Open Access
REVIEW
Plant Extracts as Biostimulant Agents: A Promising Strategy for Managing Environmental Stress in Sustainable Agriculture
1 Department of Land Management, Faculty of Agriculture, Universiti Putra Malaysia, Serdang, 43400, Malaysia
2 Natural Medicines and Products Research Laboratory, Institute of Bioscience, Universiti Putra Malaysia, Serdang, 43400, Malaysia
3 Department of Cell and Molecular Biology, Faculty of Biotechnology and Biomolecular Sciences, Universiti Putra Malaysia, Serdang, 43400, Malaysia
* Corresponding Author: Susilawati Kasim. Email:
(This article belongs to the Special Issue: Abiotic Stress Impacts on Plant Physiology and Their Alleviation)
Phyton-International Journal of Experimental Botany 2024, 93(9), 2149-2166. https://doi.org/10.32604/phyton.2024.054009
Received 16 May 2024; Accepted 05 August 2024; Issue published 30 September 2024
Abstract
It is imperative to enhance crop yield to meet the demands of a burgeoning global population while simultaneously safeguarding the environment from adverse impacts, which is one of the dominant challenges confronting humanity in this phase of global climate change. To overcome this problem and reduce dependency on chemical fertilizer, scientists now view the implementation of biostimulant strategies as a cost-effective and environmentally friendly approach to achieving sustainable agriculture. Plant extracts are rich in bioactive phytocompounds, which can enhance plant resistance to disease, pest, and abiotic stresses (e.g., drought, salinity, and extreme temperature), and promote plant growth and productivity. Furthermore, the application of plant extracts through soil drenching can also significantly change the rhizosphere soil microbiome, and indirectly interact with plants, eventually stabilizing plant growth. Currently, the application of plant extracts as a whole is effective, which emphasizes the contribution of complex interactions between multiple compounds, with seaweed extracts being the most widely studied and utilized. Interestingly, plant extracts are compatible with fertilizer and can be applied in conjunction with nutrient inputs to further enhance their effectiveness. Given all this knowledge, exploring the growth and functional effects induced by plant extracts, as well as understanding their interactions and mechanisms in plants, is crucial for developing advantageous approaches with potential value in integrated crop management systems, ultimately contributing to sustainable production.Keywords
Currently, environmental stresses resulting from intensifying global warming and climate change remain the biggest challenges facing the agricultural industry, severely restricting crop yield [1]. As a result of these environmental changes, abiotic stresses including drought, extreme temperatures, salinity, and heavy metal toxicity have caused major crop reductions of up to 50% [2]. Thus, boosting global food production while enhancing crop quality and minimizing the environmental impact of agricultural practices is one of the challenges to ensuring food security [3]. With the genetic potential of major crops reaching their limits and the amount of arable land shrinking, achieving this goal becomes even more challenging. To meet this demand, it is estimated that approximately 65,000 tons of pesticides and over 2.16 million tons of mineral fertilizers are used annually to achieve expected crop yields [4]. However, the misuse of fertilizers leads to lower crop yield and causes damage to the environment and human health [5]. Thus, to ensure sustainable natural agro-ecosystems for future generations without increasing the use of fertilizers and pesticides, while improving crop safety and yield, we need to both improve the efficiency of nutrient uptake and enhance the natural defense mechanisms of plants against biotic and abiotic stresses [6,7]. With these objectives in mind, research into natural resources, such as plant extracts, is being explored as alternatives to traditional chemicals. These natural agents are able to stimulate plant growth, reduce the effects of abiotic and biotic stresses, and decrease the dependence of the growing system on fertilizers and pesticides [8,9].
Bio-stimulants (BIOs) are defined as “materials which contain substance(s) and/or microorganisms, whose function when applied to plants or the rhizosphere is to stimulate natural processes to enhance/benefit nutrient uptake, nutrient efficiency, tolerance to abiotic stress, and/or crop quality, independent of its nutrient content” (EBIC, http://www.biostimulants.eu/ (accessed on 15 May 2024) [10]). As defined, BIOs can be a variety of inorganic, and organic substances. Among them, organic non-microbial BIOs include amino acids, plant extracts, protein hydrolysates, humic acid-derived substances, and other organic compounds isolated from microorganisms, such as siderophores and butanolide-derived compounds. BIOs interact with plants to protect and stimulate plant growth and development, both directly and indirectly [11]. New compounds with bio-stimulatory activity are frequently reported in the literature. Therefore, Carletti et al. [12] proposed the need to better understand BIOs’ molecular effects to improve their classification.
Research efforts over the decades have focused on finding various effective synthetic plant growth regulators that not only benefit crop yields but also protect them from biotic stresses. For example, the exogenous application of synthetic strigolactone GR24 can produce high germination rates and increase salt tolerance in sunflowers and wheat [13–15]. However, the use of synthetic chemicals can pose risks to human health and environmental safety. Thus, the application of BIOs in agricultural practice is considered an environmentally friendly and low-cost strategy to alleviate abiotic and biotic stresses, while effectively reducing the reliance on pesticides and chemical fertilizers.
The beneficial functional effects of various BIOs, including humic substances (HS), plant growth-promoting bacteria (PGPB), and protein hydrolysates (PHs), on plants have been extensively documented [16]. However, in comparison to these BIOs, the extraction process of plant extracts is relatively simple, cost-effective, and environmentally friendly. Currently, seaweed extract is the most widely studied and utilized plant extract BIO [17]. Additionally, plant extracts can be derived from abundant raw materials, such as weeds and other non-economic plants. It is noteworthy that weeds, which are often overlooked by farmers and administrators, not only compete with crops for nutrients and space but also provide habitats for numerous pests, thereby significantly impeding crop production [18]. Conversely, studies have shown that extracts from certain weeds, such as Euphorbia hirta and Elusine indica, are rich in antioxidants, including phenolics and flavonoids, and exhibit positive effects on antibacterial activity and free radical scavenging activity [19,20]. It is necessary to emphasize further research and development of plant extracts, particularly from weed resources, to facilitate their transformation from agricultural nuisances to valuable biofertilizers. Thus, in this review, we will focus on BIOs, particularly plant extracts, as abiotic and biotic mitigators. Bioactive substances in plant extracts, such as hormones initiate symbiosis with beneficial microorganisms, protect plants from environmental stresses, promote plant growth, and enhance plants’ endogenous defense systems against diseases [21,22]. It is critical to improve nutrient uptake efficiency and availability by analyzing the abiotic and biotic stresses that plants may encounter, along with their requirements for macro-and micro-nutrients, while simultaneously minimizing costs and reducing environmental pollution. Hence, various bio-stimulant strategies have been proposed to promote sustainable agriculture [8]. Soil-drenching BIOs can modulate the soil microbial community, enhance soil cation exchange capacity, improve mineral nutrient content, and regulate soil pH values [23].
This review has compiled and summarized numerous studies on the potential physiological and molecular mechanisms of plant extracts in regulating plant growth and development under environmental stresses. These findings may help the scientific community and the agricultural industry in understanding how plant extracts influence plant regulation and provide fundamental insights for future research and development directions.
2 Plant Extracts, Methods of Extraction and Application into Plants
Plant extraction typically involves both physical methods (pressure, microwaves and heat) and chemical methods (organic solvents, alkali and acids). Varied extraction techniques are selected to address the complexities of plant structures and to separate bioactive substances from insoluble residues in various solvents to obtain soluble plant metabolites. Among them, the most common method is to use water or organic solvents (methanol and ethanol) for extraction, as it is straightforward and cost-effective. Additionally, the use of alkali extraction under high-pressure conditions is also common. While this method has been found to be highly effective, it may lead to the degradation of some hormone molecules. However, alkali extraction achieves high extraction rates and has moderate degradation of polysaccharides into biologically active oligomers, which are important components of seaweed extract [24]. The initial crude extracts typically contain a complex mixture of various plant metabolites, necessitating further processing before they can be used as medicinal products. Furthermore, these initial extracts tend to be less refined when employed in agricultural applications.
The application method used to apply plant extracts plays a crucial role in their utilization and the responses elicited by the plant (Fig. 1). The most common application types are foliar spraying, soil drenching, or a combination of both. Plant extracts can be applied to soil or growing media through fertilization, drizzling, or dripping [17]. However, the concentration of plant extracts also significantly impacts their effectiveness, as excessively high concentrations may negatively affect plant growth and development. A study has reported that foliar spraying with concentrations less than or equal to 0.05% v/v of the extract is optimal for crops by enhancing disease control and improving yields [25]. The superior performance of foliar application is attributed to direct interaction with plant tissues, as foliar absorption occurs most immediately. Additionally, soil particles can adsorb plant extracts, which may reduce their immediate mobility [26]. Conversely, Elansary et al. [27] showed that soil drenching seaweed extract had better effects on plant (Spiraea and Pittosporum) growth regulation and drought resistance compared to foliar spraying under drought stress. Moreover, the optimal application time of plant extracts was found to be approximately 10–14 days to stimulate the best plant responses [28]. Overall, the quality of plant extracts is primarily influenced by three parameters; the plant part used for the initial crude, the solvent employed for extraction, and the extraction method applied.
Figure 1: The effect of plant extracts in mitigating various abiotic and biotic stresses faced by plants
3 Effect of Plant Extracts on Plant Growth and Development
As BIOs have become a focal point in sustainable agriculture in recent years, plant extracts as a type of bio-stimulant have received increasing attention, and researchers have highly explored their potential mechanisms for promoting plant growth (Table 1). Various plant extracts have been revealed to exert beneficial effects on seed germination and promote plant growth at different developmental stages [26]. Seaweeds are large macroscopic multicellular marine algae that can be green, red, and brown in colour, and are abundant and cheap sources of fertilizer nutrients and organic matter. As a result, seaweed extract (SWE), considered to be one of the most extensively studied types of plant extract, is available through various processes including alkali and acid extraction methods, and has been utilized as a bio-stimulant to improve plant yield and abiotic tolerance by regulating physiological and biochemical characteristics [29]. In addition to SWE, researchers have also explored a variety of other plant extracts. For instance, cypress leaf extract has been shown to play a crucial role in promoting the growth of zucchini plants by enhancing photosynthesis and antioxidative defense mechanisms [30]. Application of moringa (Moringa oleifera Lam.) extracts has been demonstrated to increase the yield, quality, and storage of lettuce in glasshouses [31]. Additionally, pollen grain extract has been found to enhance the performance and oil content of Ocimum basilicum by modulating the antioxidant defense system [32]. The improvement in plant growth characteristics may be attributed to the presence of small amounts of plant hormones, such as auxin, in the extracts, as well as the involvement of various stimulating processes within the plant system post-application [33]. A recent study found that foliar application of Polygonum minus extract enhanced drought tolerance in maize by regulating the osmotic and antioxidant defense system [34]. These enhancement effects have been shown to accumulate in plants, whether through foliar spraying or soil drenching. Although the mineral nutrient content in plant extracts is not high, their abundance of secondary metabolites participating in a variety of crucial metabolic pathways in plants stimulates internal regulatory mechanisms, and ultimately promotes plant development and improved productivity [30,32,34,35].
4 Effect of Plant Extracts on Plant Tolerance to Abiotic Stress
4.1 Effect on Drought and Salinity Stress
Drought and salinity are two of the most critical and harmful abiotic stresses, severely limiting crop quality and productivity worldwide [57,58]. In recent years, soil water deficits and salinization have worsened, and over time the impacts will continue to intensify [59,60]. Long-term aquifer-based irrigation poses a potential threat to sustainable agriculture and exacerbates soil salinization. These two abiotic stresses can reduce soil nutrients, lead to water deficiency and ion toxicity, negatively affect soil microorganisms and soil enzyme activity, and hinder electron transport in chloroplasts and mitochondria, which is related to the formation of reactive oxygen species (ROS) such as hydrogen peroxide (H2O2) [61,62]. Excessive ROS accumulation in plant cells can disrupt redox functions, which cause damage to cell membranes due to lipid peroxidation, and even lead to plant death [63]. To resist oxidative damage and maintain ROS homeostasis caused by drought and salinity, plants have developed two key regulatory mechanisms. One is to regulate stomatal closure by osmoprotectant accumulation such as soluble sugars. Another method is to regulate antioxidant enzyme defence systems, including catalase (CAT), superoxide dismutase (SOD), glutathione reductase (GR) and the ascorbate-glutathione (AsA-GSH) cycle [64,65].
Exogenous application of licorice (Glycyrrhiza glabra L.) root extract (LRE) has been reported to protect common bean plants against osmotic stress and salinity by enhancing cell membrane stability, water retention, and activity of the antioxidant system [45]. The application of propolis and maize grain extract alleviated salt stress in bean seedlings [66]. It was also reported to increase shoot lengths, biomass weight, and GSH and decrease malondialdehyde (MDA), H2O2 and O2− contents [46,47]. Similarly, moringa leaf extract was studied for its effects against drought stress, and it improved water utilization and physiological and biochemical properties in Cucurbita pepo L. [48]. In addition, foliar spraying of licorice extracts enhanced the drought tolerance of sesame and mitigated negative impacts [49].
4.2 Effect on Temperature Stress
Under the influence of global climate change, extreme temperatures (both high and low) are also considered to be an abiotic stress, hindering sustainable agricultural development. Plants subjected to extreme temperatures can experience disruptions in normal physiology and metabolism, ultimately limiting productivity [67]. The impacts caused by temperature stress vary depending on the degree of temperature change, exposure time, and type of plant. Additionally, plant physiology and antioxidant defense systems respond differently to short-term and rapid changes in temperature than to long-term changes [68]. Especially when the temperatures are increased, it will cause inhibitory effects on various stages of plant growth. As a result, temperature stress has attracted more and more researchers’ attention [11]. In addition to heat stress, plant also face cold stress, known as chilling stress or freezing stress, which can cause damage to plant tissues. Both heat and cold stresses will negatively affect the cell membrane, cell division, water, and nutrient uptake of plants, and eventually limit plant growth and productivity. Reactive oxygen species (ROS) are frequently produced as a reult of temperature stress. Consequently, stimulating the plant antioxidant defence system is a primary mechanism for improving tolerance to extreme temperatures. The exogenous application of BIOs containing secondary metabolites is considered an useful strategy to alleviate the adverse effects associated with temperature stress [69]. For instance, the application of moringa leaf extract improved the growth of moringa seedlings under low temperature stress [70]. Similarly, Bradáčová et al. [42] reported that application of seaweed extract mitigated cold stress by improving the uptake of nutrient elements and increasing SOD activity in roots and leaves. Generally, heat stress often coexists with other stresses such as water deficit or salinity, causing more severe damage to plants. However, applying plant extracts played a key role in improving plant tolerance to multiple abiotic stresses. This is consistent with findings by Farooq et al. [52], who reported that foliar application of sorghum, brassica, sunflower, and moringa extracts improved wheat performance by increasing water use efficiency and accumulating phenolics under terminal heat and drought stress. In addition, researchers have also applied salicylic acid (SA) to protect plants from cold stress, as the accumulation of free SA and glycosyl SA in plants is an endogenous regulatory systems to withstand cold stress [71].
4.3 Effect on Heavy Metals Phytotoxicity
Heavy metal contamination of the environment has become a major global issue, especially in developing countries. The phytotoxicity caused by heavy metals in soil seriously hinders the sustainable development of agriculture [72]. Common heavy metal (HV) contaminants include Cd, As, Co, Pb, and Hg, which induce detrimental physiological and biochemical functions in plants [73]. Consequently, lower germination, growth, and productivity were found in plants undergoing HM stress [74]. To withstand the negative effects caused by HM stress, plants have also developed endogenous regulatary mechanisms, such as limiting HM uptake and stimulating antioxidant defense systems [65]. However, these intrinsic regulations are often insufficient, prompting researchers to propose the exogenous application of BIOs to help plants alleviate metal-induced stresses [75]. Plant extracts are considered to contain high levels of polyphenols and flavonoids, which help shield membrane lipids and macromolecules from ROS-mediated damage by scavenging free radicals [17]. For instance, the exogenous application of moringa leaf extract can decrease Pb, Ni, and Cd toxicity in Saccharomyces cerevisiae by inhibiting HM accumulation and metal-induced endogenous oxidative stress [76,77]. Another study demonstrated that moringa leaf extract could mitigate Cd and salinity stresses in beans [47]. In addition to plant extracts, other secondary metabolites have also been extensively studied. Zhang et al. [78] found that applying salicylic acid (SA) alleviated the harmful effects associated with Cd or Pb in Vicia sativa L. by enhancing photosynthesis. Similarly, Kotapati et al. [79] demonstrated that SA application significantly promotes plant growth by alleviating Ni toxicity. Overall, direct applying plant extracts (secondary metabolites) shows great potential as an effective strategy for mitigating HM phytotoxicity. As a result, exploring plant extracts from various plant raw materials as an environmentally-friendly biotechnological method offers promising prospects for sustainable agriculture.
5 Effect of Plant Extracts on Plant Tolerance to Biotic Stress
5.1 Effect on Bacterial Infection
Plant roots and exudates constitute the rhizosphere, where interactions between plants and microorganisms are most intense. In addition, microorganisms also colonize the interior of plants, such as tissue and cells. These two plant-microbial interaction pathways significantly affect plant development and productivity. In turn, secondary metabolites released by plants, such as amino acids and soluble sugars, can also alter composition and function of root microbiome [80]. Plant extracts, known for their abundant and biologically active secondary metabolites, can be directly applied (soil drenching) to change soil microorganisms, promote soil-plant interactions, and indirectly influence plant health. A study was conducted on tomatoes in greenhouses by applying Ascophyllum nodosum extract (ANE) to elucidate its effect on microorganisms in rhizosphere soil, and the results showed that the species composition of microorganisms was changed, and plant morphological traits were improved [81]. Interestingly, many researchers help plants to enhance environmental stress tolerance by inoculating plant growth-promoting bacteria (PGPB) [82]. Although some scholars classify PGPB as a biofertilizer, it can also be considered BIOs based on its biochemical properties. Common PGPB species such as Bacillus spp., Pseudomonas spp., and Rhodococcus spp., have been extensively studied as biostimulants [58,83,84].
5.2 Effect on Pests and Crop Diseases
Pests and crop diseases severely threaten field crop productivity, contributing to worldwide crop yield losses ranging from 20% to 40% [85]. While pesticides and insecticides have been extensively employed to prevent pest and disease infestations, their prolonged use can be harmful to both human well-being and ecosystems due to their inherent toxicity and persistence [86]. Plants have evolved mechanisms to resist pests and diseases, notably through the increased secretion of secondary metabolites, with alkaloids being the most crucial [87]. In order to enhance plant resistance to diseases, the application of plant extracts has also been employed. For instance, the exogenous application of Reynoutria sachalinensis (F. Schmidt) Nakai extract has been demonstrated to provide protection to a variety of plants, including tomatoes, cucurbit, and strawberries, against bacterial and fungal diseases [88]. Furthermore, three extracts from polyalthia, ginger, and clerodendrum have been determined to significantly inhibit the activity of Rhizoctonia solani [89]. Therefore, these findings strongly support the application of plant extracts as bio-protectants to help plants resist pathogens, offering a viable substitute for synthetic pesticides and contributing to more sustainable agricultural practices.
Weeds, as common field plants, compete with crops for water, nutrients, and other essential resources while also serving as hosts for pathogenic bacteria and pests, severely limiting agricultural production [90]. The extent of crop losses caused by weeds varies significantly based on crop species, weed control approaches, weed types, duration of infestation, and environmental stresses [91]. Currently, weed control primarily relies on resistant cultivars and herbicides. Due to the shortage of agricultural workers, there is a global trend toward increasing the reliance on herbicides to control weed densities [92]. However, the utilization of resistant plants is not universally applicable to all crop species, and relying solely on chemical herbicides poses the risk of diminishing the health benefits of cultivars or fostering herbicide resistance in weeds. As a result, these two approaches may not fully eliminate the weed problem [93]. Thus, developing innovative solutions derived from natural sources is crucial to reducing the reliance on chemical herbicides, consequently mitigating environmental-related impacts.
Biological weed control involves the use of natural predators, substances, or organisms to inhibit the germination and growth of weed populations to an economically acceptable threshold level. Allelopathy, originally defined as the effects caused by chemical compounds released by one plant on other plants, has been redefined by various ecologists, who now prefer describing it as the negative impacts exerted by one plant on others through the release of toxic compounds [94]. Drawing on the allelopathic effect, researchers have explored new phytocompounds or allelochemicals extracted from plants as bioherbicides for controlling weeds and protecting crop [91]. A study conducted by Travaini et al. [95] reported applying Ammi visnaga extract inhibits the germination, growth, and photosynthesis of Lolium multiflorum, Echinochloa crus-galli, Digitaria sanguinalis, and Setaria italica. Puig et al. [96] reported the existence of phenolic compounds (e.g., quercetin, rutin, and ellagic acid) in an aqueous extract from the leaves of Eucalyptus globulus, and the extract inhibited the germination and early growth of Agrostis stolonifera. Similarly, Chon et al. [97] revealed that the aqueous extract of Xanthium occidentale contains allelochemicals such as chlorogenic acids, and inhibits lucerne growth. In another study, application of Aglaia odorata leaf extract exhibited a significant inhibition on the growth and development of E. crus-galli and L. perenne [98]. Based on the above findings, we found that some specific plant extracts contain allelopathic chemicals, showing strong phytotoxic effects against weeds, and therefore the utilization of plant extracts offers a promising, environmentally sustainable method for weed control.
6 Mechanisms and Pathways of Biostimulatory Activity
Plant extracts contain abundant active secondary metabolites. Therefore, incorporating plant extracts will stimulates plants, triggering various internal reactions, helping plants better resist environmental stress, and ultimately promoting plant growth and development (Fig. 2). However, it should be clarified that the benefits of plant extracts on plant growth regulation are not the result of a single active substance, but the coordinated action of multiple substances, such as plant growth regulatory hormones, antioxidants, and osmoprotectants. A study has reported that Ascophyllum nodosum extract-treated tomato plants exhibited changes in the concentration of osmolytes and expression of the tas14 dehydrin gene [99]. Another study showed that overexpression of ATHB-6 in maize activated the expression of related genes in the abscisic acid (ABA)-dependent pathway and the ROS signaling pathway under drought tolerance [100]. In addition, a study conducted with brown macroalga (A. nodosum) extract on spinach revealed improved biomass weight, chlorophyll content and increased phenolics and flavonoids and was associated with increased expression of the GS1 gene, betaine aldehyde dehydrogenase, choline monooxygenase, glutathione reductase and monodehydroascorbate reductase. All these genes attributed to the flavonoid and phenylpropanoid pathway are considered to promote growth and improve N integration [101]. Similarly, cypress leaf extract-treated zucchini seedlings showed a promoting effect on photosynthesis and salt tolerance by overexpressing key genes involved in carbohydrate metabolism (RbcL and RbcS) and salt-responsive antioxidant-related genes (CAT1, APX, CuZnSOD2, DHAR, GR, and PrxQ) [30]. Together, these results demonstrate that plant extracts can stimulate a series of metabolic signaling pathways in plants, thereby providing effective protection against abiotic stress. In addition, it is noteworthy that these extracts exhibit enhanced efficacy as an integrated whole rather than as a single bioactive compound, due to the synergistic interactions among their components that create a cumulative effect that benefits the entire plant system. Consequently, the observed effect does not stem from a single defense mechanism or a specific gene, but rather emerges from the coordinated and harmonious interaction of multiple genes.
Figure 2: Functional mechanism of plant extracts of enhanced osmotic adjustment, antioxidant enzyme activities of plants under abiotic stresses. The upward and downward arrows indicate the positive and negative effect, respectively. MDA: Malondialdehyde content; H2O2: hydrogen peroxide; O2−: superoxide content; GSH: glutathione; AsA: ascorbate content; CAT: catalase activity; POD: peroxidase activity; APX: ascorbate peroxidase
7 Challenges and Perspectives for Plant Extracts
The utilization of plant extracts as natural BIOs has gained widespread attention in sustainable agricultural management and is considered by scientists and agri-technology companies as a promising technology application to mitigate the damage caused by biotic and abiotic stress while promoting crop growth and productivity. According to market investigations and surveys, the demand for such natural plant extracts is also gradually increasing, with seaweed extract being the most widely used [102]. Although some international organizations (such as the European Union) have recommended the use of BIOs in agriculture as an independent agricultural product, there are still multiple practical challenges that hinder the actual production and application of BIOs. The elucidation of molecular mechanisms governing the impacts of plant extracts represents a noteworthy challenge in their developmental trajectory. Detailed understanding of how these bioactive compounds function is imperative for optimizing their application. For instance, certain bioactive compounds exert specific effects on the metabolic pathway in plants. Consequently, the development of more precisely targeted products can be researched according to diverse plant species and various growth stages. Due to the intricate nature of multicomponent plant extracts, the challenge may seem formidable. A viable solution to this predicament involves adopting integrative and multidisciplinary approaches, such as modern molecular technology and biochemical analyses. This integrated approach aims to identify the active molecules in the extracts and elucidate their mode of action. On one hand, the application of BIOs, particularly through soil drenching, have crucial role in improving soil aggregate structure, nutrient content, and enzyme activities [56,58]. On the other hand, the soil rhizosphere microbiome has a vital role in plant growth, and its diversification can enhance the plant resistance to stresses, such as nutrient deficiencies, drought, or pathogens [103]. When plants suffer from biotic or abiotic stresses, they can receive help from the root microbiota, leading to increased selectivity of beneficial microorganisms and improved microbial activity in the soil.
This phenomenon, known as the “cry for help” theory, has gained widespread attention in recent years [104]. Accordingly, the agronomic practice of exogenous bio-stimulant application in the plant rhizosphere can precisely manage the assembly of rhizosphere microorganisms and the recruitment of beneficial microorganisms. Unveiling the intrinsic interaction and functional mechanisms between BIOs, the rhizosphere microbiome and plant growth regulation is essential for advancing sustainable agricultural development. Currently, limited success has been reported in the development of innovative commercial products suitable for application in both conventional and protected crop production systems. Ensuring sustainable harvesting and conserving biodiversity poses a challenge, particularly when the natural production of raw plant materials is limited due to unique environmental conditions. Further to this, advancements will be made in optimizing both wet and dry processing as well as preservation methods to enhance the efficiency of processing, storage, extraction, and bio-transformation [17]. Ultimately, building trust with farmers should not be overlooked, as advocated by El Boukhari et al. [105], who recommend embracing farmer-centric approaches, emphasizing education and on-field demonstrations to address the specific needs of farmers.
In this review, we outlined published reports and verified the beneficial impacts of plant extracts on plant growth, yield, and resilience to pests, pathogens, and abiotic stresses. The effectiveness of plant extracts is contingent upon the type of plant raw materials and the quality and composition of the extract, as well as the extraction method, application concentration, and frequency. The observed enhanced growth effects have exclusively manifested with the use of the whole extract, underscoring the highly interactive nature and synergistic activity among the components of plant extract on plant growth and functions. Nevertheless, the complexities inherent in the synergistic activities and interactions of bio-molecules, along with their molecular functions in plants, remain largely unresolved. Additionally, applying plant extracts through soil drenching has effects on the soil structure, heavy metals, nutrients, enzyme activity, and rhizosphere microbiome assembly which should also be emphasized. The secondary metabolism of soil microorganisms and their symbiosis with plants are also important factors affecting plant growth. Notably, a recent study reported certain microbiomes that are thought to be beneficial to plants may have negative effects on plants under environmental stresses [106]. Therefore, fully integrating the rhizosphere metabolites with plant physiology and molecular regulation will provide clearer and deeper insights into the internal regulatory mechanisms of plants to improve plant growth and stress resistance. Since agriculture and ecology are inextricably linked, assessing the long-term effects of plant extracts on non-target crops and ecosystems, cannot be ignored. Finally, additional studies should be conducted under field conditions to demonstrate the efficacy of these botanical formulations in natural settings. It is essential to propose a safe and effective plant extract application strategy for mitigating both biotic and abiotic plant stresses.
Acknowledgement: We thank Faculty of Agriculture, Universiti Putra Malaysia, for providing the quality scientific writing course that made this work possible.
Funding Statement: This paper was supported by Universiti Putra Malaysia, Innohub Grant Scheme (Vote No. 9005004), and D’Khairan Farm Sdn Bhd (Vote No. 6300349).
Author Contributions: The authors confirm contribution to the paper as follows: conceptualisation: Mingzhao Han, Susilawati Kasim; writing and editing: Mingzhao Han, Zhongming Yang, Xi Deng; supervision: Md Kamal Uddin, Noor Baity Saidi, Effyanti Mohd Shuib. All authors reviewed the results and approved the final version of the manuscript.
Availiability of Data and Materials: Not applicable.
Ethics Approval: Not applicable.
Conflicts of Interest: The authors declared that they have no conflicts of interest to report regarding the present study.
References
1. Chaudhry S, Sidhu GPS. Climate change regulated abiotic stress mechanisms in plants: a comprehensive review. Plant Cell Rep. 2021;41(1):1–31. [Google Scholar] [PubMed]
2. Francini A, Sebastiani L. Abiotic stress effects on performance of horticultural crops. Horticulturae. 2019;5(4):67. doi:10.3390/horticulturae5040067. [Google Scholar] [CrossRef]
3. Del Buono D. Can biostimulants be used to mitigate the effect of anthropogenic climate change on agriculture? It is time to respond. Sci Total Environ. 2020;751:141763. [Google Scholar] [PubMed]
4. Davydov R, Sokolov M, Hogland W, Glinushkin A, Markaryan A. The application of pesticides and mineral fertilizers in agriculture. MATEC Web Conf. 2018;245:11003. doi:10.1051/matecconf/201824511003. [Google Scholar] [CrossRef]
5. Zhang L, Zhang W, Cui Z, Hu Y, Schmidhalter U, Chen X. Environmental, human health, and ecosystem economic performance of long-term optimizing nitrogen management for wheat production. J Clean Prod. 2021;311:127620. doi:10.1016/j.jclepro.2021.127620. [Google Scholar] [CrossRef]
6. Singh N, Joshi E, Sasode DS, Dangi RS, Chouhan N. Soil fertility, macro and micronutrient uptake and their use efficiencies under integrated nutrient management in groundnut (Arachis hypogaea L.). Inter J Chem Stud. 2020;8(1):1983–7. doi:10.22271/chemi. [Google Scholar] [CrossRef]
7. Costa JAV, Freitas BCB, Cruz CG, Silveira J, Morais MG. Potential of microalgae as biopesticides to contribute to sustainable agriculture and environmental development. J Envir Sci Health Part B. 2019;54(5):366–75. doi:10.1080/03601234.2019.1571366. [Google Scholar] [PubMed] [CrossRef]
8. Bulgari R, Franzoni G, Ferrante A. Biostimulants application in horticultural crops under abiotic stress conditions. Agronomy. 2019;9(6):306. doi:10.3390/agronomy9060306. [Google Scholar] [CrossRef]
9. Cuadrado CJL, Pinillos EO, Tito R, Mirones CS, Gamarra Mendoza NN. Insecticidal properties of capsaicinoids and glucosinolates extracted from Capsicum chinense and Tropaeolum tuberosum. Insects. 2019;10(5):132. doi:10.3390/insects10050132. [Google Scholar] [PubMed] [CrossRef]
10. Ricci M, Tilbury L, Daridon B, Sukalac K. General principles to justify biostimulant claims. Front Plant Sci. 2019;10:444124. [Google Scholar]
11. Mrid RB, Benmrid B, Hafsa J, Boukcim H, Sobeh M, Yasri A. Secondary metabolites as biostimulant and bioprotectant agents: a review. Sci Total Environ. 2021;777:146204. doi:10.1016/j.scitotenv.2021.146204. [Google Scholar] [CrossRef]
12. Carletti P, García AC, Silva CA, Merchant A. Editorial: towards a functional characterization of plant biostimulants. Front Plant Sci. 2021;12:677772. doi:10.3389/fpls.2021.677772. [Google Scholar] [PubMed] [CrossRef]
13. Boyer FD, de Saint Germain A, Pouvreau JB, Clavé G, Pillot JP, Roux A, et al. New strigolactone analogs as plant hormones with low activities in the rhizosphere. Mol Plant. 2014;7(4):675–90. doi:10.1093/mp/sst163. [Google Scholar] [PubMed] [CrossRef]
14. Sarwar Y, Shahbaz M. Modulation in growth, photosynthetic pigments, gas exchange attributes and inorganic ions in sunflower (Helianthus annuus L.) by strigolactones (GR24) achene priming under saline conditions. Pak J Bot. 2020;52:23–31. [Google Scholar]
15. Kausar F, Shahbaz M. Influence of strigolactone (GR24) as a seed treatment on growth, gas exchange and chlorophyll fluorescence of wheat under saline conditions. Int J Agric Biol. 2017;19:321–7. doi:10.17957/IJAB. [Google Scholar] [CrossRef]
16. Baltazar M, Correia S, Guinan KJ, Sujeeth N, Bragança R, Gonçalves B. Recent advances in the molecular effects of biostimulants in plants: an overview. Biomolecules. 2021;11(8):1096. doi:10.3390/biom11081096. [Google Scholar] [PubMed] [CrossRef]
17. Ali O, Ramsubhag A, Jayaraman J. Biostimulant properties of seaweed extracts in plants: implications towards sustainable crop production. Plants. 2021;10(3):531. doi:10.3390/plants10030531. [Google Scholar] [PubMed] [CrossRef]
18. Haq SM, Lone FA, Kumar M, Calixto ES, Waheed M, Casini R, et al. Phenology and diversity of weeds in the agriculture and horticulture cropping systems of Indian Western Himalayas: understanding implications for agro-ecosystems. Plants. 2023;12(6):1222. doi:10.3390/plants12061222. [Google Scholar] [PubMed] [CrossRef]
19. Iqbal M, Gnanaraj C. Eleusine indica L. possesses antioxidant activity and precludes carbon tetrachloride (CCl4)-mediated oxidative hepatic damage in rats. Environ Health Prev. 2012;17(4):307–15. doi:10.1007/s12199-011-0255-5. [Google Scholar] [PubMed] [CrossRef]
20. Sharma NK, Dey S, Prasad R. In vitro antioxidant potential evaluation of Euphorbia hirta L. Pharmacologyonline. 2007;1:91–8. [Google Scholar]
21. Noman A, Aqeel M, Qasim M, Haider I, Lou Y. Plant-insect-microbe interaction: a love triangle between enemies in ecosystem. Sci Total Environ. 2020;699:134181. doi:10.1016/j.scitotenv.2019.134181. [Google Scholar] [PubMed] [CrossRef]
22. Poveda J, Eugui D, Velasco P. Natural control of plant pathogens through glucosinolates: an effective strategy against fungi and oomycetes. Phytochem Rev. 2020;19(4):1045–59. doi:10.1007/s11101-020-09699-0. [Google Scholar] [CrossRef]
23. Rouphael Y, Colla G. Synergistic biostimulatory action: designing the next generation of plant biostimulants for sustainable agriculture. Front Plant Sci. 2018;9:1655. doi:10.3389/fpls.2018.01655. [Google Scholar] [PubMed] [CrossRef]
24. Shukla PS, Mantin EG, Adil M, Bajpai S, Critchley AT, Prithiviraj B. Ascophyllum nodosum-based biostimulants: sustainable applications in agriculture for the stimulation of plant growth, stress tolerance, and disease management. Front Plant Sci. 2019;10:655. doi:10.3389/fpls.2019.00655. [Google Scholar] [PubMed] [CrossRef]
25. Ali N, Farrell A, Ramsubhag A, Jayaraman J. The effect of Ascophyllum nodosum extract on the growth, yield and fruit quality of tomato grown under tropical conditions. J Appl Phycol. 2016;28:1353–62. doi:10.1007/s10811-015-0608-3. [Google Scholar] [CrossRef]
26. Ali O, Ramsubhag A, Jayaraman J. Phytoelicitor activity of Sargassum vulgare and Acanthophora spicifera extracts and their prospects for use in vegetable crops for sustainable crop production. J Appl Phycol. 2021;33:639–51. doi:10.1007/s10811-020-02309-8. [Google Scholar] [CrossRef]
27. Elansary HO, Skalicka-Woźniak K, King IW. Enhancing stress growth traits as well as phytochemical and antioxidant contents of Spiraea and Pittosporum under seaweed extract treatments. Plant Physiol Bioch. 2016;105:310–20. doi:10.1016/j.plaphy.2016.05.024. [Google Scholar] [PubMed] [CrossRef]
28. Arioli T, Mattner SW, Winberg PC. Applications of seaweed extracts in Australian agriculture: past, present and future. J Appl Phycol. 2015;27:2007–15. doi:10.1007/s10811-015-0574-9. [Google Scholar] [PubMed] [CrossRef]
29. Sujata Goyal V, Baliyan V, Avtar R, Mehrotra S. Alleviating drought stress in Brassica juncea (L.) Czern & Coss. by foliar application of biostimulants-orthosilicic acid and seaweed extract. Appli Biochem Biotech. 2023;195(1):693–721. doi:10.1007/s12010-022-04085-2. [Google Scholar] [PubMed] [CrossRef]
30. ElSayed AI, Rafudeen MS, Ganie SA, Hossain MS, Gomaa AM. Seed priming with cypress leaf extract enhances photosynthesis and antioxidative defense in zucchini seedlings under salt stress. Scientia Horti. 2022;293:110707. doi:10.1016/j.scienta.2021.110707. [Google Scholar] [CrossRef]
31. Admane N, Cavallo G, Hadjila C, Cavalluzzi MM, Rotondo NP, Salerno A, et al. Biostimulant formulations and Moringa oleifera extracts to improve yield, quality, and storability of hydroponic lettuce. Molecules. 2023;28(1):373. doi:10.3390/molecules28010373. [Google Scholar] [PubMed] [CrossRef]
32. Taha RS, Alharby HF, Bamagoos AA, Medani RA, Rady MM. Elevating tolerance of drought. stress in Ocimum basilicum using pollen grains extract; a natural biostimulant by regulation of plant performance and antioxidant defense system. S Afr J Bot. 2020;128:42–53. doi:10.1016/j.sajb.2019.09.014. [Google Scholar] [CrossRef]
33. Lucini L, Rouphael Y, Cardarelli M, Bonini P, Baffi C, Colla G. A vegetal biopolymer-based biostimulant promoted root growth in melon while triggering brassinosteroids and stress-related compounds. Front Plant Sci. 2018;9:472. doi:10.3389/fpls.2018.00472. [Google Scholar] [PubMed] [CrossRef]
34. Han M, Kasim S, Yang Z, Deng X, Uddin MK, Saidi NB, et al. Application of polygonum minus extract in enhancing drought tolerance in maize by regulating osmotic and antioxidant system. Phyton-Int J Exp Bot. 2024;93(2):213–26. doi:10.32604/phyton.2024.047150. [Google Scholar] [CrossRef]
35. Ali O, Ramsubhag A, Jayaraman J. Biostimulatory activities of Ascophyllum nodosum extract in tomato and sweet pepper crops in a tropical environment. PLoS One. 2019;14:e0216710. doi:10.1371/journal.pone.0216710. [Google Scholar] [PubMed] [CrossRef]
36. Ali O, Ramsubhag A, Jayaraman J. Ascophyllum nodosum (Linnaeus) Le Jolis seaweed extract improves seed germination in tomato and sweet pepper under NaCl-induced salt stress. Trop Agr. 2018;95:141–8. [Google Scholar]
37. Al-Ghamdi AA, Elansary HO. Synergetic effects of 5-aminolevulinic acid and Ascophyllum nodosum seaweed extracts on asparagus phenolics and stress related genes under saline irrigation. Plant Physiol Bioch. 2018;129:273–84. doi:10.1016/j.plaphy.2018.06.008. [Google Scholar] [PubMed] [CrossRef]
38. Borella M, Baghdadi A, Bertoldo G, Della Lucia MC, Chiodi C, Celletti S, et al. Transcriptomic and physiological approaches to decipher cold stress mitigation exerted by brown-seaweed extract application in tomato. Front Plant Sci. 2023;14:1232421. doi:10.3389/fpls.2023.1232421. [Google Scholar] [PubMed] [CrossRef]
39. Esserti S, Smaili A, Rifai LA, Koussa T, Makroum K, Belfaiza M, et al. Protective effect of three brown seaweed extracts against fungal and bacterial diseases of tomato. J Appl Phycol. 2017;29:1081–93. doi:10.1007/s10811-016-0996-z. [Google Scholar] [CrossRef]
40. Domingo G, Marsoni M, Álvarez-Viñas M, Torres MD, Domínguez H, Vannini C. The role of protein-rich extracts from Chondrus crispus as biostimulant and in enhancing tolerance to drought stress in tomato plants. Plants. 2023;12(4):845. doi:10.3390/plants12040845. [Google Scholar] [PubMed] [CrossRef]
41. Zhang L, Freschi G, Rouphael Y, de Pascale S, Lucini L. The differential modulation of secondary metabolism induced by a protein hydrolysate and a seaweed extract in tomato plants under salinity. Front Plant Sci. 2023;13:1072782. doi:10.3389/fpls.2022.1072782. [Google Scholar] [PubMed] [CrossRef]
42. Bradáčová K, Weber NF, Morad-Talab N, Asim M, Imran M, Weinmann, et al. Micronutrients (Zn/Mn), seaweed extracts, and plant growth-promoting bacteria as cold-stress protectants in maize. Chem. Biol. Technol Ag. 2016;3(1):1–10. [Google Scholar]
43. Yusuf R, Kristianse P, Warwick N. Effect of two seaweed products and equivalent mineral treatments on lettuce (Lactuca sativa L.) growth. J Agro. 2019;18(3):100–6. doi:10.3923/ja.2019.100.106. [Google Scholar] [CrossRef]
44. Di Mola I, Cozzolino E, Ottaiano L, Giordano M, Rouphael Y, Colla G, et al. Effect of vegetal- and seaweed extract-based biostimulants on agronomical and leaf quality traits of plastic tunnel-grown baby lettuce under four regimes of nitrogen fertilization. Agronomy. 2019;9(10):571. doi:10.3390/agronomy9100571. [Google Scholar] [CrossRef]
45. Rady MM, Desoky ESM, Elrys AS, Boghdady MS. Can licorice root extract be used as an effective natural biostimulant for salt-stressed common bean plants? S Afr J Bot. 2019;121:294–305. doi:10.1016/j.sajb.2018.11.019. [Google Scholar] [CrossRef]
46. Rady MM, Varma B, Howladar SM. Common bean (Phaseolus vulgaris L.) seedlings overcome NaCl stress as a result of presoaking in Moringa oleifera leaf extract. Sci Hortic. 2013;162:63–70. doi:10.1016/j.scienta.2013.07.046. [Google Scholar] [CrossRef]
47. Howladar SM. A novel Moringa oleifera leaf extract can mitigate the stress effects of salinity and cadmium in bean (Phaseolus vulgaris L.) plants. Ecotox Environ Safe. 2014;100:69–75. doi:10.1016/j.ecoenv.2013.11.022. [Google Scholar] [PubMed] [CrossRef]
48. Abd El-Mageed TA, Semida WM, Rady MM. Moringa leaf extract as biostimulant improves water use efficiency, physio-biochemical attributes of squash plants under deficit irrigation. Agr Water Manage. 2017;193:46–54. doi:10.1016/j.agwat.2017.08.004. [Google Scholar] [CrossRef]
49. Pourghasemian N, Moradi R, Naghizadeh M, Landberg T. Mitigating drought stress in sesame by foliar application of salicylic acid, beeswax waste and licorice extract. Agr Water Manage. 2020;231:105997. doi:10.1016/j.agwat.2019.105997. [Google Scholar] [CrossRef]
50. Jayaraman J, Norrie J, Punja ZK. Commercial extract from the brown seaweed Ascophyllum nodosum reduces fungal diseases in greenhouse cucumber. J Appl Phycol. 2011;23:353–61. doi:10.1007/s10811-010-9547-1. [Google Scholar] [CrossRef]
51. Jaulneau V, Lafitte C, Corio-Costet MF, Stadnik MJ, Salamagne S, Briand X, et al. An Ulva armoricana extract protects plants against three powdery mildew pathogens. Eur J Plant Pathol. 2011;131:393–401. doi:10.1007/s10658-011-9816-0. [Google Scholar] [CrossRef]
52. Farooq M, Rizwan M, Nawaz A, Rehman A, Ahmad R. Application of natural plant extracts improves the tolerance against combined terminal heat and drought stresses in bread wheat. J Agron Crop Sci. 2017;203(6):528–38. doi:10.1111/jac.2017.203.issue-6. [Google Scholar] [CrossRef]
53. Kasim WA, Hamada EAM, Shams El-Din NG, Eskander SK. Influence of seaweed extracts on the growth, some metabolic activities and yield of wheat grown under drought stress. Int J Agron Agric Res. 2015;7(2):173–89. [Google Scholar]
54. Sharma S, Chen C, Khatri K, Rathore MS, Pandey SP. Gracilaria dura extract confers drought tolerance in wheat by modulating abscisic acid homeostasis. Plant Physiol Bioch. 2019;136:143–54. doi:10.1016/j.plaphy.2019.01.015. [Google Scholar] [PubMed] [CrossRef]
55. El-Sappah AH, Metwally MA, Rady MM, Ali HM, Wang L, Maitra P, et al. Interplay of silymarin and clove fruit extract effectively enhances cadmium stress tolerance in wheat (Triticum aestivum). Front Plant Sci. 2023;14:1144319. doi:10.3389/fpls.2023.1144319. [Google Scholar] [PubMed] [CrossRef]
56. Chen D, Li Z, Yang J, Zhou W, Wu Q, Shen H, et al. Seaweed extract enhances drought resistance in sugarcane via modulating root configuration and soil physicochemical properties. Ind Crop Prod. 2023;194:116321. doi:10.1016/j.indcrop.2023.116321. [Google Scholar] [CrossRef]
57. Xie Z, Chu Y, Zhang W, Lang D, Zhang X. Bacillus pumilus alleviates drought stress and increases metabolite accumulation in Glycyrrhiza uralensis Fisch. Environ Exp Bot. 2019;158:99–106. doi:10.1016/j.envexpbot.2018.11.021. [Google Scholar] [CrossRef]
58. Zheng L, Ma X, Lang D, Zhang X, Zhou L, Wang L, et al. Encapsulation of Bacillus pumilus G5 from polyvinyl alcohol-sodium alginate (PVA-SA) and its implications in improving plant growth and soil fertility under drought and salt soil conditions. Int J Biol Macromol. 2022;209:231–43. doi:10.1016/j.ijbiomac.2022.04.017. [Google Scholar] [PubMed] [CrossRef]
59. Naeem M, Iqbal M, Shakeel A, Ul-Allah S, Hussain M, Rehman et al. Genetic basis of ion exclusion in salinity stressed wheat: implications in improving crop yield. Plant Growth Regul. 2020;92:479–96. doi:10.1007/s10725-020-00659-4. [Google Scholar] [CrossRef]
60. Rezayian M, Niknam V, Ebrahimzadeh H. Penconazole and calcium ameliorate drought stress in canola by upregulating the antioxidative enzymes. Funct Plant Biol. 2020;47(9):825–39. doi:10.1071/FP19341. [Google Scholar] [PubMed] [CrossRef]
61. Bhardwaj R, Kaur R, Bali S, Kaur P, Sirhindi G, Thukral K, et al. Role of various hormones in photosynthetic responses of green plants under environmental stresses. Curr Protein Pept Sc. 2015;16(5):435–49. doi:10.2174/1389203716666150330125215. [Google Scholar] [PubMed] [CrossRef]
62. Chamberlain SD, Hemes KS, Eichelmann E, Szutu DJ, Verfaillie JG, Baldocchi DD. Effect of drought-induced salinization on wetland methane emissions, gross ecosystem productivity, and their interactions. Ecosystems. 2020;23:675–88. doi:10.1007/s10021-019-00430-5. [Google Scholar] [CrossRef]
63. Mrid RB, El Omari R, El Mourabit N, Bouargalne Y, Nhiri M. Changes in the antioxidant and glyoxalase enzyme activities in leaves of two Moroccan sorghum ecotypes with differential tolerance to nitrogen stress. Aust J Crop Sci. 2018;12(8):1280–7. doi:10.21475/ajcs. [Google Scholar] [CrossRef]
64. Sen A, Alikamanoglu S. Antioxidant enzyme activities, malondialdehyde, and total phenolic content of PEG-induced hyperhydric leaves in sugar beet tissue culture. In Vitro Cell Dev-Pl. 2013;49:396–404. doi:10.1007/s11627-013-9511-2. [Google Scholar] [CrossRef]
65. Bhardwaj RD, Kaur L, Srivastava P. Comparative evaluation of different phenolic acids as priming agents for mitigating drought stress in wheat seedlings. Proc Natl Acad Sci India Sect B Biol Sci. 2017;87:1133–42. doi:10.1007/s40011-015-0690-y. [Google Scholar] [CrossRef]
66. Semida WM, Rady MM. Presoaking application of propolis and maize grain extracts alleviates salinity stress in common bean (Phaseolus vulgaris L.). Sci Hortic. 2014;168:210–7. doi:10.1016/j.scienta.2014.01.042. [Google Scholar] [CrossRef]
67. Lesk C, Anderson W, Rigden A, Coast O, Jägermeyr J, McDermid S, et al. Compound heat and moister extreme impacts on global crop yield under climate change. Nat Revi Earth Envir. 2022;3(12):872–89. doi:10.1038/s43017-022-00368-8. [Google Scholar] [CrossRef]
68. Sgobba A, Paradiso A, Dipierro S, de Gara L, de Pinto MC. Changes in antioxidants are critical in determining cell responses to short-and long-term heat stress. Physiol Plant. 2015;153(1):68–78. doi:10.1111/ppl.2015.153.issue-1. [Google Scholar] [CrossRef]
69. Ahammed GJ, Xu W, Liu A, Chen S. Endogenous melatonin deficiency aggravates high temperature-induced oxidative stress in Solanum lycopersicum L. Environ Exp Bot. 2019;161:303–11. doi:10.1016/j.envexpbot.2018.06.006. [Google Scholar] [CrossRef]
70. Batool S, Khan S, Basra SMA. Foliar application of moringa leaf extract improves the growth of moringa seedling in winter. S Ari J Bot. 2020;129:347–53. doi:10.1016/j.sajb.2019.08.040. [Google Scholar] [CrossRef]
71. Taşgín E, Atící Ö., Nalbantoğlu B. Effects of salicylic acid and cold on freezing tolerance in winter wheat leaves. Plant Growth Regul. 2003;41(3):231–6. doi:10.1023/B:GROW.0000007504.41476.c2. [Google Scholar] [CrossRef]
72. Shahid M, Pourrut B, Dumat C, Nadeem M, Aslam M, Pinelli E. Heavy-metal-induced reactive oxygen species: phytotoxicity and physicochemical changes in plants. Rev Environ Contam T. 2014;232:1–44. [Google Scholar]
73. Mao F, Nan G, Cao M, Gao Y, Guo L, Meng X, et al. The metal distribution and the change of physiological and biochemcial process in soybean and mung bean plants under heavy metal stress. Int J Phytoremediat. 2018;20(11):1113–20. doi:10.1080/15226514.2017.1365346. [Google Scholar] [PubMed] [CrossRef]
74. Cong W, Miao Y, Xu L, Zhang Y, Yuan C, Wang J, et al. Transgenerational memory of gene expression changes induced by heavy metal stress in rice (Oryza sativa L.). BMC Plant Biol. 2019;19:282. doi:10.1186/s12870-019-1887-7. [Google Scholar] [PubMed] [CrossRef]
75. Sytar O, Kumari P, Yadav S, Brestic M, Rastogi A. Phytohormone priming: regulator for heavy metal stress in plants. J Plant Growth Regul. 2019;38:739–52. doi:10.1007/s00344-018-9886-8. [Google Scholar] [CrossRef]
76. Kerdsomboon K, Tatip S, Kosasih S, Auesukaree C. Soluble Moringa oleifera leaf extract reduces intracellular cadmium accumulation and oxidative stress in Saccharomyces cerevisiae. J Biosci Bioeng. 2016;121(5):543–9. doi:10.1016/j.jbiosc.2015.09.013. [Google Scholar] [PubMed] [CrossRef]
77. Kerdsomboon K, Chumsawat W, Auesukaree C. Effects of Moringa oleifera leaf extracts and its bioactive compound gallic acid on reducing toxicities of heavy metals and metalloid in Saccharomyces cerevisiae. Chemosphere. 2021;270:128659. doi:10.1016/j.chemosphere.2020.128659. [Google Scholar] [PubMed] [CrossRef]
78. Zhang F, Zhang H, Xia Y, Wang G, Xu L, Shen Z. Exogenous application of salicylic acid alleviates cadmium toxicity and reduces hydrogen peroxide accumulation in root apoplasts of Phaseolus aureus and Vicia sativa. Plant Cell Rep. 2011;30:1475–83. doi:10.1007/s00299-011-1056-4. [Google Scholar] [PubMed] [CrossRef]
79. Kotapati KV, Palaka BK, Ampasala DR. Alleviation of nickel toxicity in finger millet (Eleusine coracana L.) germinating seedlings by exogenous application of salicylic acid and nitric oxide. Crop J. 2017;5(3):240–50. doi:10.1016/j.cj.2016.09.002. [Google Scholar] [CrossRef]
80. Koprivova A, Kopriva S. Plant secondary metabolites altering root microbiome composition and function. Curr Opin Plant Biol. 2022;67:102227. doi:10.1016/j.pbi.2022.102227. [Google Scholar] [PubMed] [CrossRef]
81. Renaut S, Masse J, Norrie JP, Blal B, Hijri M. A commercial seaweed extract structured microbial communities associated with tomato and pepper roots and significantly increased crop yield. Microb Biotechnol. 2019;12(6):1346–58. doi:10.1111/mbt2.v12.6. [Google Scholar] [CrossRef]
82. Ramakrishna W, Yadav R, Li K. Plant growth promoting bacteria in agriculture: two sides of a coin. Appl Soil Ecol. 2019;138:10–8. doi:10.1016/j.apsoil.2019.02.019. [Google Scholar] [CrossRef]
83. Zhao D, Zhao H, Zhao D, Zhu X, Wang Y, Duan Y, et al. Isolation and identification of bacteria from rhizosphere soil and their effect on plant growth promotion and root-knot nematode disease. Biol Control. 2018;119:12–9. doi:10.1016/j.biocontrol.2018.01.004. [Google Scholar] [CrossRef]
84. Barros-Rodríguez A, Rangseekaew P, Lasudee K, Pathom-Aree W, Manzanera M. Regulatory risks associated with bacteria as biostimulants and biofertilizers in the frame of the European Regulation (EU) 2019/1009. Sci Total Environ. 2020;740:140239. doi:10.1016/j.scitotenv.2020.140239. [Google Scholar] [PubMed] [CrossRef]
85. Douglas AE. Strategies for enhanced crop resistance to insect pests. Annu Rev Plant Biol. 2018;69:637–60. doi:10.1146/arplant.2018.69.issue-1. [Google Scholar] [CrossRef]
86. Aremu AO, Masondo NA, Rengasamy KR, Amoo SO, Gruz J, Bíba O, et al. Physiological role of phenolic biostimulants isolated from brown seaweed Ecklonia maxima on plant growth and development. Planta. 2015;241(6):1313–24. doi:10.1007/s00425-015-2256-x. [Google Scholar] [PubMed] [CrossRef]
87. Liu X, Cao A, Yan D, Ouyang C, Wang Q, Li Y. Overview of mechanisms and uses of biopesticides. Int J Pest Manage. 2019;67(1):65–72. [Google Scholar]
88. Jones JG, Korir RC, Walter TL, Everts KL. Reducing chlorothalonil use in fungicide spray programs for powdery mildew, anthracnose, and gummy stem blight in melons. Plant Dis. 2020;104(12):3213–20. doi:10.1094/PDIS-04-20-0712-RE. [Google Scholar] [PubMed] [CrossRef]
89. Choudhury D, Anand YR, Kundu S, Nath R, Kole RK, Saha J. Effect of plant extracts against sheath blight of rice caused by Rhizoctonia solani. J Pharm Phytoch. 2017;6(4):399–404. [Google Scholar]
90. Nichols V, Verhulst N, Cox R, Govaerts B. Weed dynamics and conservation agriculture principles: a review. Field Crop Res. 2015;183:56–68. doi:10.1016/j.fcr.2015.07.012. [Google Scholar] [CrossRef]
91. Hasan M, Ahmad-Hamdani MS, Rosli AM, Hamdan H. Bioherbicides: an eco-friendly tool for sustainable weed management. Plants. 2021;10(6):1212. doi:10.3390/plants10061212. [Google Scholar] [PubMed] [CrossRef]
92. Aktar W, Sengupta D, Chowdhury A. Impact of pesticides use in agriculture: their benefits and hazards. Inter Toxic. 2009;2(1):1–12. doi:10.2478/v10102-009-0001-7. [Google Scholar] [PubMed] [CrossRef]
93. Masi M, Pannacci E, Santoro E, Zermane N, Superchi S, Evidente A. Stoechanones A and B, phytotoxic copaane sesquiterpenoids isolated from Lavandula stoechas with potential herbicidal activity against Amaranthus retroflexus. J Nat Prod. 2020;83(5):1658–65. doi:10.1021/acs.jnatprod.0c00182. [Google Scholar] [PubMed] [CrossRef]
94. Bhadoria PBS. Allelopathy: a natural way towards weed management. Am J Exp Agr. 2011;1(1):7–20. [Google Scholar]
95. Travaini ML, Sosa GM, Ceccarelli EA, Walter H, Cantrell CL, Carrillo NJ, et al. Khellin and visnagin, furanochromones from Ammi visnaga (L.) Lam., as potential bioherbicides. J Agr Food Chem. 2016;64(50):9475–87. doi:10.1021/acs.jafc.6b02462. [Google Scholar] [PubMed] [CrossRef]
96. Puig CG, Reigosa MJ, Valentao P, Andrade PB, Pedrol N. Unravelling the bioherbicide potential of Eucalyptus globulus Labill: biochemistry and effects of its aqueous extract. PLoS One. 2018;13(2):e0192872. doi:10.1371/journal.pone.0192872. [Google Scholar] [PubMed] [CrossRef]
97. Chon SU, Kim YM, Lee JC. Herbicidal potential and quantification of causative allelochemicals from several compositae weeds. Weed Res. 2002;43(6):444–50. [Google Scholar]
98. Kato-Noguchi H, Suzuki M, Noguchi K, Ohno O, Suenaga K, Laosinwattana C. A potent phytotoxic substance in Aglaia odorata Lour. Chem Biodivers. 2016;13(5):549–54. doi:10.1002/cbdv.201500175. [Google Scholar] [PubMed] [CrossRef]
99. Goñi O, Quille P, O’Connell S. Ascophyllum nodosum extract biostimulants and their role in enhancing tolerance to drought stress in tomato plants. Plant Physiol Bioch. 2018;126:63–73. doi:10.1016/j.plaphy.2018.02.024. [Google Scholar] [PubMed] [CrossRef]
100. Jiao P, Jiang Z, Wei X, Liu S, Qu J, Guan S, et al. Overexpression of the homeobox-leucine zipper protein ATHB-6 improves the drought tolerance of maize (Zea mays L.). Plant Sci. 2022;316:111159. doi:10.1016/j.plantsci.2021.111159. [Google Scholar] [PubMed] [CrossRef]
101. Fan D, Hodges DM, Critchley AT, Prithiviraj B. A commercial extract of brown macroalga (Ascophyllum nodosum) affects yield and the nutritional quality of spinach in vitro. Commun Soil Sci Plan. 2013;44(12):1873–84. doi:10.1080/00103624.2013.790404. [Google Scholar] [CrossRef]
102. Yakhin O, Lubyanov AA, Yakhin IA, Brown PH. Biostimulants in plant science: a global perspective. Front Plant Sci. 2017;7:2049. [Google Scholar] [PubMed]
103. Pereira LB, Thomazella DP, Teixeira PJ. Plant-microbiome crosstalk and disease development. Curr Opin Plant Biol. 2023;72:102351. doi:10.1016/j.pbi.2023.102351. [Google Scholar] [PubMed] [CrossRef]
104. Bakker PA, Pieterse CM, de Jonge R, Berendsen RL. The soil-borne legacy. Cell. 2018;172(6):1178–80. doi:10.1016/j.cell.2018.02.024. [Google Scholar] [PubMed] [CrossRef]
105. El Boukhari MEM, Barakate M, Bouhia Y, Lyamlouli K. Trends in seaweed extract based biostimulants: manufacturing process and beneficial effect on soil-plant systems. Plants. 2020;9(3):359. doi:10.3390/plants9030359. [Google Scholar] [PubMed] [CrossRef]
106. Getzke F, Wang L, Chesneau G, Böhringer N, Mesny F, Denissen N, et al. Physiochemical interaction between osmotic stress and a bacterial exometabolite promotes plant disease. Nat Commun. 2024;15(1):4438. doi:10.1038/s41467-024-48517-5. [Google Scholar] [PubMed] [CrossRef]
Cite This Article
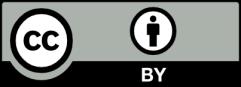
This work is licensed under a Creative Commons Attribution 4.0 International License , which permits unrestricted use, distribution, and reproduction in any medium, provided the original work is properly cited.