Open Access
ARTICLE
Response of Bacterial Community and Enzyme Activity of Greenhouse Tomato under Different Irrigation Systems
1 Faculty of Engineering, Huanghe Science and Technology University, Zhengzhou, 450063, China
2 Northwest Land and Resources Research Center, Shaanxi Normal University, Xi’an, 710119, China
* Corresponding Author: Mingzhi Zhang. Email:
Phyton-International Journal of Experimental Botany 2024, 93(7), 1543-1568. https://doi.org/10.32604/phyton.2024.050915
Received 22 February 2024; Accepted 24 May 2024; Issue published 30 July 2024
Abstract
The micro-sprinkler irrigation mulched (MSM) has been suggested as a novel water-saving approach in controlled environment agriculture. However, the effects of microbial community structure and enzyme activity in the rhizosphere soil on crop growth under MSM remain unclear. This study conducted a randomized experimental design using greenhouse tomatoes to investigate changes in bacterial community structure and enzyme activity in rhizosphere soil under different irrigation frequencies (F) and amounts (I) of MSM. The findings revealed that with the increase of F or I, The total count of soil bacteria in tomatoes first rose and then fell in terms of Operational Taxonomic Units (OTUs) classification. Compared to other F, the most abundance of nitrogen and phosphorus metabolism genes and enzyme activities were observed with a 5-day F. Moreover, the diversity of soil bacterial community structure initially rose before eventually declining with the increase of the I. Applying 1.00 Epan (cumulative evaporation of a 20 cm standard pan) under MSM helped boost the abundance of nitrogen and phosphorus metabolism functional genes in soil bacteria, ensuring higher enzyme activities related to nitrogen, carbon, and phosphorus metabolism in the rhizosphere soil of tomatoes. Tomatoes’ yield initially rose before eventually declining with the increase in F or I, whereas I had a more significant effect on yield. A 1.00% increase in I yielded a minimum of 39.24% increase in tomato yield. The study showed a positive correlation between soil bacterial community, soil enzyme activity, and greenhouse tomato yield under MSM. Considering the results comprehensively, the combined irrigation mode of F of 5 d and I of 1.00 Epan was recommended for greenhouse tomatoes under MSM. This conclusion provides theoretical support for water-saving practices and yield improvement in facility agriculture, especially tomato cultivation.Keywords
Nomenclature
F | Irrigation frequency |
I | Irrigation amount |
A | Plot area |
Epan | Evaporation rate |
Kcp | Crop-pan coefficient |
K05306 | KEGG orthology number of a gene |
PhnX | Gene name |
ACE | ACE index of soil bacteria |
CHAO | CHAO index of soil bacteria |
SOBS | SOBS index of soil bacteria |
OTUs | Operational Taxonomic Units |
PCoA | Principal Coordinate Analysis |
BG | β-Glucosidase activity |
LAP | Leucine aminopeptidase activity |
NAG | N-acetyldglucosaminidasealk |
AP | Aline phosphatase activity |
U/g | Unit of enzyme activity |
Y | Yield |
NMGA | Soil bacterial nitrogen metabolism function gene abundance |
PMGA | Phosphorus metabolism function gene abundance |
* | p ≤ 0.05 |
** | p ≤ 0.01 |
ns | p > 0.05 |
The rapid growth of crops and heavy use of soil water and fertilizer in facility agriculture have sparked a significant debate on optimizing the efficient use of these resources [1,2]. Soil microorganisms, a crucial component of the agroecosystem, play a significant role in energy and matter circulation [3]. Soil bacteria, with their high diversity, abundance, and functional completeness, are key in regulating soil microbial communities and nutrient cycles [4,5]. Previous research has indicated that soil bacterial diversity and heterogeneity are valuable indicators of soil fertility and environmental risks [6,7]. These factors are strongly influenced by soil physical and chemical properties, root development, litter content, and other factors [8,9]. In the Huang-Huai-Hai Plain, environmental variables explain nearly a quarter of the spatial variation in soil bacterial communities [10]. Therefore, studying the soil microbial community structure in facility agriculture is essential for sustainable agricultural development.
The main nutrients essential for crop growth are soil carbon, nitrogen, and phosphorus, with their cycling largely dependent on soil enzymes [11,12]. Soil enzyme activity is intricately linked to various factors such as crop root development, soil microbial activity, and nutrient levels [13,14]. Soil-glucosidase (BCG) plays a crucial role in soil carbon transformation, while Soil leucine aminopeptidase (LAP) and Soil N-acetyl glucosaminidase (NAG) are key players in the soil nitrogen cycle. Soil alkaline phosphatase (AP) is pivotal in the conversion of soil organic phosphorus. Consequently, numerous studies have been conducted on soil enzymes like BCG, LAP, NAG, and AP [15]. Hence, research on soil enzyme activity holds significant importance in enhancing soil nutrient cycling and promoting the uptake and utilization of nutrients in precision agriculture.
Proper irrigation management practices play a significant role in changing the distribution of soil water and heat, which directly affects the soil bacterial community and soil enzyme activity. Researchers have suggested different techniques to improve the resilience of the soil bacterial community structure and enzyme activity, including microbial inoculation and the use of growth regulators [16]. However, implementing these methods faces challenges such as high costs, environmental issues, and low awareness [17,18]. Some researchers have proposed optimizing soil microbial community structure and enzyme activity through regulated irrigation management. The way farmland is managed greatly affects the variety and numbers of soil microbial communities [19,20]. Studies have demonstrated that alterations in irrigation frequency and techniques can directly impact soil moisture levels. Furthermore, fluctuations in soil water content significantly influence soil bacterial communities and enzyme activity [21–23]. A stable soil bacterial community structure and high enzyme activity can enhance nutrient cycling, root development, and the accumulation of carbon and nitrogen in crop rhizospheres [24]. The positive relationships between crop roots, soil microorganisms, and enzyme activities are crucial for achieving stable crop yields [25–27]. Hence, it is crucial to study the impact of different irrigation management techniques on soil nutrient utilization, microbial community structure, and enzyme activity in order to comprehend agricultural water conservation and increase crop yields by optimizing soil nutrient utilization.
Micro-sprinkler irrigation mulched (MSM) is an innovative water-saving technology in controlled agriculture that it has demonstrated successful outcomes with greenhouse-grown cucumbers, celery, and watermelon [28–30]. Previous research has primarily concentrated on drip irrigation when examining how irrigation management practices impact soil bacterial community structure and enzyme activity. However, there are few studies on how MSM with unique soil wetlands affects these aspects. The effects of bacterial community structure and enzyme activity in the rhizosphere soil of crop growth under MSM remain unclear.Moreover, there is an absence of both qualitative and quantitative analysis regarding the connections between soil bacterial community structure, soil enzyme activity, and tomato yield in the presence of MSM. The objective of this study was to examine how different irrigation frequency and amount on bacterial communities, soil enzyme activity, and yield of greenhouse tomatoes under MSM conditions. By conducting experiments and mathematical analysis, theoretical support for water-saving practices and yield enhancement methods in facility agriculture were provided, particularly in tomato cultivation.
2.1 Experimental Site and Management
The research was carried out in a greenhouse situated at the Modern Agricultural Science and Technology Exhibition Center in Xi’an, Shaanxi Province, China (108°52′E, 34°03′N). The experimental greenhouse had a ridge planting structure and measured 85 m in length and 15 m in width [19]. The planting schedule for both spring and autumn tomatoes can be found in Table 1.
In this study, greenhouse tomatoes were irrigated by Micro-sprinkler irrigation under mulched (MSM) [31]. MSM is a micro-irrigation technology that combines micro-sprinkler irrigation with plastic film technology and uses multiple sets of micro-hole outflows under plastic film for irrigation.
Two factors of irrigation frequency and irrigation amount were set in the experiment.
Irrigation frequency (F) was set to 3 levels: 3 d (F1), 5 d (F2) and 7 d (F3).
The irrigation amount (I) was calculated according to the cumulative evaporation from a 20 cm Class A pan(DY.AM3) (Weifang Dayu Hydrology Technology Co., Ltd. in Shandong, China). I was controlled by Epan. Different I were applied by controlling crop-pan coefficient Kcp. Kcp was set to 3 levels: 0.70 (I1),1.00 (I2), and 1.20 (I3).
In this experiment, there were 9 treatments (Table 2).
At 08:00 AM, the evaporation rate (Epan) was recorded for each F before initiating irrigation. Eq. (1) was utilized to calculate the required I [24]. The irrigation details for tomatoes can be observed in Fig. 1.
(1)
Figure 1: Irrigation amount and dates for greenhouse tomatoes
In Eq. (1), W denotes the I, while A denotes the plot area.
2.3 Methods of Measurement and Computation
Samples of soil were gathered from the rhizosphere surrounding of tomato plants 72 days after planting. The soil shaking method was used to extract the soil by excavating a cylindrical section of soil around the tomato roots within a 20 cm radius and at a depth of 5–25 cm, then shaking off any loose soil attached to the roots. The soil tightly bound to the roots was delicately brushed off with a sterilized soft brush, representing the rhizosphere soil of greenhouse tomatoes. Three rhizosphere soil samples were randomly chosen from each experimental plot and taken to the laboratory immediately. Any fresh plant residues found in the samples were eliminated. Composite soil samples were created for sequencing within the experimental area by thoroughly mixing and homogenizing three soil samples from each plot. The soil samples were frozen quickly using liquid nitrogen upon collection. Subsequently, the frozen samples were stored in a −80°C freezer and sent to Shanghai Meiji Biomedical Technology Co., Ltd. (Shanghai, China) for the analysis of soil bacterial communities. The assessment of soil-related parameters was finalized within a span of 10 days.
2.3.2 Analysis of Soil Bacterial Community
The assessment of the soil bacterial community was carried out in four stages:
A. DNA extraction and PCR amplification
DNA was extracted from the rhizosphere soil of tomatoes using the E.Z.N.A. soil kit from Omega Bio-tek, located in Norcross, GA, USA. This kit is highly effective in extracting high-quality genomic DNA from various soil samples, with the ability to process up to 1 g of soil in 60 min. After confirming DNA extraction quality through 1% agarose gel electrophoresis, the PCR was used to amplify the V3-V4 variable region using 338 F and 806 R primers. The PCR amplification process involved an initial pre-denaturation step at 95°C for 3 min, followed by 27 cycles of denaturation at 95°C for 30 s, annealing at 55°C for 30 s, extension at 72°C for 30 s, and a final extension step at 72°C for 10 min using the ABI GeneAmp 9700 PCR instrument.
B. Sequencing using Illumina MiSeq
The amplification primers used for targeting the bacterial 16S rDNA V3-V4 region were 338 F (5′-ACTCCTACGGGGAGGCAGCAG-3′) and 806 R (5′-GGACTACNNGGGTATCTAAT-3′). Following amplification, the PCR products were retrieved from a 2% agarose gel, purified, and eluted for further analysis. The PCR reaction mixture (with a total volume of 25 μL) comprised 12.5 μL of KAPA 2G Robust Hot Start Ready Mix, 1 μL of Forward Primer (5 mol/L), 1 μL of Reverse Primer (5 mol/L), 5 μL of DNA (30 ng total), and 5.5 μL of ddH2O. The reaction conditions involved an initial denaturation at 95°C for 5 min, followed by 28 cycles consisting of denaturation at 95°C for 45 s, annealing at 55°C for 50 s, and extension at 72°C for 45 s. A final extension step was carried out at 72°C for 10 min.
C. Processing data for sequencing
The original FASTQ files were separated by sample using a custom perl script, then filtered for quality using fastp version 0.19.6, and finally combined using FLASH version 1.2.7 based on specific criteria.
(i) Reads with an average quality score of <20 over a 50 bp sliding window were truncated at any site, and those shorter than 50 bp were excluded, as well as reads containing ambiguous characters. (ii) Overlapping sequences longer than 10 bp were exclusively assembled based on their overlap with a maximum mismatch ratio of 0.2 in the overlap region. Any unassembleable reads were removed. (iii) Samples were distinguished by barcodes and primers, adjusting sequence direction with exact barcode matching and allowing a 2-nucleotide mismatch in primer matching. Subsequently, the optimized sequences were binned into operational taxonomic units (OTUs) at a 97% sequence similarity level using UPARSE 7.1. The most prevalent sequence in each OTU was designated as a representative. To mitigate the impact of sequencing depth on alpha and beta diversity analysis, the number of 16S rRNA gene sequences per sample was rarefied to 20,000, ensuring an average coverage of 99.09%.
The taxonomic classification of each Operational Taxonomic Unit (OTU) representative sequence was assessed using RDP Classifier version 2.2 against the 16S rRNA gene database (e.g., Silva v138) with a confidence threshold of 0.7. Metagenomic functions were predicted through PICRUSt2 (Phylogenetic Investigation of Communities by Reconstruction of Unobserved States) based on these OTU representative sequences. PICRUSt2 is a software that includes several tools: HMMER was utilized to align OTU representative sequences with reference sequences, EPANG and Gappa placed OTU representative sequences on a reference tree, Castor normalized the 16S gene copies, and Min-Path predicted gene family profiles and identified gene pathways. The entire analytical procedure adhered to the guidelines established by PICRUSt2.
D. Analyzing statistics through sequencing.
The soil microbiota was analyzed using the Majorbio Cloud platform in Shanghai, China (https://login.majorbio.com/login, accessed on 01/04/2024). OTUs information, rarefaction curves, and alpha diversity indices (including observed OTUs, ACE index, CHAO index, and SOBS index) of soil bacteria were calculated with Mothur v1.30.1. Venn diagrams were utilized to identify common and unique species OTUs across different groups or samples. Samples with a 97% similarity at the OTU taxonomic level were chosen for analysis. The species composition histogram at the genus level for various treated soil samples was generated using the R language (version 3.3.1) tool and data from the tax_summary_folder of the Meiji Bio-cloud platform. Dominant species at the genus level and their relative abundances in each sample were determined from the histogram. Principal coordinate analysis (PCoA) based on Bray-Curtis dissimilarity using the Vegan v2.5-3 package was employed to assess the similarity among microbial communities in different samples.
The analysis made use of the Kyoto Encyclopedia of Genes and Genomes along with pertinent references [32] to identify functional genes in soil bacteria. By emphasizing their functional roles, it becomes possible to efficiently pinpoint the key genes specific to each function and trace the pathways leading to these particular genes. Statistical methods were then used to analyze the abundance of these selected functional genes. The study focused on determining the functional genes related to nitrogen metabolism in soil bacteria by combining those associated with nitrogen fixation, nitrification, and denitrification. Table 3 displays the functional genes connected with soil bacteria.
2.3.3 Determination of Soil Enzyme Activity
Soil β-glucosidase enzyme activity (BG), soil leucine aminopeptidase activity (LAP), and soil N-acetylglucosaminidase (NAG) were assessed through fluorescence analysis using a 96-well microplate reader (RT-6100, Shanghai Precision Instrument Co., Ltd., Shanghai, China). Fluorescence readings were obtained using a multifunctional microplate reader at wavelengths of 365 and 460 nm [33,34]. The soil samples were thawed from −20°C to 4°C for revival. A total of 2.75 g of fresh soil sample was mixed with 91 mL of Tris-Buffer (pH 8, mimicking the soil pH) and placed on a 200 r/min rotary shaker (at 25°C) for 2 h. Following homogenization, 200 μL of the soil suspension was transferred to a 96-well microplate, and 50 μL of 10 mmol/L substrate was added. This procedure included sample controls (50 μL Tris-Buffer + 200 μL soil suspension), substrate controls (50 μL substrate + 200 μL Tris-Buffer), and fluorescence standard solutions (50 μL fluorescence standard solution + 200 μL Tris-Buffer). Each sample was then incubated in darkness at 25°C for 4 h before being analyzed with the multifunctional microplate reader.
The alkaline phosphatase activity (AP) in the soil suspension was measured by extracting it and using disodium phenyl phosphate colorimetry. To determine the enzyme activity, a standard curve was created using the absorbance of the gradient of pnitrophenol concentrations. Expressed in units of mg/g/d, the enzyme activity indicated the quantity of phenol that was extracted from 1 g of soil over a 24-h period [35].
2.3.4 Determination of Root-Related Indexes
Seventy-six and seventy-eight days after planting spring and autumn tomatoes, Three plants with a depth of about 0.4 m and a diameter of 0.2 m were randomly excavated from each plot. The soil samples were sieved and then scanned with an Epson Perfection V700 scanner to produce TIF images. The TIF images were analyzed using Win RHIZO Pro software to determine the total root length, total number of root tips, and number of bifurcations of tomatoes in the greenhouse. The root activity was evaluated using the triphenyl tetrazolium chloride method [36]. The procedure involved drying the root sample, extracting it with 95% ethanol to dissolve the red substance, removing impurities through centrifugation and ethanol washing, fixing the volume to 10 ml, measuring the optical density with a spectrophotometer at a wavelength of 485 nm after centrifugation, and calculating the reduction amount of tetrazole based on a standard curve.
In each experimental plot, 4 tomatoes were randomly selected and the weight of mature tomatoes with 4 ears was measured for each one. The yield of ripe fruit was assessed using a precise 0.01 g electronic balance. The total weight of the four mature tomato fruits was recorded as the plant’s yield, and the yield per hectare was calculated through conversion.
The Cobb-Douglas model was utilized to both qualitatively and quantitatively depict the impact of F and I on tomato yield. The relationship between soil bacterial community structure, soil enzyme activity, and tomato yield was quantitatively explained through Pearson correlation and regression analyses. Significance testing was conducted using the F test in SPSS 22.0 (IBM Crop., Armonk, New York, NY, USA) with a significance level set at p < 0.05. Pearson’s two-tailed test was performed using SPSS 22.0 (IBM Crop., Armonk, New York, NY, USA). Graphs were generated by OriginPro 2019 (Origin Lab Corporation, Northampton, MA, USA), while Excel 2016 was employed for regression analysis, which was Microsoft Excel 2016 (Microsoft, Washington DC, USA).
3.1 Effects of Different Treatments on Soil Bacterial Community Structure Diversity and Species Composition of Greenhouse Tomatoes
3.1.1 Bacterial Community Structure Diversity
Irrigation frequency (F) had significant effects on soil bacterial community structure diversity ACE index, CHAO index and SOBS index of spring tomatoes and autumn tomatoes (Fig. 2). One-way analysis of variance showed that the ACE index of soil bacteria in F2I2 treatment of spring tomato and autumn tomato was higher than that of F1I1, F1I2, F1I3, F2I1, F3I1 and F3I2 by about 30.73% and 17.36%, 14.69% and 2.95%, 20.97% and 7.78%, 20.90% and 1.62%, 44.49% and 45.25%, 5.22% and 14.14%, respectively. The irrigation frequency (F) and amount (I) had a significant impact on the diversity of soil bacterial community structure in spring and autumn tomatoes (Fig. 2). Results from one-way analysis of variance revealed that the ACE index for soil bacteria under the F2I2 treatment was higher in spring and autumn tomatoes F1I1, F1I2, F1I3, F2I1, F3I1, and F3I2 by approximately 30.73% and 17.36%, 14.69% and 2.95%, 20.97% and 7.78%, 20.90% and 1.62%, 44.49% and 45.25%, 5.22% and 14.14%, respectively. The soil bacterial CHAO index of F2I2 treatment was higher than that of F1I1, F1I2, F1I3, F2I1, F3I1, F3I2 and F3I3 by about 30.45% and 17.38%, 14.01% and 2.45%, 20.32% and 7.94%, 17.59% and 1.68%, 44.05% and 46.74%, 5.10% and 12.75%, 5.38% and 10.07%, respectively. The SOBS index of soil bacteria in F2I2 treatment was higher than that of F1I1, F1I2, F1I3, F2I1, F3I1, F3I2 and F3I3 by about 35.00% and 22.34%, 18.69% and 3.01%, 25.17% and 6.21%, 19.72% and 5.27%, 50.40% and 57.25%, 4.03% and 16.35%, 6.10% and 11.91%, respectively.
Figure 2: Effects of Irrigation schemes on the diversity of soil bacterial community structure diversity. F represents irrigation frequency; I represents irrigation amount; The data in the figure are displayed as the mean and standard deviation. If different letters are present in the same color column, it indicates a significant difference at the 0.05 level; the same below. The ACE, CHAO, and SOBS represent the ACE index, CHAO index, and SOBS index of soil bacteria, respectively
When keeping I constant, as F decreases, the diversity of soil bacterial community structure shown by ACE index, CHAO index, and SOBS index in spring and autumn tomatoes under MSM initially increases and then decreases. Likewise, when maintaining the F constant, as I increases, the ACE index, CHAO index, and SOBS index of soil bacterial community structure diversity in spring and autumn tomatoes under MSM also exhibit an initial increase followed by a decrease.
3.1.2 Bacterial Community Structure Species Composition
Within the soil bacteria community of tomatoes treated with 9 treatments, there were 1287 and 1787 OTUs in common, representing 24.48% and 26.56% of the total OTUs, respectively (see Fig. 3). The interaction analysis revealed that treatment F2I2 had the most soil bacteria communities, with 3548 OTUs in spring tomatoes and 4710 OTUs in autumn tomatoes.
Figure 3: Effect of irrigation scheme on soil bacterial community at OTUs classification level. F represents irrigation frequency; I represents irrigation amount
When the I was held constant, the overall soil bacterial communities in tomatoes initially increased and then decreased as the F decreased at the OTUs classification level. Likewise, when F was held constant, the total soil bacterial communities in both spring and autumn tomatoes initially increased and then decreased as the I was increased at the OTUs classification level.
Fig. 4 illustrates that the predominant bacterial populations in the spring tomato soil at the genus level were mainly Bacillus (9.22%–25.11%) and Streptomyces (1.58%–3.39%). In contrast, the dominant bacterial populations in the autumn tomato soil were mainly Sphingomonas (5.46%–10.98%) and Bacillus (2.50%–5.88%). Bacillus is a common presence in tomato soil. As the F decreased, the abundance of bacterial Bacillus initially increased and then decreased in tomato soil. Specifically, the abundance of bacterial Bacillus in F2 of spring and autumn tomatoes was significantly higher than that of F3 by approximately 1.35% and 26.15%, respectively. With the increase of I, the bacterial Bacillus abundance in tomato soil decreased. Among them, the soil bacterial Bacillus abundance of I2 was 7.71% and 5.14% higher than that of I1.
Figure 4: Effects of irrigation scheme on the genus level of soil bacteria community. The “others” category included species that were merged due to having a species abundance of less than 1.00%. The vertical axis depicted different treatments, while the horizontal axis represented the proportion of soil bacterial populations at the genus level
Fig. 5 demonstrates that the combined contribution rate of PC1 and PC2 surpassed 52.00%. Within the positive range of PC1 and PC2, the F2I2 treatment emerged as the most effective population density of soil bacteria in tomatoes at the genetic level.
Figure 5: Effects of irrigation scheme on the soil bacteria community at the genus level by Principal Coordinate Analysis (PCoA). F represents irrigation frequency; I represents irrigation amount
3.2 Analysis of Functional Genes Related to Nitrogen and Phosphorus Metabolism in Soil Bacteria
As shown in Fig. 6, the combination of F and I had a significant influence on the abundance of nitrogen metabolism function genes (NFGA), and phosphorus metabolism function genes (PMGA) in tomato soil. Comparing tomatoes treated with F2I2 with other treatments, the NFGA, NGA, DFGA, NMGA, and PMGA were not significantly lower than F2I3, but were higher than F1I1, F1I2, F1I3, F2I1, F3I1, F3I2, and F3I3 in both spring and autumn tomato soil. As F decreased under constant I, the levels of NFGA, NGA, DFGA, NMGA, and PMGA in the soil of tomatoes initially increased and then fell, the bacterial community structure diversity and the abundance of functional genes linked to bacterial nitrogen and phosphorus metabolism were highest in the rhizosphere soil of both spring and autumn tomatoes when using F2 irrigation frequency. Conversely, under the condition of the same F, the NFGA, NGA, DFGA, NMGA, and PMGA of soil bacteria increased with higher levels of I, the bacterial community structure diversity and the abundance of functional genes linked to bacterial nitrogen and phosphorus metabolism were highest in the rhizosphere soil of both spring and autumn tomatoes when using I2 irrigation amount. The results showed that F2I2 treatment could improve the metabolism of nitrogen and phosphorus in soil bacteria.
Figure 6: Comparative analysis of the abundance of functional genes related to N and P metabolism bacteria under the regulation of irrigation regimes. F represents irrigation frequency; I represents irrigation amount. The data are shown as average ± standard deviation in the figure, different letters in the same color column meant significant difference at 0.05 level, the same as blow
3.3 Effects of Different Treatments on Soil Enzyme Activities of Greenhouse Tomatoes
Both F and I had significant effects on the BG, LAD, NAG, and AP in tomato soils (p ≤ 0.05) (Table 4). As the F decreased, the soil BG of tomatoes initially rose before eventually declining. This indicates that the soil BG of F2 was approximately 13.73% and 11.81% higher than that of F1, 31.72%, and 26.73% higher than that of F3. As the F decreased, the soil LAD of tomatoes initially rose before eventually declining. The soil LAD of spring tomatoes and autumn tomatoes F2 was higher than that of F1, and F3 by about 20.60% and 15.11%, 42.83%, and 29.89%, respectively. As the F decreased, the soil NAG of tomatoes initially rose before eventually declining. The soil NAG of F2 of spring tomatoes and autumn tomatoes was higher than that of F1, and F3, by 20.92% and 30.06%, respectively. The soil AP levels for tomatoes initially rose before eventually declining as the F decreased. This indicates that the soil AP for tomatoes under F2 was higher than F1 and F3.
As the I increased, the soil BG of tomatoes initially rose before eventually declining. The soil BG of spring tomatoes and autumn tomatoes I2 was higher than that of I1 and I3 by about 15.85% 13.64%, 1.83%, and 2.00%, respectively. As the I increased, the soil LAD of tomatoes initially rose before eventually declining. The soil LAD of spring tomatoes and autumn tomatoes I2 was higher than that of I1, and I3 by about 17.88% and 12.93%, −2.32%, and 2.43%, respectively. As the I increased, the soil NAG of tomatoes initially rose before eventually declining. The soil NAG of spring tomatoes and autumn tomatoes I2 was higher than that of I1, and I3 by about 17.28% and 19.57%, −0.48% and 1.93%, respectively. With the increase of I, the soil AP of tomatoes initially rose before eventually declining. The soil AP of tomatoes I2 was higher than that of I1, and I3.
3.4 Effect of Different Treatments on Yield of Greenhouse Tomatoes
Table 5 displays the results of a one-way analysis of variance, indicating that the yields of tomatoes in both spring and autumn under the F2I2 were notably greater than F1I1, F2I1, F3I1, F3I2, and F3I3 treatments by approximately 33.44% and 31.00%, 31.88%, and 28.03%, 44.08% and 43.38%, 27.49% and 16.73%, 21.04% and 32.03%, respectively. the yield of tomatoes initially rose before eventually declining with the decrease of F. The F2 of tomatoes in both spring and autumn yields were higher than F1, and F3 by about 5.27% and 3.24%, 19.31%, and 11.30%. With the increase of I, the yield of tomatoes initially rose before eventually declining. The yield of I2 tomatoes in both spring and autumn was higher than that of I1 and I3. To further analyze this relationship quantitatively, the Cobb-Douglas model was utilized, with F and I as independent variables and tomato yield as the dependent variable. The regression analysis results are presented in Table 6.
The formula above shows that in the MSM system, changes in I had a greater impact on the yield elasticity than changes in F. Specifically, a 1.00% increase in I resulted in a minimum 39.24% increase in tomato yield, while a 1.00% increase in F led to a roughly 11.23% decrease in tomato yield. The Cobb-Douglas model fitting results confirmed that the effects of F and I on tomato yield aligned with the conventional variance test.
3.5 Interaction among Soil Bacteria Community, Soil Enzyme Activity, and Yield under the Control of Irrigation Frequency and Irrigation Amount
Using Pearson’s two-tailed test, a positive relationship was noticed among soil bacterial community, soil enzyme activity, and yield. The strongest correlations were observed between soil bacterial community and soil enzyme activity, as well as between soil bacterial community structure diversity (CHAO index) and soil BG (0.898 and 0.805, for spring and autumn tomatoes). Soil bacteria’s NMGA and PMGA showed the highest correlations with LAP + NAG (0.923 and 0.797) and AP activity (0.948 and 0.770), respectively. To delve deeper into the interaction between soil bacterial community and soil enzyme activity, regression analysis was conducted, as shown in Fig. 7. In the correlation analysis between soil enzyme activity and yield, significant positive correlations were found for soil BG, LAP + NAG, AP, and yield (0.793 and 0.746, 0.847 and 0.843, 0.699 and 0.726). Multiple regression analysis was then utilized to further explain the variability in yield by quantitatively describing the relationship between soil enzyme activity and yield (Fig. 7).
Figure 7: Correlation between soil bacterial community and soil enzyme activity. Note: the NMGA, PMGA, CHAO, BG, LAP, NAG, and AP represent the abundance of soil bacterial nitrogen metabolism functional genes, the abundance of phosphorus metabolism functional genes, the CHAO index of soil bacteria, β-glucosidase activity, leucine aminopeptidase activity, N-acetylglucosaminidase, and alkaline phosphatase activity, respectively. The U/g represents the unit of enzyme activity
A quadratic parabolic relationship between CHAO and BG was observed with determination coefficients of R2 > 0.7265, R2 > 0.6122, and R2 > 0.8083 (see Fig. 7). The regression model showed a strong fit, allowing for the evaluation of the interplay between soil bacterial community and soil enzyme activity at this time. Additionally, the findings indicated a positive correlation between soil enzyme activity and yield, as detailed in Table 7.
The regression model demonstrated a satisfactory fit between soil enzyme activity and the yield of spring and autumn tomatoes (R2 > 0.8765) (Table 7). In other words, the combination of soil BG, LAP + NAG, and AP can account for over 87.65% of the variability in yield. The regression analysis of soil enzyme activity and yield can be used for the prediction of tomato yield and for assessing the production potential of the field.
4.1 Effects of Different Treatments on Bacterial Community in Rhizosphere Soil of Greenhouse Tomatoes
Variations in the frequency and intensity of wet-dry cycles caused different effects on soil microbial communities. Past research has shown that these cycles can lead to a mortality rate of around 58% for microorganisms, but those that survive benefit from the release of active organic matter during rapid changes in water availability [37–39]. In this research, it was discovered that the diversity of bacterial communities in the soil surrounding tomato roots showed an initial increase followed by a decrease as the frequency of wetting cycles intensified. This observed trend is likely due to the formation of small wetting zones and prolonged wet periods, causing excessive soil evaporation in consistently wet conditions. Moreover, the reduced soil aeration linked to high irrigation frequencies can lead to competition between small wetting zones and poorly aerated soil for resources like water, nutrients, and oxygen, significantly impacting the microbial community structure [26,27]. The richness of microbial communities in the root area is constrained by factors like insufficient moisture levels in the tillage layer [40–42]. For instance, when the moisture level was insufficient (F3), the soil volumetric water content for spring and autumn tomatoes was lowered by around 2.16% and 6.02% compared to the irrigation frequency. This decrease in soil moisture serves as a primary constraint on the bacterial community within the rhizosphere soil of tomatoes under MSM [40]. Additionally, When the irrigation frequency is 7 d, the water content of the soil is unevenly distributed in space and time, which promotes the root soil of the crop to be dry or wet for a long time and reduces the number of dry-wet cycles of the soil, the mineralization ability of the soil, and the substrate source required for microbial survival [43–45]. Past studies have demonstrated that water plays a crucial role in influencing the diversity and quantity of soil bacteria communities in times of soil drought. When the porosity of the soil, which is filled with water, is too high, there can be a restriction in gas exchange between the soil and the external environment. This makes it easier for soil-aerobic bacteria to survive and out-compete other organisms. Consequently, the diversity and abundance of soil microbial communities decrease as a result of environmental filtering processes [32,46]. This study observed that the NMGA in the F2 treatment with a more frequent MSM was higher than those in the F1 and F3 treatments. High irrigation frequency F1 may cause the soil to remain wet for a long time, which will increase the water-filled porosity of the soil. In this state, nitrogen-fixing, nitrifying, and denitrifying bacteria (mainly aerobic bacteria) in the soil may face a decline in abundance due to competition for survival [47]. On the other hand, when the irrigation frequency reaches F3, the crop root soil may undergo a long period of drying and wetting alternation, which will cause water and hypoxia stress, thereby reducing the number of roots absorbed by the tillage layer and the number of root exudates [48,49]. This not only reduces the survival substrates of bacteria but also may further reduce the abundance of nitrogen metabolism-related functional bacteria because they are at a disadvantage in niche competition [50,51]. With an increased frequency of MSM, the PMGA in the soil of tomato plants initially increased and then decreased, indicating that the F2 treatment helped accelerate the soil phosphorus cycle [52,53].
Prior research indicated that as irrigation intensity (I) increased, soil volumetric water content also increased, while soil air permeability decreased [40]. The latest research noted a comparable pattern in the diversity of bacterial community composition in the soil surrounding tomato roots at I levels. Initially, the diversity increased but later decreased, possibly due to water stress resulting from insufficient irrigation (I1). This stress could hinder the morphological development of crop roots, decreasing the input of organic matter from root exudates and litter, subsequently limiting the availability of essential carbon for soil bacteria survival. Conversely, excessive irrigation (I3) led to water-filling soil pores, significantly impacting bacterial diversity [54,55]. Reduced soil oxygen levels due to limited air intake under excessive irrigation can stress crop roots and lower soil pH, which affects soil microorganism diversity positively correlated with pH [56]. Competition between crop roots and soil-aerobic bacteria under low oxygen stress can decrease soil-aerobic bacterial species [57,58]. This finding aligns with Li’s [59] conclusion that cucumber rhizosphere microbial community diversity initially increases and then decreases with underground drip irrigation, showing similarities between the effects of managed soil profile fertility (MSM) and drip irrigation on rhizosphere microbial community diversity.
The research determined that the tomato rhizosphere soil treated with irrigation amount I2 had the highest abundance of nitrogen metabolism functional genes. This may be due to the increase of soil volumetric heat capacity with the increase of irrigation amount, which helps to maintain soil temperature and reduce the diurnal variation of soil temperature [60]. Stable soil temperature helps to reduce the fluctuation of soil internal structure, thus providing a good environment for the stable growth of dominant bacteria in soil [61,62]. Nevertheless, excessive soil moisture hindered proper soil aeration. Furthermore, the study was carried out under film irrigation and mulching, restricting soil gas and air exchange. Consequently, the proliferation of aerobic bacteria involved in nitrogen fixation, such as bacterial nitrogen fixation and rhizobia, was constrained. Excessive soil moisture impeded soil ventilation, thereby limiting the reproduction of aerobic bacteria. This limitation, including bacterial nitrogen fixation and rhizobia, led to the lower NMGA found in the tomato rhizosphere soil treated with irrigation intensities of I1 and I3 in this study [58,63]. These results align with Wang et al.’s [64] the conclusion that increasing winter wheat irrigation can enhance NMGA and with Chen et al.’s [65] findings showing a positive correlation between irrigation amount and soil denitrifying bacteria abundance. The collective studies indicate a consistent relationship between irrigation levels and NMGA in crop rhizosphere soil.
4.2 Effects of Different Treatments on Rhizosphere Soil Enzyme Activities of Tomato in Greenhouse
Previous research indicated that the number of dry-wet cycles experienced by the soil in the root zone varied under different irrigation cycles for crops. More frequent dry-wet cycles were found to break up soil aggregates, increase the release of organic carbon, and enhance the available substrate for soil microorganisms [66,67]. Soil treated with F2 of MSM showed higher activity levels of carbon, nitrogen, and phosphorus metabolic enzymes compared to F1 and F3 treatments. This difference in enzyme activities may be due to the soil moisture concentration in the shallow soil layer during more frequent irrigation (F1). This led to an increase in water-filled porosity in the shallow soil, limiting gas exchange between the deep soil layer and external air. The resulting hypoxic stress impeded the morphological growth of tomato roots. Additionally, it was observed that the total root length and activity of tomatoes treated with F2 were greater than those treated with F1. The reduced root exudates and soil enzyme activity associated with F1 were linked to impaired root morphological development [68–70].
Under the reduced irrigation frequency (F3), soil microorganisms struggle to adjust to changing environmental conditions, leading to long-term dry or wet conditions in the soil due to decreased moisture and oxygen levels. This prolonged dry or wet state diminishes the diversity of the soil bacterial community, reduces population abundance, and hinders the activation of soil nutrients, hampering the growth of tomato roots. Spring and autumn tomato plants subjected to F2 treatment exhibited greater total root length and root activity compared to those under F3 conditions. There exists a positive relationship between soil enzyme activity and root exudates [64,71,72]. Li’s research [59] revealed that setting the irrigation frequency at 8 days resulted in a notable presence of soil microorganisms and high soil enzyme activity. These disparities may stem from various factors like irrigation techniques, regional climate, and soil composition. It is crucial to acknowledge that these factors can influence the structure of the soil microbial community [73]. Nevertheless, additional research and analysis are necessary to fully grasp the extent of these relationships and their implications.
As irrigation increased, the activities of soil enzymes (BG, LAD, NAG, and AP) initially rose and then declined in the greenhouse tomato plants under limited water conditions. Soil moisture is important to be a key factor constraining soil enzyme function at lower irrigation levels (I1) [74]. Once irrigation surpassed a certain threshold, soil aeration became inadequate. For example, the soil water saturation level for I3 was approximately 3.55% and 2.25% higher than that for I2, leading to a hypoxic microenvironment in the soil that suppressed the growth of aerobic microorganisms like Bacillus. The reduced diversity and abundance of the soil microbial community led to a decrease in the uptake and utilization of soil nutrients by plant roots, as well as hindered the metabolic processes of the roots. Additionally, the decrease in root metabolic compounds, including root exudates and soil microbial biomass, directly constrained the improvement of soil enzyme activity [40]. This finding is consistent with the research on soil carbon and nitrogen-related enzymes of Tuo [75] and the soil alkaline phosphatase activity of Zhou [76] with the change of irrigation amount.
4.3 Exploring the Effects of Various Treatments on Tomato Production in a Greenhouse
The results indicated that the yield of tomatoes increased at first and then decreased with a higher F of irrigation under the same I. This trend is believed to be attributed to the requirement for stable soil water potential and a greater rate of soil oxygen diffusion to facilitate ideal crop growth [77–79]. Frequent and small amounts of watering in treatment F1 resulted in water being retained in a smaller area of soil, and soil moisture was mainly concentrated in shallow soil (0–10 cm). The average soil moisture content in the top 20 cm of soil in treatment F2 was lower than that in treatment F1. This led to a decrease in the depth at which water could penetrate the soil, causing a reduction in the roots. Additionally, the shallow soil exhibited higher water saturation but poor permeability [80–82]. Under different levels of irrigation intervals (7 days, F3), the distribution of soil moisture was found to be uneven both spatially and temporally. When soil either remained too dry or excessively saturated for extended periods, it led to water and hypoxia stress. Previous studies have shown that soil microorganisms were affected by these conditions of soil moisture and hypoxia stress. In addition, the limited growth of root morphology affected the composition of the soil bacterial community and the presence of functional genes [83]. For example, when the irrigation frequency was set at 5 days, the soil samples from spring and autumn tomato plants indicated a higher abundance of NMGA and PMGA compared to frequencies of 3 and 7 days. This difference in gene abundance led to a decrease in urease and alkaline phosphatase activities in the soil, thereby limiting the turnover of nitrogen and phosphorus and resulting in a negative feedback effect. Additionally, this unfavorable condition impeded the growth of crop roots’ morphology and affected the accumulation of dry matter via leaf photosynthesis. In addition, the harvest of both spring and autumn tomatoes showed higher yields when irrigation was carried out every 5 days. JacobFara et al.’s [84] research demonstrated that tomato yield were higher under film compared to 5-day irrigation, contradicting the findings of the present study. This inconsistency may have been due to variations in the physical and chemical properties of the saline-alkali test soil. In addition to traditional fertilization, it is recommended to incorporate limestone and gypsum before transplanting tomatoes. The study confirmed a positive relationship between irrigation frequency and volume, which aids in leaching soil salts and mitigating salt alkali stress on tomato roots [85]. Furthermore, before transplanting tomatoes per Sorotori’s method, the soil underwent 60 cm subsoiling tillage, resulting in improved soil aeration, water retention, and decreased susceptibility to drought stress impacting tomato growth [86]. It is consistent with the changing trend of tomato yield under drip irrigation with irrigation frequency in Zhang et al. [87], indicating that the influence of the irrigation schedule in MSM on tomato production closely mirrors that of drip irrigation.
The study showed a trend in which tomato yield first rose and then fell as irrigation levels were raised from 0.70 to 1.20 Epan. This outcome was likely due to the insufficient soil volumetric water content in the 0.70 Epan treatment, leading to water stress caused by reduced I. It was crucial to uphold a suitable soil volumetric water content to alleviate soil water stress and enhance crop yield optimization [88,89]. Nonetheless, the application of 1.20 Epan led to a notable increase in soil water saturation, consequently decreasing the aeration within the tomato soil. This combination of water stress and limited oxygen levels had a detrimental effect on the growth of the crop roots. Conversely, the use of 1.00 Epan created an optimal soil moisture environment that encouraged superior root development. Specifically, the combined total root length of tomatoes treated with 1.00 Epan exceeded those subjected to 0.70 and 1.20 Epan treatments. Moreover, the root activity of tomatoes under the 1.00 Epan regimen outperformed those under the 0.70 and 1.20 Epan protocols. The increased root growth positively impacted the composition of the bacterial community. For example, the bacterial ACE index in the rhizosphere soil of tomatoes treated with 1.00 Epan was higher than in those treated with 0.70 and 1.20 Epan. Additionally, the presence of functional genes linked to nitrogen metabolism in tomatoes was more pronounced in the 1.00 Epan treatment compared to the 0.70 and 1.20 Epan approaches. The accelerated growth of root structure and the enhanced metabolic activity of soil bacteria led to an increase in the release of soil enzymes [77,90]. This elevated enzyme activity facilitated the uptake of soil nutrients by crop roots, boosting the advancement of root morphology to a greater extent [91]. The favorable synergy among roots, soil bacteria, and soil enzyme activity contributed to the increased yield of tomatoes treated with 1.00 Epan. It was consistent with Wang et al.’s [92] study that the yield of fragrant pear increased first and then decreased with the increase of drip irrigation amount, which was consistent with Wang et al.’s [93] research, it was observed that the yield of tomatoes initially rose and then declined as the I of drip irrigation increased. This suggests that the impact of adjusting irrigation levels on tomato yield within the MSM was similar to drip irrigation, which further indicated that the effect of I on crop yield did not change due to the difference in crop types and irrigation methods.
4.4 Correlation among Soil Bacterial Community, Soil Enzyme Activity, and Yield
Previous studies have shown that there is a cascade effect in interactions among plants, soil, and microbes. Root exudates are essential in controlling the interplay between plant root enzymes and microbes, ultimately improving the absorption and utilization of nutrients by plants. Furthermore, the presence of root exudates, root dispersion, and soil nutrients significantly impact the movement of bacterial communities [94,95]. Root hairs can adjust to root features to support plant nutrition in dry conditions. Soil moisture is more crucial than root exudates in influencing hydrolase activity during this time. It is essential to recognize that both soil moisture and root exudates collaborate to affect the hydrolase activity of carbon, nitrogen, and phosphorus in the soil surrounding the roots [94]. The diversity of soil bacteria and the activity of hydrolases were constrained by soil moisture, and a synergistic phenomenon of change was present [96]. The optimal water and heat conditions in the soil were conducive to the growth of tomato root structure, which enhanced the resilience of the soil’s bacterial community and increased soil enzyme activity. Additionally, the suitable water and heat conditions in the soil played a crucial role in ensuring a consistent tomato yield [97,98]. The findings indicated a direct relationship between soil bacterial community, soil enzyme activity, and the yield of tomatoes. This may be because soil moisture is an important factor limiting soil bacterial community structure, enzyme activity and yield in this study.
It was discovered that excessive or insufficient soil moisture levels were not beneficial for enhancing tomato root activity, total root length, and root surface area. Inadequate root morphology development can lead to a decrease in root exudate production, which in turn diminishes the interaction between the rhizosphere soil bacterial community, enzyme activity, and roots. This limitation can ultimately hinder the absorption and utilization of soil nutrients, impacting the enhancement of tomato yield. Furthermore, research indicates that drought conditions may elevate soil available phosphorus levels while simultaneously diminishing soil microbial diversity and reducing underground biomass [99]. The study findings revealed a mutually beneficial connection between the composition of the soil’s bacterial community, the activity of soil enzymes, and the growth of tomatoes. This discovery was consistent with Wang’s research [100], highlighting enzymes as indicators of soil function and endorsing the use of functional gene abundance to support model prediction. Similarly, Xing et al.’s conclusion [101] noted a positive connection between potato tuber yield, soil microbial population, and soil enzyme activity in drip irrigation systems. The consistency across these findings indicated that both the MSM technique and drip irrigation influenced the relationship between yield and microbial and enzyme activity in the soil in a similar manner.
In this research, the impact of varying irrigation frequencies and irrigation amounts of MSM on the bacterial community and enzyme activity in the rhizosphere soil of greenhouse tomatoes was examined. The results showed that, in terms of OTU classification, the total number of soil bacterial communities in tomatoes increased initially and then decreased as the levels of F or I increased. Notably, the bacterial community structure diversity and the abundance of functional genes linked to bacterial nitrogen and phosphorus metabolism were highest in the rhizosphere soil of tomatoes when using F2 irrigation frequency. Similarly, soil enzyme activities including BG, LAP, NAG, and AP were notably higher in both spring and autumn tomato soil with F2 compared to F1 and F3. Furthermore, the CHAO, NMGA, and PMGA in the rhizosphere soil of tomatoes were higher with I2 compared to I1 and I3. As F or I increased, there was an initial rise followed by a decrease in tomato yield, emphasizing that I had a more significant impact on tomato yield. A minimum increase of 39.24% in tomato yield was observed for every 1.00% increase in I. The Pearson double-tailed test demonstrated a positive correlation among soil bacterial community, soil enzyme activity, and greenhouse tomato yield under MSM irrigation methods. After a thorough analysis of the experimental data, it was recommended to implement the F2I2 combined irrigation mode under MSM in Northwest China.
Acknowledgement: The authors sincerely thank the editorial teachers and anonymous reviewers who made valuable comments on this paper.
Funding Statement: The research is funded by the Natural Science Foundation of China (No. 41807041), the Science and Technology Research Project of Henan Province (242102111101), and the Mechanical Design, Manufacturing, and Automation Key Discipline of Henan Province (JG [2018] No.119).
Author Contributions: Formal analysis was conducted by Haijian Yang. Data curation was performed by Na Xiao. Yuan Li was responsible for project administration. Mingzhi Zhang conducted writing, review, and editing. All authors reviewed and approved the final version of the manuscript for publication.
Availability of Data and Materials: The datasets used and/or analyzed during the current study are available from the corresponding author upon reasonable request.
Ethics Approval: Not applicable.
Conflicts of Interest: The authors declare that they have no conflicts of interest to report regarding the present study.
References
1. Zhao M, Li C, Zhang C, Han B, Wang X, Zhang J, et al. Typical microplastics in field and facility agriculture dynamically affect available cadmium in different soil types through the physicochemical dynamics of carbon, iron, and microbes. J Hazard Mater. 2022;440:129726. doi:10.1016/j.jhazmat.2022.129726. [Google Scholar] [PubMed] [CrossRef]
2. Zhang M, Xiao N, Li Y, Li Y, Zhang D, Xu Z, et al. Growth and fruit yields of greenhouse tomato under the integrated water and fertilizer by moistube irrigation. Agronomy. 2022;12:1630. doi:10.3390/agronomy12071630. [Google Scholar] [CrossRef]
3. Zhu X, Shong C, Chen G, Zhang W, Li B, Wang G. Advancement in research on bacterial chemotaxis in soil. Acta Pedologica Sinica. 2019;56:259–75 (In Chinese). [Google Scholar]
4. Li G, Ma KM. PICRUSt-based predicted metagenomic analysis of treeline soil bacteria on Mount Dongling, Beijing. Acta Ecologica Sinica. 2018;38:2180–6 (In Chinese). [Google Scholar]
5. Zhu X, Gong W, Li W, Bai X, Zhang C. Reclamation of waste coal gangue activated by Stenotrophomonas maltophilia for mine soil improvement: solubilizing behavior of bacteria on nutrient elements. J Environ Manage. 2022;320:115865. doi:10.1016/j.jenvman.2022.115865. [Google Scholar] [PubMed] [CrossRef]
6. Sura S, Waiser MJ, Tumber V, Raina-Fulton R, Cessna AJ. Effects of a herbicide mixture on primary and bacterial productivity in four prairie wetlands with varying salinities: an enclosure approach. Sci Total Environ. 2015;512–513:526–39. [Google Scholar] [PubMed]
7. Hermans SM, Buckley HL, Case BS, Curran-Cournane F, Taylor M, Lear G. Using soil bacterial communities to predict physico-chemical variables and soil quality. Microbiome. 2020;8(1):79–92. doi:10.1186/s40168-020-00858-1. [Google Scholar] [PubMed] [CrossRef]
8. Zhang J, Zhang B, Liu Y, Guo Y, Shi P, Wei G. Distinct large-scale biogeographic patterns of fungal communities in bulk soil and soybean rhizosphere in China. Sci Total Environ. 2018;644:791–800. doi:10.1016/j.scitotenv.2018.07.016. [Google Scholar] [PubMed] [CrossRef]
9. Huang Y, Huang M, Chai L, Zhao Y. Drivers of the spatial patterns of soil microbial communities in arid and semi-arid regions. Ecol Environ Sci. 2018;27:191–8. [Google Scholar]
10. Shi Y, Li Y, Xiang X, Sun R, Yang T, He D, et al. Spatial scale affects the relative role of stochasticity versus determinism in soil bacterial communities in wheat fields across the North China Plain. Microbiome. 2018;6(1):27–39. doi:10.1186/s40168-018-0409-4. [Google Scholar] [PubMed] [CrossRef]
11. Xu H, Qu Q, Li G, Liu G, Geissen V, Ritsema CJ, et al. Impact of nitrogen addition on plant-soil-enzyme C–N–P stoichiometry and microbial nutrient limitation. Soil Biol Biochem. 2022;170:108714. doi:10.1016/j.soilbio.2022.108714. [Google Scholar] [CrossRef]
12. Acharya P, Ghimire R, Acosta-Martínez V. Cover crop-mediated soil carbon storage and soil health in semi-arid irrigated cropping systems. Agr Ecosyst Environ. 2024;361(2):108813. doi:10.1016/j.agee.2023.108813. [Google Scholar] [CrossRef]
13. Sardans J, Peñuelas J. Drought decreases soil enzyme activity in a Mediterranean Quercus ilex L. forest. Soil Biol Biochem. 2005;37(3):455–61. doi:10.1016/j.soilbio.2004.08.004. [Google Scholar] [CrossRef]
14. Feyissa A, Gurmesa GA, Yang F, Long C, Zhang Q, Cheng X. Soil enzyme activity and stoichiometry in secondary grasslands along a climatic gradient of subtropical China. Sci Total Environ. 2022;825(1):154019. doi:10.1016/j.scitotenv.2022.154019. [Google Scholar] [PubMed] [CrossRef]
15. Zhang L, Zhang QS, Ren KY, Li JJ, Duan YH, Xu MG. Soil ecoenzymatic stoichiometry and relationship with microbial biomass in fluvo-aquic soils with various fertilities. Scientia Agr Sinica. 2020;53:4226–36. [Google Scholar]
16. Lewis WH, Tahon G, Geesink P, Sousa DZ, Ettema TJG. Innovations to culturing the uncultured microbial majority. Nat Rev Microbiol. 2020;19(4):225–40. [Google Scholar] [PubMed]
17. Zhao Q, Xing Y, Sun Y, Lin X, Zhu F. Effects of different soil conditioner application on coffee seedlings growth and soil enzyme activities in acidic continuous cropping soil. Chin J Tropic Crops. 2017;38:1868–73 (In Chinese). [Google Scholar]
18. Boddington CL, Dodd JC. Evidence that differences in phosphate metabolism in mycorrhizas formed by species of Glomus and Gigaspora might be related to their life-cycle strategies. New Phytol. 1999;142(3):531–8. doi:10.1046/j.1469-8137.1999.00422.x. [Google Scholar] [CrossRef]
19. Sun A, Jiao X, Chen Q, Wu A, Zheng Y, Lin Y, et al. Microbial communities in crop phyllosphere and root endosphere are more resistant than soil microbiota to fertilization. Soil Biol Biochem. 2021;153:108113. doi:10.1016/j.soilbio.2020.108113. [Google Scholar] [CrossRef]
20. Qiao Y, Wang T, Huang Q, Guo H, Zhang H, Xu Q, et al. Core species impact plant health by enhancing soil microbial cooperation and network complexity during community coalescence. Soil Biol Biochem. 2024;188:109231. doi:10.1016/j.soilbio.2023.109231. [Google Scholar] [CrossRef]
21. Mutlu A. The influence of different bacteria consortium and irrigation levels on yield and physiological attributes of durum wheat (Triticum durum Desf.) grown under organic farming conditions. J King Saud Univ Sci. 2022;34(8):102366. doi:10.1016/j.jksus.2022.102366. [Google Scholar] [CrossRef]
22. Yang Y, Wang Z, Hu Y, Zeng Z. Irrigation frequency alters the abundance and community structure of ammonia-oxidizing archaea and bacteria in a northern Chinese upland soil. Eur J Soil Biol. 2017;83:34–42. doi:10.1016/j.ejsobi.2017.09.005. [Google Scholar] [CrossRef]
23. Peng Y, Fei L, Liu X, Sun G, Hao K, Cui N, et al. Coupling of regulated deficit irrigation at maturity stage and moderate fertilization to improve soil quality, mango yield and water-fertilizer use efficiency. Sci Hortic-Amsterdam. 2023;307(3):111492. doi:10.1016/j.scienta.2022.111492. [Google Scholar] [CrossRef]
24. Bickel S, Or D. Aqueous habitats and carbon inputs shape the microscale geography and interaction ranges of soil bacteria. Commun Biol. 2023;6(1):626. doi:10.1038/s42003-023-04703-7. [Google Scholar] [PubMed] [CrossRef]
25. Wang J, Du Y, Niu W, Han J, Li Y, Yang P. Drip irrigation mode affects tomato yield by regulating root-soil–microbe interactions. Agr Water Manage. 2022;260(5):107188. doi:10.1016/j.agwat.2021.107188. [Google Scholar] [CrossRef]
26. Wang J, Li Y, Niu W. Effect of alternating drip irrigation on soil gas emissions, microbial community composition, and root-soil interactions. Agr Water Manage. 2021;256(5):107123. doi:10.1016/j.agwat.2021.107123. [Google Scholar] [CrossRef]
27. Zhang M, Xiao N, Yang H, Li Y, Gao F, Li J, et al. The layout measures of micro-sprinkler irrigation under plastic film regulate tomato soil bacterial community and root system. Front Plant Sci. 2023;14:65. doi:10.3389/fpls.2023.1136439. [Google Scholar] [PubMed] [CrossRef]
28. Xie N. Effects of micro-sprinkling irrigation under film on growth and yield of celery in greenhouse. Water Conserv Sci Cold Region Eng. 2019;2:30–2. [Google Scholar]
29. Yin Y, Li K, Hou Y, Qi Q, Wang L, Wei W, et al. Design of simplified water fertilizer integrated machine and its application effect test in cucumber planting in solar greenhouse. Water Saving Irrig. 2021;7–11. [Google Scholar]
30. Zeng Y, Li C, Dai YX, Ha XJ, Tian L, Li C, et al. Effects of different micro-spray irrigation frequency on growth characteristics and quality of mini watermelon in greenhouse. China Cucurbits Veg. 2021;34:73–6 (In Chinese). [Google Scholar]
31. Zhang M, Niu W, Bai Q, Li Y, Wang J, Wang Z, et al. Improvement of quality and yield of greenhouse tomato (Solanum lycopersicum L.) plants by micro-sprinkler irrigation under plastic film. Appl Ecol Env Res. 2020;18:6905–26. doi:10.15666/aeer. [Google Scholar] [CrossRef]
32. Wang J, Niu W, Li Y. Nitrogen and phosphorus absorption and yield of tomato increased by regulating the bacterial community under greenhouse conditions via the alternate drip irrigation method. Agronomy. 2020;10(3):315. doi:10.3390/agronomy10030315. [Google Scholar] [CrossRef]
33. Puissant J, Jones B, Goodall T, Mang D, Blaud A, Gweon HS, et al. The pH optimum of soil exoenzymes adapt to long term changes in soil pH. Soil Biol Biochem. 2019;138(9):107601. doi:10.1016/j.soilbio.2019.107601. [Google Scholar] [CrossRef]
34. Peng Z, Liu W, Tian R, Yang S, Wang J, Huang J, et al. Effects of altitude and aspect on soil extracellular enzyme activities in Tanggula Mountain. Acta Ecologica Sinica. 2021;41:7659–68. [Google Scholar]
35. Wang G, Jin Z, Wang X, George TS, Feng G, Zhang L. Simulated root exudates stimulate the abundance of Saccharimonadales to improve the alkaline phosphatase activity in maize rhizosphere. Appl Soil Ecol. 2022;170:104274. doi:10.1016/j.apsoil.2021.104274. [Google Scholar] [CrossRef]
36. Wang J, Li Y, Niu W. Deficit alternate drip irrigation increased root-soil-plant interaction, tomato yield, and quality. Int J Environ Res Public Health. 2020;17(3):781. doi:10.3390/ijerph17030781. [Google Scholar] [PubMed] [CrossRef]
37. Van Gestel M, Merckx R, Vlassak K. Microbial biomass responses to soil drying and rewetting: the fate of fast-and slow-growing microorganisms in soils from different climates. Soil Biol Biochem. 1993;25:109–23. doi:10.1016/0038-0717(93)90249-B. [Google Scholar] [CrossRef]
38. Guo X, Du S, Guo H, Min W. Long-term saline water drip irrigation alters soil physicochemical properties, bacterial community structure, and nitrogen transformations in cotton. Appl Soil Ecol. 2023;182:104719. doi:10.1016/j.apsoil.2022.104719. [Google Scholar] [CrossRef]
39. Majumdar A, Upadhyay MK, Giri B, Srivastava S, Srivastava AK, Jaiswal MK, et al. Arsenic dynamics and flux assessment under drying-wetting irrigation and enhanced microbial diversity in paddy soils: a four year study in Bengal delta plain. J Hazard Mater. 2021;409:124443. doi:10.1016/j.jhazmat.2020.124443. [Google Scholar] [PubMed] [CrossRef]
40. Wang J. Effect of mulched drip irrigation on crop root-zone soil microenvironment and crop growth in plastic greenhouse. Xi’an: Northwest A&F University; 2017. p. 170. [Google Scholar]
41. Adu JK, Oades JM. Physical factors influencing decomposition of organic materials in soil aggregates. Soil Biol Biochem. 1978;10:109–15. doi:10.1016/0038-0717(78)90080-9. [Google Scholar] [CrossRef]
42. Do Nascimento FAL, Da Silva AJP, Freitas FTO, Fernandes RDM, Veimrober Junior LA. Sensors and frequencies of soil water content measurement affecting agro-hydrological simulations and irrigation management. Comput Electron Agr. 2022;194:106763. doi:10.1016/j.compag.2022.106763. [Google Scholar] [CrossRef]
43. Qu F, Zhang Q, Jiang Z, Zhang C, Zhang Z, Hu X. Optimizing irrigation and fertilization frequency for greenhouse cucumber grown at different air temperatures using a comprehensive evaluation model. Agr Water Manage. 2022;273:107876. doi:10.1016/j.agwat.2022.107876. [Google Scholar] [CrossRef]
44. Luo G, Zhu Q, Jin T, Peng J, Zuo N, Zhang H, et al. Crop types and irrigation regimes as drivers of plastisphere bacterial communities in plastic-mulching croplands of subtropical China. Appl Soil Ecol. 2023;182:104696. doi:10.1016/j.apsoil.2022.104696. [Google Scholar] [CrossRef]
45. Yang X, Jiang N, Sun D. Dry-wet cycles induce the decoupling of carbon and nitrogen mineralization at high temperatures in semi-arid grassland soil. Soil Biol Biochem. 2024;188:109227. doi:10.1016/j.soilbio.2023.109227. [Google Scholar] [CrossRef]
46. Gregorutti VC, Caviglia OP. Impact of crop aerial and root biomass inputs on soil nitrifiers and cellulolytic microorganisms. Soil Tillage Res. 2019;191(4):85–97. doi:10.1016/j.still.2019.03.018. [Google Scholar] [CrossRef]
47. Liu J, Li H, Yuan Z, Feng J, Chen S, Sun G, et al. Effects of microbial fertilizer and irrigation amount on growth, physiology and water use efficiency of tomato in greenhouse. Sci Hortic. 2024;323(2):112553. doi:10.1016/j.scienta.2023.112553. [Google Scholar] [CrossRef]
48. Grandy AS, Daly AB, Bowles TM, Gaudin ACM, Jilling A, Leptin A, et al. The nitrogen gap in soil health concepts and fertility measurements. Soil Biol Biochem. 2022;175(1):108856. doi:10.1016/j.soilbio.2022.108856. [Google Scholar] [CrossRef]
49. Veresoglou SD, Li GC, Chen J, Johnson D. Direction of plant-soil feedback determines plant responses to drought. Global Change Biol. 2022;28(13):3995–7. doi:10.1111/gcb.16204. [Google Scholar] [PubMed] [CrossRef]
50. Wang Y, Zhang F, Wang H, Bi L, Cheng MH, Yan FL, et al. Effects of the frequency and amount of drip irrigation on yield, tuber quality and water use efficiency of potato in sandy soil of Yulin. Chin J Appl Ecol. 2019;30:4159–68 (In Chinese). [Google Scholar]
51. Maheshwari D. Plant growth and health promoting bacteria. Berlin: Springer-Verlag; 2011. vol. 18, p. 45–79. [Google Scholar]
52. Balemi T, Negisho K. Management of soil phosphorus and plant adaptation mechanisms to phosphorus stress for sustainable crop production: a review. J Soil Sci Plant Nut. 2012;12(3):547–61. [Google Scholar]
53. Monokrousos N, Papatheodorou EM, Orfanoudakis M, Jones DG, Scullion J, Stamou GP. The effects of plant type, AMF inoculation and water regime on rhizosphere microbial communities. Eur J Soil Sci. 2020;71(2):265–78. doi:10.1111/ejss.12882. [Google Scholar] [CrossRef]
54. Zhang M, Wang L, Wang H, Xiao N, Liu J, Liu H, et al. Exploration of water saving and high-yield irrigation model for tomato under microsprinkler irrigation with plastic film in a greenhouse based on spatial analysis. J Sensors. 2022;2022(12):1–12. doi:10.1155/2022/3452727. [Google Scholar] [CrossRef]
55. Lv H, Zhou W, Dong J, He S, Chen F, Bi M, et al. Irrigation amount dominates soil mineral nitrogen leaching in plastic shed vegetable production systems. Agric Ecosyst Environ. 2021;317(9):107474. doi:10.1016/j.agee.2021.107474. [Google Scholar] [CrossRef]
56. Liu J, Shu A, Song W, Shi W, Li M, Zhang W, et al. Long-term organic fertilizer substitution increases rice yield by improving soil properties and regulating soil bacteria. Geoderma. 2021;404:115287. doi:10.1016/j.geoderma.2021.115287. [Google Scholar] [CrossRef]
57. Yuan J, Li G, Yan L, Chen G, Zhang S, Teng R. Soil and plant carbon, nitrogen, and phosphorus content and their stoichiometry in spring wheat under different irrigation treatments in the Loess Plateau. Pratacult Sci. 2020;37:1803–12 (In Chinese). [Google Scholar]
58. Jiang J, Tang W, Zhu Q, Ren J. N2O production from soil nitrification and denitrification bacteria at different water-filled pore space. J Agro-Environ Sci. 2006;25(6):1535–40 (In Chinese). [Google Scholar]
59. Li H, He H, Li T. Microbial activity and functional diversity in rhizosphere of cucumber under different subsurface drip irrigation scheduling. Chin J Appl Ecol. 2014;25:2349–54 (In Chinese). [Google Scholar]
60. Guo Z, Liu C, Hua K, Wang D, Wu P, Wan S, et al. Changing soil available substrate primarily caused by fertilization management contributed more to soil respiration temperature sensitivity than microbial community thermal adaptation. Sci Total Environ. 2024;912(1):169059. doi:10.1016/j.scitotenv.2023.169059. [Google Scholar] [PubMed] [CrossRef]
61. Han Y, Qiao DM, Qi X, Li P, Guo W, Cui B, et al. Effects of reclaimed water irrigation levels on soil salinity and composition of soil bacteria community. Trans Chin Soc Agric Eng. 2020;36:106–17. [Google Scholar]
62. Meisner A, Snoek BL, Nesme J, Dent E, Jacquiod S, Classen AT, et al. Soil microbial legacies differ following drying-rewetting and freezing-thawing cycles. ISME J. 2021;15(4):1207–21. doi:10.1038/s41396-020-00844-3. [Google Scholar] [PubMed] [CrossRef]
63. González EM, Larrainzar E, Marino D, Wienkoop S, Gil-Quintana E, Arrese-Igor C. Physiological responses of N2-fixing legumes to water limitation. Cham: Springer International Publishing; 2015. p. 5–33. [Google Scholar]
64. Wang C, Ma H, Feng Z, Yan Z, Song B, Wang J, et al. Integrated organic and inorganic fertilization and reduced irrigation altered prokaryotic microbial community and diversity in different compartments of wheat root zone contributing to improved nitrogen uptake and wheat yield. Sci Total Environ. 2022;842(2):156952. doi:10.1016/j.scitotenv.2022.156952. [Google Scholar] [PubMed] [CrossRef]
65. Chen H, Shang Z, Cai H, Zhu Y. Response of soil N2O emissions to soil microbe and enzyme activities with aeration at two irrigation levels in greenhouse tomato (Lycopersicon esculentum Mill.) fields. Atmosphere. 2019;10(2):72. doi:10.3390/atmos10020072. [Google Scholar] [CrossRef]
66. Devêvre OC, Horwáth WR. Decomposition of rice straw and microbial carbon use efficiency under different soil temperatures and moistures. Soil Biol Biochem. 2000;32(11–12):1773–85. doi:10.1016/S0038-0717(00)00096-1. [Google Scholar] [CrossRef]
67. Ridgeway JR, Morrissey EM, Brzostek ER. Plant litter traits control microbial decomposition and drive soil carbon stabilization. Soil Biol Biochem. 2022;175(1):108857. doi:10.1016/j.soilbio.2022.108857. [Google Scholar] [CrossRef]
68. Zhang X, Bilyera N, Fan L, Duddek P, Ahmed MA, Carminati A, et al. The spatial distribution of rhizosphere microbial activities under drought: water availability is more important than root-hair-controlled exudation. New Phytol. 2022;237(3):780–92. [Google Scholar] [PubMed]
69. Jiang O, Li L, Duan G, Gustave W, Zhai W, Zou L, et al. Root exudates increased arsenic mobility and altered microbial community in paddy soils. J Environ Sci. 2023;127(1):410–20. doi:10.1016/j.jes.2022.05.036. [Google Scholar] [PubMed] [CrossRef]
70. Zhang X, Dippold MA, Kuzyakov Y, Razavi BS. Spatial pattern of enzyme activities depends on root exudate composition. Soil Biol Biochem. 2019;133:83–93. doi:10.1016/j.soilbio.2019.02.010. [Google Scholar] [CrossRef]
71. Feng X, Pu J, Liu H, Wang D, Liu Y, Qiao S, et al. Effect of fertigation frequency on soil nitrogen distribution and tomato yield under alternate partial root-zone drip irrigation. J Integr Agr. 2022;22(3):897–907. [Google Scholar]
72. Sałata A, Lombardo S, Pandino G, Mauromicale G, Buczkowska H, Nurzyńska-Wierdak R. Biomass yield and polyphenol compounds profile in globe artichoke as affected by irrigation frequency and drying temperature. Ind Crop Prod. 2022;176(4):114375. doi:10.1016/j.indcrop.2021.114375. [Google Scholar] [CrossRef]
73. Obayomi O, Seyoum MM, Ghazaryan L, Tebbe CC, Murase J, Bernstein N, et al. Soil texture and properties rather than irrigation water type shape the diversity and composition of soil microbial communities. Appl Soil Ecol. 2021;161:103834. doi:10.1016/j.apsoil.2020.103834. [Google Scholar] [CrossRef]
74. Baldrian P, Merhautová V, Petránková M, Cajthaml T, Šnajdr J. Distribution of microbial biomass and activity of extracellular enzymes in a hardwood forest soil reflect soil moisture content. Appl Soil Ecol. 2010;46(2):177–82. doi:10.1016/j.apsoil.2010.08.013. [Google Scholar] [CrossRef]
75. Tuo Y, Tan H, Liang J, Li J, Xiang P, Yang Q, et al. Optimization of water and fertilizer management of Panax pseudoginseng based on changes in soil microbial biomass carbon and nitrogen and enzyme activities. Appl Soil Ecol: Sec Agr Ecosyst Environ. 2024;196(54):105282. doi:10.1016/j.apsoil.2024.105282. [Google Scholar] [CrossRef]
76. Zhou S, Zhang M, Zhang K, Yang X, He D, Yin J, et al. Effects of reduced nitrogen and suitable soil moisture on wheat (Triticum aestivum L.) rhizosphere soil microbiological, biochemical properties and yield in the Huanghuai Plain. China J Integr Agr. 2020;19(1):234–50. doi:10.1016/S2095-3119(19)62697-3. [Google Scholar] [CrossRef]
77. Uçan K, Kıllı F, Gençoğlan C, Merdun H. Effect of irrigation frequency and amount on water use efficiency and yield of sesame (Sesamum indicum L.) under field conditions. Field Crop Res. 2007;101(3):249–58. doi:10.1016/j.fcr.2006.11.011. [Google Scholar] [CrossRef]
78. Holzapfel E, Jara J, Coronata AM. Number of drip laterals and irrigation frequency on yield and exportable fruit size of highbush blueberry grown in a sandy soil. Agr Water Manage. 2015;148(2):207–12. doi:10.1016/j.agwat.2014.10.001. [Google Scholar] [CrossRef]
79. Cui J, Cheng Q, Chen P, Guo S, Wang L, Zheng Z, et al. Effects of drip irrigation frequency on soil physical and chemical characteristics and cotton yield under subsoiling condition. J Soil Water Conserv. 2019;33:263–9. [Google Scholar]
80. Buwalda F, Kim KS. Effects of irrigation frequency on root formation and shoot growth of spray chrysanthemum cuttings in small jute plugs. Sci Hortic. 1994;60(1–2):125–38. doi:10.1016/0304-4238(94)90067-1. [Google Scholar] [CrossRef]
81. Chachar AN, Mirjat MU, Soothar RK, Shaikh IA, Mirjat MH, Dahri SA. Effects of irrigation frequencies on soil salinity and crop water productivity of fodder maize. Acta Ecologica Sinica. 2019;176:91–9. [Google Scholar]
82. Wang F, Kang Y, Liu S. Effects of drip irrigation frequency on soil wetting pattern and potato growth in North China Plain. Agr Water Manage. 2006;79(3):248–64. doi:10.1016/j.agwat.2005.02.016. [Google Scholar] [CrossRef]
83. Li Y, Niu W, Zhang M, Wang J, Zhang Z. Artificial soil aeration increases soil bacterial diversity and tomato root performance under greenhouse conditions. Land Degrad Dev. 2020;31(12):1443–61. doi:10.1002/ldr.3560. [Google Scholar] [CrossRef]
84. JacobFara S, Delazari F, Gomes R, Luiz Araújo W, Silvaa DJH. Stomata opening and productiveness response of fresh market tomato under different irrigation intervals. Sci Hortic. 2019;25:86–95. [Google Scholar]
85. Li X, Zhang C, Huo Z, Adeloye AJ. A sustainable irrigation water management framework coupling water-salt processes simulation and uncertain optimization in an arid area. Agr Water Manage. 2020;231(3):105994. doi:10.1016/j.agwat.2019.105994. [Google Scholar] [CrossRef]
86. Kuang N, Tan D, Li H, Gou Q, Li Q, Han H. Effects of subsoiling before winter wheat on water consumption characteristics and yield of summer maize on the North China Plain. Agr Water Manage. 2020;227:105786. doi:10.1016/j.agwat.2019.105786. [Google Scholar] [CrossRef]
87. Zhang J, Xiang L, Liu Y, Jing D, Zhang L, Liu Y, et al. Optimizing irrigation schedules of greenhouse tomato based on a comprehensive evaluation model. Agr Water Manage. 2024;295:108741. [Google Scholar]
88. Liu H, Duan A, Li F, Sun J, Wang Y, Sun C. Drip irrigation scheduling for tomato grown in solar greenhouse based on pan evaporation in North China Plain. J Integr Agr. 2013;12(3):520–31. doi:10.1016/S2095-3119(13)60253-1. [Google Scholar] [CrossRef]
89. Patanè C, Tringali S, Sortino O. Effects of deficit irrigation on biomass, yield, water productivity and fruit quality of processing tomato under semi-arid Mediterranean climate conditions. Sci Hortic. 2011;129(4):590–6. doi:10.1016/j.scienta.2011.04.030. [Google Scholar] [CrossRef]
90. Chen H, Hou H, Wang X, Zhu Y, Saddique Q, Wang Y, et al. The effects of aeration and irrigation regimes on soil CO2 and N2O emissions in a greenhouse tomato production system. J Integr Agr. 2018;17(2):449–60. doi:10.1016/S2095-3119(17)61761-1. [Google Scholar] [CrossRef]
91. Shang ZH, CaI HJ, Chen H, Sun YN, Li L, Zhu Y, et al. Effect of water-fertilizer-gas coupling on soil N2O emission and yield in greenhouse tomatoes. Huanjing Kexue. 2020;41(6):2924–35 (In Chinese). [Google Scholar] [PubMed]
92. Wang J, He X, Gong P, Heng T, Zhao D, Wang C, et al. Response of fragrant pear quality and water productivity to lateral depth and irrigation amount. Agr Water Manage. 2024;292(1–2):108652. doi:10.1016/j.agwat.2023.108652. [Google Scholar] [CrossRef]
93. Wang H, Qu Y, Wen Z, Cheng M, Zhang F, Fan J, et al. Interactive effects of irrigation and N fertilization management on fruit yield, quality and water-N productivity of greenhouse cherry tomato. Sci Hortic. 2024;328:112895. doi:10.1016/j.scienta.2024.112895. [Google Scholar] [CrossRef]
94. Jing J, Cong W, Bezemer TM. Legacies at work: plant-soil–microbiome interactions underpinning agricultural sustainability. Trends Plant Sci. 2022;27(8):781–92. doi:10.1016/j.tplants.2022.05.007. [Google Scholar] [PubMed] [CrossRef]
95. Ghatak A, Chaturvedi P, Waldherr S, Subbarao GV, Weckwerth W. PANOMICS at the interface of root–soil microbiome and BNI. Trends Plant Sci. 2022;28(1):106–22. [Google Scholar] [PubMed]
96. Lane JM, Delavaux CS, van Koppen L, Lu P, Cade-Menun BJ, Tremblay J, et al. Soil sample storage conditions impact extracellular enzyme activity and bacterial amplicon diversity metrics in a semi-arid ecosystem. Soil Biol Biochem. 2022;175:108858. doi:10.1016/j.soilbio.2022.108858. [Google Scholar] [CrossRef]
97. Ulbrich TC, Rivas-Ubach A, Tiemann LK, Friesen ML, Evans SE. Plant root exudates and rhizosphere bacterial communities shift with neighbor context. Soil Biol Biochem. 2022;172(1):108753. doi:10.1016/j.soilbio.2022.108753. [Google Scholar] [CrossRef]
98. Zhang M, Niu W, Xu J, Li Y. Influences of micro-irrigation and subsoiling before planting on enzyme activity in soil rhizosphere and summer maize yield. Chin J Appl Ecol. 2016;27:1925–34 (In Chinese). [Google Scholar]
99. Sokol NW, Foley MM, Blazewicz SJ, Bhattacharyya A, DiDonato N, Estera-Molina K, et al. The path from root input to mineral-associated soil carbon is dictated by habitat-specific microbial traits and soil moisture. Soil Biol Biochem. 2024;193:109367. [Google Scholar]
100. Wang G, Gao Q, Yang Y, Hobbie SE, Reich PB, Zhou J. Soil enzymes as indicators of soil function: a step toward greater realism in microbial ecological modeling. Global Change Biol. 2022;28:1935–50. doi:10.1111/gcb.v28.5. [Google Scholar] [CrossRef]
101. Xing Y, Zhang T, Jiang W, Li P, Shi P, Xu G, et al. Effects of irrigation and fertilization on different potato varieties growth, yield and resources use efficiency in the Northwest China. Agr Water Manage. 2022;261:107351. doi:10.1016/j.agwat.2021.107351. [Google Scholar] [CrossRef]
Cite This Article
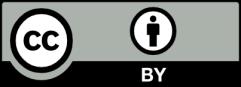
This work is licensed under a Creative Commons Attribution 4.0 International License , which permits unrestricted use, distribution, and reproduction in any medium, provided the original work is properly cited.