control)], number of seeds per plant [900/620 (drought control)], total seed weight per plant [1.7/3.8 g (drought control)], and 1000 seeds weight [3.7/2.6 g (drought control)], in quinoa plants under drought stress, comparable to the respective control plants (Fig. 3A–G).
Figure 3: Foliar spray effect of silicon and molybdenum on reproductive growth attributes of quinoa under drought stress. Panicle length (A) panicle fresh mass (B) panicle dry weight (C) number of seeds/plants (D) seed weight/plant (E) 1000 seeds weight (F) and visual phenotype of seed mass/plant (G). Numeric data is represented by means and standard errors of three biological replicates with at least four technical replicates marked with letters denoting significant differences at p ≤ 0.05
3.3 Effect of Silicon and Molybdenum on Physiological Attributes of Quinoa under Drought Stress
In quinoa under drought stress, the quantitative results exposed a statistically significant reduction in relative water content [49/73% (control)], and leaf water loss [32/54% (control)], compared to control (C) without stress, with elevated electrolyte leakage [27/15% (control)].
Nevertheless, silicon and molybdenum combined supplementation exhibited statistically significant promotion in relative water content [75/49% (drought control)], and leaf water loss [53/32% (drought control)] with a decline in electrolyte leakage [20/27% (drought control)] in quinoa plants under drought stress, comparable to the respective control plants (Fig. 4A–C).
Figure 4: Foliar spray effect of silicon and molybdenum on physiological attributes and photosynthetic pigments content of quinoa under drought stress. Relative water content (A) leaf water loss (B) electrolytes leakage (C) chlorophyll a (D) chlorophyll b (E) total chlorophyll (F) chlorophyll a/b (G) and total carotenoids (H). Numeric data is represented by means and standard errors of three biological replicates with at least four technical replicates, marked with letters denoting significant differences at p ≤ 0.05
3.4 Effect of Silicon and Molybdenum on Biochemical Attributes of Quinoa under Drought Stress
Under drought stress, the quantitative results exposed statistically significant reduction in the chlorophyll a [1.4/4.6 (mg/g FW) (control)], chlorophyll b [0.9/2.3 (mg/g FW) (control)], total chlorophyll [2.3/7.1 (mg/g FW) (control)], chlorophyll a/b ratio [1.4/1.9 (control)], and carotenoid content [0.3/1.3 (mg/g FW) (control)], compared to control (C) without stress.
Silicon and molybdenum combined supplementation exhibited statistically significant promotion in chlorophyll a [3.9/1.4 (mg/g FW) (drought control)], chlorophyll b [2.1/0.9 (mg/g FW) (drought control)], total chlorophyll [6.1/2.3/(drought control)], chlorophyll a/b in quinoa plants under drought stress, comparable to the respective control plants (Fig. 4D–H).
3.4.2 Protein, Lipid and Soluble Sugars Content
Under drought stress, the quantitative results exposed statistically significant promotion in the content of total protein [51/32 (mg/g FW) (control)], proline [0.9/0.3 (mg/mL) (control)], lipids [0.2/0.9 (mg/g FW) (control)], and total soluble sugars [32/72 (mg/g FW) (control)], compared to control (C) without stress.
Silicon, and molybdenum combined supplementation exhibited a statistically significant further promotion in the total protein [73/51 (mg/g FW) (drought control)], proline [1.2/0.3 (mg/mL) (drought control)], lipids [0.5/0.2 (mg/g FW) (drought control)], and total soluble sugars content [43/32 (mg/g FW) (drought control)], in quinoa plants under drought stress, comparable to the respective control plants (Fig. 5A–E).
Figure 5: Foliar spray effect of silicon and molybdenum on biochemical attributes of quinoa under drought stress. Proline (A) total proteins (B) total lipids (C) total soluble sugar (D) total phenols (E) flavonoids content (F) tannin (G) lycopene (H) and β-carotene (I). Numeric data is represented by means and standard errors of three biological replicates with at least four technical replicates, marked with letters denoting significant differences at p ≤ 0.05
3.4.3 Total Phenolics and Flavonoids Content
Under drought stress, the quantitative results exposed statistically significant promotion in the total phenolics [32/17 (mg/g FW) (control)], and total flavonoids [0.5/0.36 mg (mg/mL) (control)], compared to control (C) without stress.
Silicon and molybdenum combined supplementation exhibited a statistically significant further promotion in the total phenolics [43/32 (mg/g FW) (drought control)], and total flavonoids [0.86/0.5 (mg/mL) (drought control)], in quinoa plants under drought stress, comparable to the respective control plants (Fig. 5F–G).
3.4.4 Tannin, Lycopene, and β-Carotene Content
Under drought stress, the quantitative results exposed statistically significant promotion in the tannins [41/30 (mg/mL) (control)], lycopene [0.2/0.1 (mg/mL) (control)], and beta-carotene [0.2/0.1 (mg/g FW) (control)], compared to control (C) without stress.
Silicon, and molybdenum combined supplementation exhibited a statistically significant further promotion in tannins [57/30 (mg/mL) (drought control)], lycopene [0.4/0.2 (mg/mL) (drought control)], and beta-carotene [0.4/0.1 (mg/g FW) (drought control)], in quinoa plants under drought stress, comparable to the respective control plants (Fig. 5G–I).
3.5 Effect of Silicon and Molybdenum on Hormonal Content of Quinoa under Drought Stress
Under drought stress, the quantitative results exposed a statistically significant decrease in the IAA content [0.5/2.1 (mg/mL) (control)], GA3 content [12/22 (mg/mL) (control)], and SA content [36/57 (mg/g FW) (control)], compared to control (C) without stress. However, silicon and molybdenum combined supplementation exhibited a statistically significant promotion in IAA content [1.5/0.5 (mg/mL) (drought control)], GA3 content [25/22 (mg/mL) (drought control)], and SA content [58/57 (mg/g FW) (control)], in quinoa plants under drought stress, comparable to the respective control plants (Fig. 6A–C).
Figure 6: Foliar spray effect of silicon and molybdenum on metabolic content of quinoa under drought stress. IAA content (A) GA3 content (B) salicylic acid content (C) and ABA content (D). Numeric data is represented by means and standard errors of three biological replicates with at least four technical replicates, marked with letters denoting significant differences at p ≤ 0.05
The quantitative results exposed statistically significant promotion in the ABA content [1.3/0.4 (mg/mL) (control)], compared to control (C). However, silicon and molybdenum combined supplementation exhibited a statistically significant further promotion in ABA content [0.5/1.3 (mg/mL) (drought control)], in quinoa plants under drought stress, comparable to the respective control plants (Fig. 6D–I).
3.6 Effect of Silicon and Molybdenum on Antioxidant Capacity and ROS Status of Quinoa under Drought Stress
Under drought stress, ROS production was studied for assessment of oxidative burst in quinoa upon silicon and molybdenum application. To this end, the quantity of H2O2 was detected as brown spots in the leaves of the plant using DAB staining in the leaf tissues of quinoa (Fig. 7A).
Figure 7: Foliar spray effect of silicon and molybdenum on ROS accumulation and antioxidant capacity of quinoa under drought stress. Endogenous ROS accumulation (A) H2O2 content (B) ascorbate peroxidase (C) guaiacol peroxidase (D) catalase (E) ascorbic acid content (F) and total antioxidant (G). Numeric data is represented by means and standard errors of three biological replicates with at least four technical replicates, marked with letters denoting significant differences at p ≤ 0.05
The highest quantity of H2O2 accumulation was recorded in drought-treated plant tissues [48/27 nmol g−1 fresh weight (control)], compared to control (C) without stress (Fig. 7B). However, under drought stress, silicon, and molybdenum combined supplementation exhibited a significantly (p ≤ 0.05) decreased amount of H2O2 tissues [34/48 nmol g−1 fresh weight (drought control)], and intensity of the DAB staining, in quinoa that has been stressed by drought, comparable to the respective control plants (Fig. 7A, B).
Antioxidants (both non-enzymatic and enzymatic) were investigated, in quinoa with separate and collective supplementation of silicon and molybdenum under drought conditions. Fig. 7C–F depicts the differential responses seen under various treatments. The level of enzymatic antioxidants (Ascorbate peroxidase enzyme, Guaiacol peroxidase, Catalase), and non-enzymatic antioxidants (ascorbic acid) was predominantly elevated both under normal and drought stress conditions upon supplementation, both individually and in combination, of silicon and molybdenum compared to control.
Under drought stress, the quantitative results exposed statistically significant promotion in the Ascorbate peroxidase enzyme [75/27 (mg/g FW) (control)], compared to control (C) without stress. Nevertheless, under drought stress, silicon and molybdenum combined supplementation exhibited a significant (p ≤ 0.05) further promotion in ascorbate peroxidase enzyme [121/75 (mg/g FW) (drought control)] in quinoa under drought stress, comparable to the respective control plants (Fig. 7C). Under drought stress, the quantitative results exposed statistically significant promotion in the Guaiacol peroxidase enzyme [181/108 (mg/g FW) (control)], compared to control (C) without stress. Nevertheless, silicon and molybdenum combined supplementation exhibited a significant (p ≤ 0.05) further promotion in Guaiacol peroxidase enzyme [205/181 (mg/g FW) (drought control)] in quinoa under drought stress, comparable to the respective control plants (Fig. 7D).
Under drought stress, the quantitative results exposed statistically significant promotion in the catalase enzyme [107/71 (mg/g FW) (control)], compared to control (C) without stress. Nevertheless, silicon and molybdenum combined supplementation exhibited a statistically significant further promotion in catalase enzyme [128/107 (mg/g FW) (drought control)] in quinoa under drought stress, comparable to the respective control plants (Fig. 7E). Under drought stress, the quantitative results exposed statistically significant promotion in the ASA content [0.68/0.51 (mg/g FW) (control)], compared to control (C) without stress. Nevertheless, silicon and molybdenum combined supplementation exhibited a significant (p ≤ 0.05) further promotion in ASA content [0.85/0.68 (mg/g FW) (drought control)] in quinoa under drought stress, comparable to the respective control plants (Fig. 7F).
Under drought stress, the quantitative results exposed a statistically significant promotion in the Total antioxidants [0.35/0.27 (mg/g FW) (control)], compared to control (C) without stress. Silicon and molybdenum combined supplementation exhibited a significant (p ≤ 0.05) further promotion in total antioxidants [0.5/0.35 (mg/g FW) (drought control)] in quinoa under drought stress, comparable to the respective control plants (Fig. 7G).
3.7 Effect of Silicon and Molybdenum on Mineral Status of Quinoa under Drought Stress
During the current investigation, it was found that drought stress caused a significant (p < 0.05) over-accumulation of Na+, and Ca2+, and concentration and a reduction of K+, and Mg2+ in quinoa compared to control. Under drought stress, the quantitative results exposed a statistically significant promotion in the Na+ content [9.4/5.7 (mg/g FW) (control)], compared to control (C) without stress. Nevertheless, under drought stress, silicon and molybdenum combined supplementation exhibited a significantly (p ≤ 0.05) decreased Na+ content [6.8/9.4 (mg/g FW) (drought control)] in quinoa under drought stress, comparable to the respective control plants (Fig. 8A).
Figure 8: Foliar spray effect of silicon and molybdenum on ionic concentration of Chenopodium quinoa under drought stress. Sodium content (A) potassium content (B) calcium content (C) magnesium content (D) molybdenum content (E) and silicon content (F). Numeric data is represented by means and standard errors of three biological replicates with at least four technical replicates, marked with letters denoting significant differences at p ≤ 0.05
Under drought stress, the quantitative results exposed a statistically significant decrease in the K+ content [523/710 (mg/g FW) (control)], compared to control (C) without stress. Silicon and molybdenum combined supplementation exhibited a significantly (p ≤ 0.05) elevated K+ content [697/523 (mg/g FW) (drought control)] in quinoa under drought stress, comparable to the respective control plants (Fig. 8B). Under drought stress, the quantitative results exposed a statistically significant promotion in the Ca2+ content [304/257 (mg/g FW) (control)], compared to control (C) without stress. Silicon and molybdenum combined supplementation exhibited a significantly (p ≤ 0.05) elevated Ca2+ content [697/523 (mg/g FW) (drought control)] in quinoa under drought stress, comparable to the respective control plants (Fig. 8C). Under drought stress, the quantitative results exposed a statistically significant promotion in the Mg2+ content [99/146 (mg/g FW) (control)], compared to control (C) without stress. Silicon and molybdenum combined supplementation exhibited a significantly (p ≤ 0.05) elevated Mg2+ content [123/94 (mg/g FW) (drought control)] in quinoa under drought stress, comparable to the respective control plants (Fig. 8D–F).
3.8 Quantitative Gene Expression Analysis of CqSnRK2.10 under Drought Stress
To measure the level of expression of selected drought stress marker genes, the RT-qPCR method was used for evaluating the induction of sucrose non-fermenting1 (SNF1)-associated protein kinase 2 (SnRK2.10), which belongs to a comparatively small plant-specific family of serine/threonine (Ser/Thr) protein kinases [11]. In the present study, gene expression analysis for the CqSnRK2.10, in the leaf tissues of quinoa grown under drought revealed a statistically significant promotion in CqSnRK2.10 expression [(3.8-fold/1.9-fold (control)]. Under drought stress, silicon, and molybdenum combined supplementation resulted in a further promotion in CqSnRK2.10 expression [(8.5-fold/3.8-fold (drought control)] in leaf tissue.
Moreover, gene expression analysis for the CqSnRK2.10, in the root tissues of quinoa grown under drought, revealed a statistically significant promotion in the expression [(3.5-fold/2.1-fold (control)]. However, under drought stress, silicon and molybdenum combined supplementation resulted in a further promotion in expression [(10-fold/3.5-fold (drought control)] in root tissue (Fig. 9).
Figure 9: Expression profiling of CqSNRK2.10 gene measured by RT-qPCR using leaf and root tissue of quinoa foliar supplemented with silicon and molybdenum, under drought stress. Numeric data is represented by means and standard errors of three biological replicates with at least four technical replicates, marked with letters denoting significant differences at p ≤ 0.05
Water scarcity is a critical factor that prevents plants from growing, especially during the vegetative and reproductive phases of plant growth of susceptible plants such as quinoa. However, the exploitation of plant drought stress tolerance using the exogenous application of drought stress tolerance mediators and micronutrients, silicon (Si) and molybdenum (Mo) has shown restoration of the sustainable growth of quinoa under drought stress in the present study. Plant nutrition plays an important function in maintaining healthy growth and augmenting stress tolerance. Micronutrients, such as Si and Mo, have been shown to provide tolerance to plants against various stresses [22].
Under drought stress, Si application provides plant tolerance through various mechanisms. Si application can up-regulate aquaporin genes (PIP) and mitigate ROS-induced aquaporin activity inhibition in plants. By raising the concentration of soluble carbohydrates and amino acids contents in the xylem sap, which increases osmotic potential, or by triggering K+ transport to the xylem sap via the SKOR gene impacting the osmoregulation [49]. Another trace element that is present in soil, and is essential for plant growth is molybdenum (Mo). It exists in different oxidation states, with the most prevalent form found in agricultural soils being Mo (VI). The primary form of molybdenum that is accessible to plants is molybdate. Despite having a major impact on several redox processes, molybdenum is only necessary in very small amounts when compared to other vital micronutrients [50]. Molybdenum is utilized by specific plant enzymes as part of the molybdenum co-factor to participate in oxidation-reduction reactions. Mo is required for the activity of more than 50 enzymes, including five found in plants, involved in nitrogen, sulfur, purine, and phytohormone metabolism [51]. It is part of several enzymes, including nitrate reductase (NR), xanthine dehydrogenase/oxidase (XDH), aldehyde oxidase (AO), and sulphite oxidase (SO), which are involved in nitrogen fixation and assimilation, purine catabolism, phytohormone synthesis, and sulfur metabolism, respectively [52]. Consistently, the present research also showed that under drought stress, individual, as well as combined supplementation of silicon and molybdenum, improved the vegetative and reproductive growth of quinoa in terms of plant length, biomass, seed growth, and yield.
Present results align with previous studies, demonstrating a significant reduction in various growth attributes under drought stress in different plant species, as drought stress disrupts plant metabolism, particularly photosynthetic pigments, by generating excessive reactive oxygen species (ROS) [53]. The decrease in chlorophyll content observed under water shortage is primarily attributed to protein degradation in chloroplast membranes caused by ROS overproduction [54]. Relative water content (RWC) reflects the ability of cell membranes to withstand environmental cues, including drought stress [55].
In the present investigation, comparable to other studies, a decrease in RWC under water shortage conditions is observed. This decline in RWC indicates a loss of turgor, limiting water availability for cell expansion and subsequently suppressing plant development and growth. RWC is considered a reliable indicator of drought stress tolerance in plants. Proline accumulation as a result of water stress has been well-documented as a mechanism to maintain leaf tissue homeostasis in various plant species. In addition to this, the accumulation of sugars serves as a self-protective strategy employed by plants against adverse environmental conditions, and current results also showed a promotion in total soluble sugars and non-reducing sugars under water shortage conditions, in line with the conclusions of previous reports that sugars act as osmoprotectants and confer water stress tolerance in plants. In the present study, drought-stress individuals as well as combined supplementation of silicon and molybdenum, elevated the photosynthetic activity, and relative water content of quinoa grown under drought stress.
It is also known that Si supplementation can improve root hydraulic conductance, modify root growth, increase root/shoot ratio, elevate aquaporin activity, and enhance osmotic driving force. This, in turn, results in elevated water uptake and transport, leading to higher photosynthetic rates and improved plant resistance to water deficiency [56]. Si application can also alter gas exchange attributes in plants enhance antioxidant defense by increasing the biochemical activities, and protect seedlings from oxidative damage. Furthermore, Si In several crop species, application under water-scarce circumstances boosts photosynthetic rate, leaf and root water and osmotic potential, and water usage efficiency, while lowering transpiration activity and membrane trafficking [57]. Molybdenum (molybdate) is also essential for plant development and plays a vital role in physiological processes [22].
Si has been widely reported to decrease oxidative damage by enhancing the activities of antioxidant enzymes (SOD, APX, CAT, and POD) under drought stress in wheat, sunflower, and tomato plants [58]. Si pretreatment has been shown to up-regulate the expression of drought-specific genes in rice during drought stress [59]. Molybdenum is essential for promoting the production of enzymes including POD, CAT, and SOD as well as raising the proline concentration in cells, thus supporting the plant defense system and playing an important role in mung bean plants under water stress conditions [22]. In the present study, individual as well as combined supplementation of silicon and molybdenum, also elevated the antioxidant capacity of quinoa grown under drought stress, thus reducing ROS production and oxidative damage.
Si and Mo translocation in plant tissues also affect the uptake of mineral ions (Na+, Ca2+, K+, and Mg2+), as it is known that the translocation of one type of micronutrient influences the translocation of others. For example, the translocation of silicon is reported to mitigate the concentration of copper in root and shoot in A. thaliana in a water-based culture. Thus, silicon translocation reduces the excessive copper concentration in plant tissue to reduce metal toxicity. The transport of molybdenum aids in the transportation of the micronutrients in the plant’s vascular tissues and leaf buds [60]. Different mineral elements also play a crucial role in physiological role in plant development and growth. For example, K+ plays a crucial role in water-use efficiency, stomatal control, aerial and underground biomass, and photosynthesis [61]. It also serves as a mineral osmolyte that regulates turgor and osmotic pressure to facilitate plant growth, cell enlargement, and leaf stomata opening and closing. In Carthamus tinctorius, K+ elevated the leaf’s relative moisture content, while magnesium decreased it [62]. The Mg2+ concentration was affected by drought stress, with a decrease observed in plant leaves as drought stress elevated [63,64]. The same outcomes were reported. in tomatoes, where drought led to a decrease in Mg concentration [65]. Mg2+ levels significantly influence chlorophyll content but exert a negative impact on Ca2+ levels in safflower [62]. With the severity of drought stress increasing, Ca2+ concentration decreased, with the lowest concentration being seen in control plants. Similar findings were reported in tomatoes, where water stress reduced Ca2+ concentrations [65]. Ca2+ ions act as Essential alternative messengers are crucial for different phases of plant development and growth and trigger physiological changes due to drought stress.
Additionally, Ca2+ is essential for controlling the development of polar tissues and cells as well as plant stress tolerance. It influences the response of plants to drought stress and ABA-induced stomatal closure in plants [66,63,67]. Na+ concentration elevated with increasing drought stress, with the lowest concentration observed in control plants. The water usage potential of olive leaves altered during drought stress, and elevated K+ and Na+ levels enhanced the starchy content of the leaf. Na+ influenced metabolism and the photosynthetic response favorably when K+ levels were insufficient [63,64]. Ca2+ concentration elevated with the intensity of drought stress, with the lowest concentration observed in control plants Similar findings were reported in tomatoes, where water scarcity triggered a reduction in Ca2+ concentrations [65]. Ca2+ ions serve as significant signaling molecules, and messengers that, in response to the stress of drought, promote physiological mechanisms positively, and are essential for various phases of plant development and growth. Ca2+ plays a significant part in controlling the development of polar tissues and cells as well as plant stress tolerance through adaptability. It affects abscisic acid Ca2+ concentration elevated with the intensity of drought stress, with the lowest concentration observed in control plants Similar findings were reported in tomatoes, where water stress reduced Ca2+ concentrations [65,67]. Ca2+ ions act as important signaling molecules that stimulate physiological functions as a result of drought stress and are essential for various stages of plant development and growth. Ca2+ also plays a key role in regulating polar cell and tissue growth and plant adaptation to stress.
The present study revealed that individual as well as combined supplementation of silicon and molybdenum readjusted the mineral status of quinoa by affecting root-to-shoot transportation. Various transporters have been found in plants up to this point. For instance, several transporters, such as the high-affinity K+/Na+ transporter (HKT) and the K+ transporter (KT), are used to move the ion K+, Ca2+-ATPases, Ca2+/H+ exchanger transports, and transporters Ca2+ permeable ion channels are used to move the calcium (Ca). Magnesium (Mg) is transported throughout the plant system by the magnesium transporter (MGT). Molybdenum (Mo) is transported throughout the plant via the molybdenum transporter type (MOT). According to a review [60], the molybdenum transporter type 1 (MOT1) is more prevalent in plants. Previously, exogenous Si application was up-regulated. Numerous monocot and dicot plant species have been found to contain the Si-transporter aquaporin genes AQPs [68]. In the current study, Si and Mo induced the expression of drought-resistant marker gene (CqSnRK2.10) serine/threonine (Ser/Thr) protein kinases in quinoa grown under drought, implying a crucial role in the regulation of drought stress resistance due to the induction of CqSnRK2.10 expression, triggering the signaling mechanism for readjustment of drought stress tolerance response in quinoa, and the overall findings of present work have been depicted in graphical abstract in Fig. 10.
Figure 10: Graphical presentation showing the role of exogenous application of Si and Mo in quinoa under stress. Drought stress inhibited plant growth, while the combined application of Si and Mo ameliorated the drought stress tolerance and promoted the growth and yield by activating the expression of CqSNRK2.10 (a drought-resistant marker gene), for induction of drought tolerance through metabolic, antioxidant, and ionic reshuffling and rebalancing in quinoa
Current research concludes the prospective significance of individual and combined supplementation of silicon and molybdenum for growth promotion and drought stress alleviation in quinoa. This is achieved by triggering the signaling mechanism driven by the drought-resistant marker gene (CqSnRK2.10) serine/threonine (Ser/Thr) protein kinases. Consequently, silicon and molybdenum supplementation readjusted the physiological, biochemical, antioxidant, hormonal, and mineral status of quinoa plants under drought stress to a level well-suited for optimal growth, development, and yield.
Acknowledgement: We extend our appreciation to the Researchers Supporting Project No. RSP2024R191, King Saud University, Riyadh, Saudi Arabia.
Funding Statement: The authors declare that the current research was supported by the IFS—International Foundation for Science, Stockholm, Sweden (C/5895-1).
Author Contributions: Study conception and design: Humaira Gul, Zahid Ali Butt, and Mamoona Rauf. Data collection and main experiments: Asmat Askar, Mamoona Rauf, and Muhammad Arif. Analysis and interpretation of results: Asmat Askar, Humaira Gul, Mamoona Rauf, and Muhammad Arif. Draft manuscript preparation: Humaira Gul, and Mamoona Rauf. Validation and revision of results and manuscript: Muhammad Arif, Muhammad Hanayun, Sajid Ali, Yoon-Ha Kim, Seong-Hoon Kim, and Abdulwahed Fahad Alrefaei. Financial support for the project: Mamoona Rauf, Seong-Hoon Kim, Mikhlid H. Almutairi, and Abdulwahed Fahad Alrefaei. All authors reviewed the results and approved the final version of the manuscript.
Availability of Data and Materials: The datasets generated during and/or analyzed during the current study are available from the corresponding author upon reasonable request.
Ethics Approval: This article does not contain any studies with human participants or animals by any of the authors.
Conflicts of Interest: All authors declare that they have no conflicts of interest to report regarding the present study.
References
1. Han G, Lu C, Guo J, Qiao Z, Sui N, Qiu N, et al. C2H2 zinc finger proteins: master regulators of abiotic stress responses in plants. Front Plant Sci. 2020;11:115. doi:10.3389/fpls.2020.00115. [Google Scholar] [PubMed] [CrossRef]
2. UN. 2010–2020: UN decade for deserts and the fight against desertification. Why now? United Nations. 2010. Available from: http://www.un.org/en/events/desertification_decade/whynow.shtml. [Accessed 2021]. [Google Scholar]
3. Damberg L, AghaKouchak A. Global trends and patterns of drought from space. Theor Appl Climatol. 2014;117:441–8. doi:10.1007/s00704-013-1019-5. [Google Scholar] [CrossRef]
4. Seleiman MF, Al-Suhaibani N, Ali N, Akmal M, Alotaibi M, Refay Y, et al. Drought stress impacts on plants and different approaches to alleviate its adverse effects. Plants. 2021;10(2):259. doi:10.3390/plants10020259. [Google Scholar] [PubMed] [CrossRef]
5. Geerts S, Raes D, Garcia M, Vacher J, Mamani R, Mendoza J, et al. Introducing deficit irrigation to stabilize yields of quinoa (Chenopodium quinoa Willd.). Eur J Agron. 2008;28(3):427–36. doi:10.1016/j.eja.2007.11.008. [Google Scholar] [CrossRef]
6. Quinoa FAO. An ancient crop to contribute to world food security. Reg Office Latin Am Caribbean. 2011;2:73–87. [Google Scholar]
7. Fiallos-Jurado J, Pollier J, Moses T, Arendt P, Barriga-Medina N, Morillo E, et al. Saponin determination, expression analysis and functional characterization of saponin biosynthetic genes in Chenopodium quinoa leaves. Plant Sci. 2016;250:188–97. doi:10.1016/j.plantsci.2016.05.015. [Google Scholar] [PubMed] [CrossRef]
8. Lin PH, Chao YY. Different drought-tolerant mechanisms in quinoa (Chenopodium quinoa Willd.) and djulis (Chenopodium formosanum Koidz.) based on physiological analysis. Plants. 2021;10(11):2279. doi:10.3390/plants10112279. [Google Scholar] [PubMed] [CrossRef]
9. Huan X, Li L, Liu Y, Kong Z, Liu Y, Wang Q, et al. Integrating transcriptomics and metabolomics to analyze quinoa (Chenopodium quinoa Willd.) responses to drought stress and rewatering. Front Plant Sci. 2022;13:988861. doi:10.3389/fpls.2022.988861. [Google Scholar] [PubMed] [CrossRef]
10. Sun W, Wei J, Wu G, Xu H, Chen Y, Yao M, et al. CqZF-HD14 enhances drought tolerance in quinoa seedlings through interaction with CqHIPP34 and CqNAC79. Plant Sci. 2022;323:111406. doi:10.1016/j.plantsci.2022.111406. [Google Scholar] [PubMed] [CrossRef]
11. Zhu XL, Wang BQ, Wang X, Wei XH. Identification of the CIPK-CBL family gene and functional characterization of CqCIPK14 gene under drought stress in quinoa. BMC Genom. 2022;1:447. [Google Scholar]
12. Aslam MU, Raza MA, Saleem MF, Waqas M, Iqbal R, Ahmad S, et al. Improving strategic growth stage-based drought tolerance in quinoa by rhizobacterial inoculation. Commun Soil Sci Plant Anal. 2020;51(7):853–68. doi:10.1080/00103624.2020.1744634. [Google Scholar] [CrossRef]
13. Kammann CI, Linsel S, Gobling JW, Koyro HW. Influence of biochar on drought tolerance of Chenopodium quinoa Willd and on soil-plant relations. Plant Soil. 2011;345(1–2):195–210. [Google Scholar]
14. Hameed A, Sheikh MA, Jamil A, Basra SM. Seed priming with sodium silicate enhances seed germination and seedling growth in wheat (Triticum aestivum L.) under water deficit stress induced by polyethylene glycol. Pak J Life Soc Sci. 2013;11(1):19–24. [Google Scholar]
15. Ning D, Zhang Y, Li X, Qin A, Huang C, Fu Y, et al. The effects of foliar supplementation of silicon on physiological and biochemical responses of winter wheat to drought stress during different growth stages. Plants. 2023;12(12):2386. doi:10.3390/plants12122386. [Google Scholar] [PubMed] [CrossRef]
16. Liang Y, Nikolic M, Belanger R, Gong H, Song A. Silicon in agriculture. In: From Theory to practice. Dordrecht Heidelberg: Springer; 2015. vol. 3, p. 4. [Google Scholar]
17. Al-Issawi M, Rihan HZ, Woldie WA, Burchett S, Fuller MP. Exogenous application of molybdenum affects the expression of CBF14 and the development of frost tolerance in wheat. Plant Physiol Biochem. 2013;63:77–81. doi:10.1016/j.plaphy.2012.11.010. [Google Scholar] [PubMed] [CrossRef]
18. Ghafarian AH, Zarghami R, Zand B, Bayat V. Wheat performance as affected by foliar application of molybdenum (Mo) under drought stress condition. Intl J Agron Plant Prod. 2013;4(11):3050–6. [Google Scholar]
19. Zoz T, Steiner F, Testa JV, Seidel EP, Fey R, Castagnara DD, et al. Foliar fertilization with molybdenum in wheat. Semin-Cienc Agrar. 2012;33(2):633–8. doi:10.5433/1679-0359. [Google Scholar] [CrossRef]
20. Hadi F, Ali N, Fuller MP. Molybdenum (Mo) increases endogenous phenolics, proline and photosynthetic pigments and the phytoremediation potential of the industrially important plant Ricinuscommunis L. for removal of cadmium from contaminated soil. Environ Sci Pollut Res Int. 2016;23:20408–30. doi:10.1007/s11356-016-7230-z. [Google Scholar] [PubMed] [CrossRef]
21. Liu J, Wang Y, Li Y, Peñuelas J, Zhao Y, Sardans J, et al. Soil ecological stoichiometry synchronously regulates stream nitrogen and phosphorus concentrations and ratios. CATENA. 2023;231:107357. doi:10.1016/j.catena.2023.107357. [Google Scholar] [CrossRef]
22. Hayyawi NJ, Al-Issawi MH, Alrajhi AA, Al-Shmgani H, Rihan H. Molybdenum induces growth, yield, and defence system Mechanisms of the Mung Bean (Vigna radiata L.) under water stress conditions. Intl J Agron. 2020;2020(1):8887329. [Google Scholar]
23. Lutts S, Almansouri M, Kinet JM. Salinity and water stress have contrasting effects on the relationship between growth and cell viability during and after stress exposure in durum wheat callus. Plant Sci. 2004;167(1):9–18. doi:10.1016/j.plantsci.2004.02.014. [Google Scholar] [CrossRef]
24. Mata CG, Lamattina L. Nitric oxide induces stomatal closure and enhances the adaptive plant responses against drought stress. Plant Physiol. 2001;126(3):1196–204. doi:10.1104/pp.126.3.1196. [Google Scholar] [PubMed] [CrossRef]
25. Clarke JM, McCaig TN. Excised-leaf water retention capability as an indicator of drought resistance of Triticum genotypes. Can J Plant Sci. 1982;62(3):571–8. doi:10.4141/cjps82-086. [Google Scholar] [CrossRef]
26. Naz F, Hussain A, Iqbal A, Kiligu HY, Afzal KS, Khan SA, et al. Molecular mechanism of Cu metal and drought stress resistance triggered by Porostereum spadiceum AGH786 in Solanum lycopersicum L. Front Plant Sci. 2022;13:1029836. doi:10.3389/fpls.2022.1029836. [Google Scholar] [PubMed] [CrossRef]
27. Mohammadkhani N, Heidari R. Drought-induced accumulation of soluble sugars and proline in two maize varieties. World Appl Sci J. 2008;3(3):448–53. [Google Scholar]
28. Ali R, Gul H, Hamayun M, Rauf M, Iqbal A, Hussain A, et al. Endophytic fungi controls the physicochemical status of maize crop under salt stress. Pol J Environ Stud. 2021;31:561–73. [Google Scholar]
29. Javed J, Rauf M, Arif M, Hamayun M, Gul H, Ud-Din A, et al. Endophytic fungal consortia enhance basal drought-tolerance in Moringaoleifera by upregulating the antioxidant enzyme (APX) through Heat shock factors. Antioxidants. 2022;11(9):1669. doi:10.3390/antiox11091669. [Google Scholar] [PubMed] [CrossRef]
30. Rehman B, Javed J, Rauf M, Khan SA, Arif M, Hamayun M, et al. ACC deaminase-producing endophytic fungal consortia promotes drought stress tolerance in M. oleifera by mitigating ethylene and H2O2. Front Plant Sci. 2022;13:967672. doi:10.3389/fpls.2022.967672. [Google Scholar] [PubMed] [CrossRef]
31. Rauf M, Ur-Rahman A, Arif M, Gul H, Ud-Din A, Hamayun M, et al. Immunomodulatory molecular mechanisms of Luffacylindrica for downy mildews resistance induced by growth-promoting endophytic fungi. J Fungi. 2022;8(7):689. doi:10.3390/jof8070689. [Google Scholar] [PubMed] [CrossRef]
32. Nagata M, Yamashita I. Simple method for simultaneous determination of chlorophyll and carotenoids in tomato fruit. Nippon Shokuhin Kogyo Gakkaishi. 1992;39(10):925–8. doi:10.3136/nskkk1962.39.925. [Google Scholar] [PubMed] [CrossRef]
33. Makkar HPS, Blummel M, Borowy NK, Becker K. Gravimetric determination of tannins and their correlations with chemical and protein precipitation methods. J Sci Food Agric. 1993;61:161–5. doi:10.1002/jsfa.v61:2. [Google Scholar] [CrossRef]
34. Rahman KU, Ali K, Rauf M, Arif M. Aspergillus nomiae and fumigatus ameliorating the hypoxic stress induced by waterlogging through ethylene metabolism in Zea mays L. Microorganisms. 2023;11(8):2025. doi:10.3390/microorganisms11082025. [Google Scholar] [PubMed] [CrossRef]
35. Benizri E, Courtade A, Picard C, Guckert A. Role of maize root exudates in the production of auxins by Pseudomonas fluorescens M. 3.1. Soil Biol Biochem. 1998;30(10):1481–4. [Google Scholar]
36. Khan Z, Gul H, Rauf M, Arif M, Hamayun M, Ud-Din A, et al. Sargassum wightii aqueous extract improved salt stress tolerance in Abelmoschus esculentus by mediating metabolic and ionic rebalance. Front Mar Sci. 2022;9:853272. doi:10.3389/fmars.2022.853272. [Google Scholar] [CrossRef]
37. Jagota SK, Dani HM. A new colorimetric technique for the estimation of vitamin C using Folin phenol reagent. Anal Biochem. 1982;127(1):178–82. doi:10.1016/0003-2697(82)90162-2. [Google Scholar] [PubMed] [CrossRef]
38. Ergun N, Topcuogu SF, Yildiz A. Auxin (Indole-3-acetic acid), gibberellic acid (GA3), abscisic acid (ABA) and cytokinin (zeatin) production by some species of mosses and lichens. Turk J Bot. 2002;26(1):13–8. [Google Scholar]
39. Prieto P, Pineda M, Aguilar M. Spectrophotometric quantitation of antioxidant capacity through the formation of a phosphomolybdenum complex: specific application to the determination of vitamin E. Anal Biochem. 1999;269(2):337–41. doi:10.1006/abio.1999.4019. [Google Scholar] [PubMed] [CrossRef]
40. Gul H, Ali R, Rauf M, Hamayun M, Arif M, Khan SA, et al. Aspergillus welwitschiae BK isolate ameliorates the physicochemical characteristics and mineral profile of maize under salt stress. Plants. 2023;12(8):1703. doi:10.3390/plants12081703. [Google Scholar] [PubMed] [CrossRef]
41. Egley GH, Paul RN, Vaughn KC, Duke SO. Role of peroxidase in the development of water-impermeable seed coats in Sidaspinosa L. Planta. 1983;157:224–32. doi:10.1007/BF00405186. [Google Scholar] [PubMed] [CrossRef]
42. Nakano Y, Asada K. Hydrogen peroxide is scavenged by ascorbate-specific peroxidase in spinach chloroplasts. Plant Cell Physiol. 1981;22(5):867–80. [Google Scholar]
43. Yokawa K, Kagenishi T, Baluska F. UV-B induced generation of reactive oxygen species promotes formation of BFA-induced compartments in cells of Arabidopsis root apices. Front Plant Sci. 2016;6:1162. [Google Scholar] [PubMed]
44. Alie K, Awais M, Ud-Din A, Aziz UD, Awais M, Rahman MM, et al. Molecular mechanisms of the 1-aminocyclopropane-1-carboxylic acid (ACC) deaminase producing Trichodermavasperellum MAP1 in enhancing wheat tolerance to waterlogging stress. Front Plant Sci. 2021;11:2213. [Google Scholar]
45. Alik R, Sajid ZH, Lee IJ, Aziz UD, Khilji SA, Husna, et al. Growth-promoting endophytic fungus (Stemphyliumlycopersici) ameliorates salt stress tolerance in maize by balancing ionic and metabolic status. Front Plant Sci. 2022;13:890565. doi:10.3389/fpls.2022.890565. [Google Scholar] [PubMed] [CrossRef]
46. Nayar PK, Misra AK, Patnaik S. Rapid microdetermination of silicon in rice plant. Plant and Soil. 1975;42:491–4. doi:10.1007/BF00010025. [Google Scholar] [CrossRef]
47. Kamburova M, Kostova D. Tetrazolium violet--a new spectrophotometric reagent for molybdenum determination. Chemija. 2008;19(2):13–8. [Google Scholar]
48. Untergasser A, Cutcutache I, Koressaar T, Ye J, Faircloth BC, Remm M, et al. Primer3—new capabilities and interfaces. Nucleic Acids Res. 2012;40(15):115. doi:10.1093/nar/gks596. [Google Scholar] [PubMed] [CrossRef]
49. Zargar SM, Mahajan R, Bhat JA, Nazir M, Deshmukh R. Role of silicon in plant stress tolerance: opportunities to achieve a sustainable cropping system. 3 Biotech. 2019;9(3):73. doi:10.1007/s13205-019-1613-z. [Google Scholar] [PubMed] [CrossRef]
50. Tejada-Jiménez M, Chamizo-Ampudia A, Galván A, Fernández E, Llamas Á. Molybdenum metabolism in plants. Metallomics. 2013;5(9):1191–203. doi:10.1039/c3mt00078h. [Google Scholar] [PubMed] [CrossRef]
51. Mendel RR. Metabolism of molybdenum. Metallomics and Cell. 2013;12:503–28. doi:10.1007/978-94-007-5561-1. [Google Scholar] [CrossRef]
52. Rihan HZ, Al-Issawi M, Al Shamari M, Woldie WA, Kiernan M, Fuller MP. The effect of molybdenum on the molecular control of cold tolerance in cauliflower (Brassica oleracea var. botrytis) artificial seeds. PCTOC. 2014;118:215–28. doi:10.1007/s11240-014-0475-7. [Google Scholar] [CrossRef]
53. Siddiqui MH, Al-Whaibi MH, Faisal M, Al Sahli AA. Nano-silicon dioxide mitigates the adverse effects of salt stress on Cucurbita pepo L. Environ Toxicol Chem. 2014;33(11):2429–37. doi:10.1002/etc.v33.11. [Google Scholar] [CrossRef]
54. Manuchehri R, Salehi H. Physiological and biochemical changes of common bermudagrass (Cynodon dactylon [L.] Pers.) under combined salinity and deficit irrigation stresses. S Afr J Bot. 2014;1(92):83–8. [Google Scholar]
55. Zhi QQ, Chen Y, Hu H. Physiological and transcriptome analyses reveal tissue-specific responses of Leucaena plants to drought stress. Plant Physio Bioch. 2024;9:108926. [Google Scholar]
56. Chen D, Wang S, Yin L, Deng X. How does silicon mediate plant water uptake and loss under water deficiency? Front Plant Sci. 2018;9:340168. [Google Scholar]
57. Rizwan M, Emam MA, Ibrahim M, Farid M, Adrees M, Bharwana SA, et al. Mechanisms of silicon-mediated alleviation of drought and salt stress in plants: a review. Environ Sci Pollut Res. 2015;22:15416–31. doi:10.1007/s11356-015-5305-x. [Google Scholar] [PubMed] [CrossRef]
58. Shi Y, Zhang Y, Han W, Feng R, Hu Y, Guo J, et al. Silicon enhances water stress tolerance by improving root hydraulic conductance in Solanum lycopersicum L. Front Plant Sci. 2016;7:196. [Google Scholar] [PubMed]
59. Khattab HI, Emam MA, Emam MM, Helal NM, Mohamed MR. Effect of selenium and silicon on transcription factors NAC5 and DREB2A involved in drought-responsive gene expression in rice. Biol Plant. 2014;58:265–73. doi:10.1007/s10535-014-0391-z. [Google Scholar] [CrossRef]
60. Sharma A, Pandey H, Devadas VS, Kartha BD, Vashishth A. Beneficial effect of heavy metals, antagonistic effect and gene regulations in nutrient translocation through soilless culture. J Agric Food Res. 2023;12:100600. [Google Scholar]
61. Sardans J, Penuelas J, Coll M, Vayreda J, Rivas-Ubach A. Stoichiometry of potassium is largely determined by water availability and growth in C atalonian forests. Funct Ecol. 2012;26(5):1077–89. doi:10.1111/fec.2012.26.issue-5. [Google Scholar] [CrossRef]
62. Vafaie A, Ebadi A, Rastgou B, Moghadam SH. The effects of potassium and magnesium on yield and some physiological traits of safflower (Carthamus tinctorius). Intl J Agri Crop Sci. 2013;5(17):1895–900. [Google Scholar]
63. Tadayyon A, Nikneshan P, Pessarakli M. Effects of drought stress on concentration of macro-and micro-nutrients in Castor (Ricinus communis L.) plant. J Plant Nutr. 2018;41(3):304–10. doi:10.1080/01904167.2017.1381126. [Google Scholar] [CrossRef]
64. Yi J, Li H, Zhao Y, Shao M, Zhang H, Liu M. Assessing soil water balance to optimize irrigation schedules of flood-irrigated maize fields with different cultivation histories in the arid region. Agric Water Manag. 2022;265:107543. doi:10.1016/j.agwat.2022.107543. [Google Scholar] [CrossRef]
65. Nahar K, Gretzmacher R. Effect of water stress on nutrient uptake, yield and quality of tomato (Lycopersicon esculentum Mill.) under subtropical conditions. Bodenkultur. 2002;53(1):45–51. [Google Scholar]
66. Song WY, Zhang ZB, Shao HB, Guo XL, Cao HX, Zhao HB, et al. Relationship between calcium decoding elements and plant abiotic-stress resistance. Int J Biol Sci. 2008;4(2):116. [Google Scholar] [PubMed]
67. Zhao Y, Wang H, Song B, Xue P, Zhang W, Peth S, et al. Characterizing uncertainty in process-based hydraulic modeling, exemplified in a semiarid Inner Mongolia steppe. Geoderma. 2023;440:116713. doi:10.1016/j.geoderma.2023.116713. [Google Scholar] [CrossRef]
68. Deshmukh RK, Vivancos J, Guerin V, Sonah H, Labbe C, Belzile F, et al. Identification and functional characterization of silicon transporters in soybean using comparative genomics of major intrinsic proteins in Arabidopsis and rice. Plant Mol Biol. 2013;83:303–15. doi:10.1007/s11103-013-0087-3. [Google Scholar] [PubMed] [CrossRef]
Cite This Article
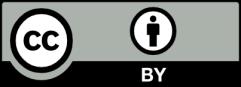
This work is licensed under a Creative Commons Attribution 4.0 International License , which permits unrestricted use, distribution, and reproduction in any medium, provided the original work is properly cited.