Open Access
ARTICLE
Chemically Mediated Interactions between Grapevine, Aphid, Ladybird, and Ant in the Context of Insect Chemical Ecology
1 Department of Chemistry, College of Science, Taif University, P.O. Box 11099, Taif, 21944, Saudi Arabia
2 Department of Biology, College of Science, Taif University, P.O. Box 11099, Taif, 21944, Saudi Arabia
3 Chemistry Department, Faculty of Science, King AbdulAziz University, P.O. Box 80200, Jeddah, 21589, Saudi Arabia
* Corresponding Author: Taghreed Alsufyani. Email:
Phyton-International Journal of Experimental Botany 2024, 93(7), 1523-1542. https://doi.org/10.32604/phyton.2024.050351
Received 04 February 2024; Accepted 24 May 2024; Issue published 30 July 2024
Abstract
This study simplifies the complex relationship among grapevine plants, aphids, ladybirds, and ants, which is essential for effective pest management and ecological balance. This study investigated the impact of aphid attacks and the presence of ants and ladybirds on the volatile compounds profile released into the chemosphere of the community consisting of the common vine Vitis vinifera, the aphid Aphis illinoisensis, the ladybird Coccinella undecimpunctata-and the ant Tapinoma magnum. This study aims to analyze the volatile compounds emitted by the grapevine and surrounding insects in response to these intricate interactions. The extraction of volatile organic compounds (VOCs) was carried out using closed-loop stripping (CLS) and then analyzed via gas chromatography-mass spectrometry (GC-MS) and principles coordinated analysis (PCA) was performed. The grapevine was exposed to different types and order of treatments, including non-infested, aphid-infested, aphid-infested with ant, aphid-infested with ladybird, and various combinations of ant and ladybird. After the aphid attack, the outcomes uncovered massive alterations in the volatile compound profiles. Infested grapevine displayed distinct emissions of germacrene D, an alcohol, and an alkene compared to non-infested plants. The characteristic VOC profile was the share of infested grapes in the presence of ants, with benzene derivatives and sesquiterpenes dominating the components. The coexistence of ladybirds with ants and aphids resulted in a different volatile profile characterized by elevated levels of aldehydes, ketones, α-farnesene, and its hydroxy derivative. It was concluded that the emission of VOCs into the chemosphere of the grapevine communities varied qualitatively and quantitatively depending on the level of the relationship complexity within each community in response to the infestation of grapevines by aphids, the presence of ladybirds as natural predators, and the presence of ant as protector. The grapevine’s status-dependent compounds can serve as indicators of infestation status and contribute to non-destructive early-stage diagnosis of the aphid.Keywords
Nomenclature
V. vinifera | Vitis vinifera |
A. illinoisensis | Aphis illinoisensis |
C. undecimpunctata | Coccinella undecimpunctata |
T. magnum | Tapinoma magnum |
VOCs | Volatile organic compounds |
CLS | Closed-loop stripping |
GC–MS | Gas chromatography-mass spectrometry |
RT | Retention time |
G1, G2, G3, G4, G5, G6 | Groups 1, 2, 3, 4 5, and 6, respectively |
PCA | Principal component analysis |
TPC1 | The tripartite community consists of grapevine leaves + A. illinoisensis + T. magnum |
TPC2 | The tripartite community consists of grapevine leaves + A. illinoisensis + C. undecimpunctata |
QPC1 | The quadripartite community consists of grapevine leaves + A. illinoisensis + T. magnum + C. undecimpunctata |
QPC2 | The quadripartite community consists of grapevine leaves + A. illinoisensis + C. undecimpunctata + T. magnum |
°C | Degree Celsius |
h | Hour |
µL | Microliter |
mL | Milliliter |
min | Minute |
m/z | M stands for mass and Z stands for charge number of ions |
amu | Atomic mass unit |
eV | Electron volt |
|r| | Absolute value describes the distance from zero that a number is on the number line, without considering direction |
The value of grapevine (Vitis vinifera) at the farm gate is around 80 billion dollars worldwide, making it a valuable fruit crop [1]. V. vinifera is the second most important economic fruit in Saudi Arabia after the date. The Taif Governate produces 3000 tons of V. vinifera, a special grape characterized worldwide by its taste and shape [2]. It is one of the most common grape species in the Vitaceae family and belongs to the genus Vitis. Phenolic compounds, aromatic compounds, flavonoids, proanthocyanins, and stilbenoids are among the significant chemicals found in grapevine [3,4]. Grapevines are at the center of complex communities and relationships in trophic networks, with a wide number of organisms residing both above and below the ground. Some of these organisms, such as rhizobacteria, mycorrhizal fungi, or entomophagous arthropods, are beneficial, while others, such as microbial pathogens or phytophagous arthropods, are detrimental to grape production [5]. The production of grapes is restricted by a variety of biotic and abiotic factors, including pathogens, arthropod pests, increasing global temperature, and drought [6,7]. Pests have the power to influence plant growth and development by feeding directly, spreading viruses or bacteria indirectly, or changing plant biochemistry [8]. Hemipteran insects, which have piercing or sucking mouthparts, bypass the direct breakdown of plant material and instead extract plant materials by ingesting liquids, either through external digestion of cell contents or feeding on phloem or xylem [9]. Plant growth or development may be significantly impacted by these insects, either through the removal of resources or the introduction of viruses. The use of salicylic acid has been suggested for insects that feed by sucking or piercing to induce direct plant defenses [10]. There are many indirect defenses that can be initiated, such as plant hormone changes, beneficial insect attraction, and volatile chemical release [11]. Plant hormones can be released to alter plant growth, metabolism, and photosynthetic rate when sucking insects are present [12–14].
Beneficial insects’ attraction decreases the effect of sucking insects, as they eat or parasitize parasitize insects feeding on plants [15]. In horticulture, aphids are considered among the most destructive pests [16]. The aphid-ant relationship is a typical example of symbiosis due to their common association with ants for protection [17]. Aphids are the target of attacks from various predators, including ladybirds and lacewing larvae [18,19]. Ladybirds are regarded as one of the most prominent hunters of aphids and coccids worldwide. This greatly underestimates the diversity of their biology, and maximizing the impact on their prey is also essential for modern conservation. Additionally, indigenous natural enemies are being utilized for biological control instead of the classical method of introducing foreign species [20].
Ladybirds are among the most beneficial insects in the vineyard [21]. Both young and adult larvae are active feeders; young larvae try to penetrate their prey and suck out all the nutrients and components that keep them alive [22]. In contrast, larvae or adult ladybirds help engulf moth eggs, some pathogens, and small insects; they also survive on pollen and nectar in and near vineyards [20].
In response to insect infestation, there has been limited research on VOCs in grapevines. However, one study did demonstrate changes in grapevines inflicted with phylloxera (Daktulosphaira vitis Fitch) [23]. Infected grapevines have higher levels of certain compounds, including methyl salicylate and benzaldehyde. In plants attacked by Hemiptera, as well as under stressful abiotic conditions, the levels of numerous salicylic pathway compounds are known to increase. Insinuating that these plants usually respond defensively to sucking insects’ attacks [24]. The study by Alotaibi et al. [25] defined aphid species chemically upon mechanical stress, and A. illinoisensis was distinguished by a group of VOCs, specifically esters, benzenoids, and monoterpenes.
Nonetheless, key physiological, biochemical, and subatomic viewpoints of extraordinary edge development exist; how grapevines respond to environmental pressure and deal with biotic and abiotic stresses is ambiguous [26].
The volatile profile of a system can indeed undergo significant alterations due to various biotic factors, such as the introduction or removal of organisms, including symbionts, as well as abiotic changes encompassing temperature, pH, humidity, light exposure, geographical location, and seasonal variations, among others [27].
The purpose of this study is to enhance our knowledge of the chemical ecology of the pest (aphids) and their interactions with other organisms and the environment such as the host plant (grapevine), the natural enemy (ladybird), and pest protector (ant). Nonetheless, it also offers valuable insights that can be used to develop effective pest management strategies. Specifically, by discerning how volatile profiles respond to such alterations, we can identify candidate compounds for use in pest trapping and repellent applications. This comprehensive examination aimed to inform the development of eco-friendly pest management strategies, including utilizing chemical signals released by the grapevine, and the aggregation pheromone of the aphid for pest trapping, employing the defence compounds released by the grapevine in the presence of the aphid for pest repellence, and harnessing alarm pheromones released by aphid in the presence of the natural enemy ladybird as a means of pest repellent. This study explored the potential heightened impact of chemical signals released by ladybirds in the presence of both the aphid and its protector compared to those released in the presence of the aphid alone without a protector for pest repellence purposes. Finally, the potential use of aggregation pheromones produced by prey (aphids) as a means to attract natural enemies for pest control was investigated [28,29].
Individuals of the species Aphis illinoisensis, Tapinoum magnum, and Coccinella undecimpunctata with accession numbers MZ091377, ON149799, and ON149796, respectively (National Center for Biotechnology Information: NCBI repository), were collected from Taif city and used in this study [30].
For molecular identification of the collected insects, DNA extraction was accomplished using the QIAamp® DNA Mini Kit (QIAGEN, Hilden, Germany) as described elsewhere. The mitochondrial cytochrome oxidase gene (COI) was amplified with PCR, using the primer set (LCO1490) (F-50-GGTCAACAAATCATAAAGATATTGG-30) and (R HCO2) (R-50-TAAACTTCAGGGTGACCAAAAAATCA-30). The PCR reaction was performed as described in our previous study [30]. The assembled sequences were deposited in GenBank with the following accession numbers (A. illinoisensis: MZ091377, C. undecimpunctata: ON149796, and T. magnum: ON149799).
Six groups (n = 24) were set up in 2 L glass desiccators-style chambers (Fig. 1) to minimize VOC loss according to the following criteria:
Figure 1: Experimental setup using closed-loop stripping (CLS) to collect VOCs from the chemosphere of six treatments (G1–G6). The experiment workflow started with intact grape leaves (G1) and ended with quadripartite communities (G5: QPC1, G6: QPC2) passing through G2: aphid-infested grape leaves, G3: TPC1, and G4: TPC2
Group 1 (G1) included a noninfested grapevine (Vitis vinifera), in which an intact branch 20 cm tall was placed. Following G1 were 50–60 A. illinoisensis aphids added to form Group 2 (G2), which included infested grapevine plants. 20 T. magnum ants (G3), or 10 C. undecimpunctata eleven-spot ladybird females (G4) were added to G2 to collect VOCs after 24 h of exposure. Thus, G3 and G4 introduced tripartite communities (TPC1 and TPC2, respectively). G5 (QPC1), and G6 (QPC2) introduced quadripartite communities after adding 10 ladybirds C. undecimpunctata females to G3 and 20 ants T. magnum to G4, respectively. Each group was subjected to three independent biological replicates, and G1, and G2 were initiated with six replicates.
2.4.1 Airborne Metabolite Collection
The different groups were subjected to headspace extraction using closed-loop stripping (CLS) (Fig. 1) [31,32]. Adsorbent-activated carbon traps were cleaned beforehand by rinsing with methanol and then ethyl acetate and drying at 80°C for 2 h. Airborne metabolites were collected during the continuous circulation of headspace air into the interior of the closed chambers. The enclosed volume of air containing the VOCs emitted by the different treatment groups was circulated with vacuum pumps (model no. DC6/18 F; Fürgut GmbH, Germany). The plants in each group were monitored for 24 h. After 24 h, the activated carbon traps were immediately eluted with 60 µL (3 × 20 µL) of ethyl acetate.
2.4.2 Gas Chromatography-Mass Spectrometry (GC–MS) Analysis Conditions
Samples were analyzed with a GC (580 Clarus) coupled to MS (560S Clarus) (Agilent Technologies, Santa Clara, USA). One microliter of each sample was injected into the GC port in split mode. The GC had a nonpolar Elite-5ms capillary column (30 m × 0.25 mm ID, 0.25 μm film thickness; Agilent Technologies). The GC parameters for the analysis were as follows: The carrier gas was helium at a constant flow rate of 1 mL/min. The injection predwell time was set at 0.1 min (hot needle injection). The injector temperature was 220°C. One microliter was injected into the split mode at a ratio of 1:5. The temperature program of the oven was set at 40°C for 3 min. Then, the temperature was increased from 2.8°C/min to 130°C, followed by an increase of 2.4°C/min to 180°C, where it was ramped up to the final temperature of 250°C for 6°C/min. The total GC run time was 67 min. The MS parameters were set as follows: electron energy = 70 eV, trap current emission = 100 µA, source temperature = 150°C, and transfer line temperature = 280°C. The mass spectrometric detector was operated in scan mode (m/z 40–450 amu). The components were identified by comparing their mass spectra with those of Wiley09 and the National Institute of Standard Technology (NIST 14) mass spectral database. The strategy of identifying compounds based on reverse matching for untargeted analysis was adopted by Alsufyani et al. [33]. All the chromatograms were checked manually, and the area of each peak was normalized by the sum of all the peak areas in the sample’s chromatogram. The means of replicates were used for further statistical analysis. Airborne metabolites from different samples were collected and subjected to advanced ecological statistical analysis. The unconstrained discriminant principal component analysis (PCA) was applied to the raw data.
2.4.3 Principal Component Analysis (PCA)
PCA was carried out using XLSTAT 2023 (XLstst.exe, version 25.1.1407; Lumivero, Denver, Colorado) [34]. Before processing the data by PCA, preprocessing was performed, which consisted of calculating the average data for each group of samples and the absence of any compound considered to be zero.
The resulting first two principal axes, F1 and F2, as well as sample coordinates, were then imported into SigmaPlot (version 11.0, Systat Software, USA) for graphical illustration. The biomarkers were screened for significant correlation coefficients, with PCA axes Compounds having a correlation coefficient (|r| ≥ 0.1) with one of the two PCA axes retained as significantly characteristic compounds.
Comprehending the plant’s chemical environment before and after it has been infested with agricultural pests, taking into account the presence or absence of natural enemies or protectors will aid in pest prevention, control, and resistance. In this study, we extracted VOCs emitted in the chemosphere, where 33 airborne metabolites stimulate the beneficial and detrimental interactions between grapevine and A. illinoisensis as key entities and the rest of the surrounding organisms either a pest-protector T. magnum (TPC1), or a pest-natural enemy C. undecimpunctata (TPC2), or all of them (QPC1, 2) (Table 1, Fig. 2A), without exposure to plant, or insect tissue using CLS. The activated charcoal traps used in CLS allowed us to cover a broad range of airborne metabolites that differed across the six treatments of grape V. vinifera. According to the specifications of our study, we are confident that the chemosphere compounds were released either from V. vinifera or A. illinoisensis, T. magnum, or C. undecimpunctata or were released due to interactions between those organisms.
Figure 2: (A) The heatmap of the average percentage of VOCs released into the chemosphere of the six grapevine treatments (G1–G6) according to the GC–MS analysis. The percentages ranged from 0% to 16.3%. Smaller values are shown as red cells and higher values are shown as blue cells in the horizontal direction. Metabolite identification was accepted if the quality varied between 50% and 99%. (B) Column chart represents the emissions percentage of VOCs released in the chemosphere of six grapevine treatments. (C) Column chart shows the distribution of sesquiterpenes in the chemosphere of six grapevine treatments. G1 (light green): intact grapevine, G2 (orange): A. illinoisensis-grapevine, G3 (red): A. illinoisensis-grapevine + T. mangum (TPC1), G4 (light blue): A. illinoisensis-grapevine + C. undecimpunctata (TPC2), G5 (yellow with black frame): A. illinoisensis-grapevine + T. mangum + C. undecimpunctata, respectively (QPC1), G6 (yellow without frame): A. illinoisensis-grapevine + C. undecimpunctata + T. mangum, respectively (QPC2)
3.1 Overview of the Compounds Detected in the Chemosphere
Thirty-three compounds were detected in the chemosphere of the different V. vinifera grapevine treatments extracted by CLS, as shown in Fig. 1.
For non-target analysis, these compounds were tentatively identified (most of which were alkanes, aldehydes, esters, benzene derivatives, and sesquiterpenes) (Fig. 2B). The chemosphere constituents of the noninfested grapes were benzene derivatives (2.28%) and esters (97.7%). After infestation by A. illinoisensis, the chemosphere of the aphid-infested grape plants released alkanes (25.1%), terpenes (26.6%), esters (42.1%), and alcohol (6.1%), but no benzene derivatives or aldehydes were detected. After the ants were added to the infested grape plants, the chemosphere profile of the tripartite community (TPC1) consisted of terpenes (41%), benzene derivatives (50.8%), and esters (8.1%), but no alcohol or aldehydes were detected. The tripartite community of the infested grape and ladybird (TPC2) profile consisted of alkanes (20.3%), terpenes (40.8%), aldehydes (9.6%), and esters (29.1%), but no alcohol or benzene was detected. The quadripartite communities (QPC1,2) were carried out in different orders. When ladybirds were added to TPC1, the chemosphere profile of QPC1 consisted of alkanes (6.5%), terpenes (63.7%), aldehydes (13.5%), and esters (16.2%), but no alcohol or benzene was detected. Additionally, when the ant was added to TPC2, the chemosphere profile of QPC2 contained the same compounds but in different percentages: alkanes (3.01%), terpenes (43.7%), aldehydes (50.5%), and esters (2.6%) (Fig. 2B). Overall, sesquiterpenes were identified only after infestation as seen in Fig. 2C. Thus, this specific sesquiterpene blend, with a retention time of 33.69 to 45.28 min (Table 1), can be used as an indicator for the infestation status of grapevine by A. illinoisensis. Germacrene D (bio #29) was detected 24 h after infestation by A. illinoisensis (G2), and disappeared after that (i.e., G3-G6), unlike caryophyllene (bio #25) which remained in all infested groups (G2-G6). The alarm pheromone β-farnesene (bio #27) was identified in G2–G4 but vanished in QPCs (G5, G6). The highest percentage recorded was the share of the isomer α-farnesene (bio #30) in the QPC1 (G5), followed by QPC2 (G6), and TPC2 (G4), respectively, while the presence of farnesene derivativ7-hydroxy farnesenene (bio #33) was limited to the QPCs (G5, G6). The presence of T. magnum in the infested grapevine (TPC1-G3) was indicated with three sesquiterpenes, namely α-cubebene (bio #26), copaene (bio #28), and cadinene (bio #31). To simplify the complicity of the profile emissions of VOCs, the multivariate principal coordinates analysis (PCA) was implemented on the raw GC-MS dataset. PCA found biomarkers in the chemosphere that are distinctive for grapevine treatments, depending on whether compounds are present, absent, or abundant in chemosphere profiles.
3.2 Biomarkers Extraction for Group Separation
The statistical analysis of PCA (Fig. 3A) revealed that there are significant differences between VOCs in the chemosphere as a result of quantitative or qualitative estimation (Figs. 2A, 3B).
Figure 3: The principal component analysis (PCA) of the chemosphere compounds (n = 33) released by the noninfested grapevine (G1), infested grapevine (G2), tetrapartite communities (G3, G4), and quadripartite communities (G5, G6) analyzed by GC/MS. (A) The first two axes, F1 and F2, which represent 30.21% and 21.09%, respectively, of the variables, are plotted. PCA demonstrated the separation of the samples based on the organisms present in the groups, which affected the presence and absence of chemosphere compounds. (B) Scaled vectors of the chemosphere compounds (ID numbers) were significant (|r| > 0.2) for the separation of the groups. Quadrant communities G5, and G6 were regarded as one group with the same biomarkers by both axes F1 and F2. The numbers refer to the biomarkers in Table 1 and Fig. 1
As shown in Fig. 3A, F1 and F2 (with eigenvalues of 9.94% and 6.96%, respectively) represented 30.12% and 21.09%, respectively, of the variabilities. These findings suggest that F1 and F2 are two variables that contain useful information for distinguishing different groups within the dataset. Such sample distribution provides insights into the relationships and differences between the groups based on the patterns or characteristics captured by these variables (Fig. 3B). Taking the distribution of samples by PCA into account (Fig. 3A), it is clear that the separation via the F1 axis is fully dependent on the infestation status of the grapevine. Thus, the infestation status introduced by the infested grapevine with the aphid-tending ant (G3: TPC1) was separated from the noninfested (G1), as well as the biotreated grapevine by C. undecimpunctata (G4–G6: TPC1, TPC2, QPC1, QPC2). Notably, G1 and G2 stood apart from the groups containing ant (G3: TPC1, G5: QPC1, and G6: QPC2), along the F2 axis. The separation of these groups via F2, particularly concerning the presence or absence of T. mangum. The separation of five groups via F1, and F2 (Fig. 3) emphasizes the intricate chemical coordination governing the interactions among grapevine plants, aphids, ants, and ladybirds. These findings unravel the complexity of ecological relationships and chemical communication mechanisms, accentuating the crucial roles of specific VOCs in shaping plant responses to infestations and the presence of natural protectors. The chemical and statistical results in Table 1, along with Fig. 3 indicate that the grapevine’s status-dependent compounds can differentiate between five out of six communities, as presented in Fig. 4.
Figure 4: The chemosphere compounds released by grapevine before and after A. illinoisensis infestation were analyzed by CLS-GC/MS and subjected to PCA analysis, with G1–G6 being referred to as Fig. 1
In noninfested grape plants (G1), VOCs represented by mesitylene (bio #7), hex-3-enyl acetate (#10), 3-hexenyl isobutyrate (#16), and 2,2,4-trimethyl-1,3-pentanediol diisobutyrate (#32) were detected in the chemosphere (Figs. 3B, 4). It’s worth noting that these VOCs either vanished or decreased significantly after infestation by A. illinoisensis (Table 1, Fig. 2).
3.4 Grapevine–Aphid Interaction (G2)
This system describes the interaction between the grapevine and A. illinoisensis. Grapevine plants are responding to aphids, which are adept sap feeders, by emitting VOCs into the chemosphere. Three significant biomarkers serve as indicators of infestation such as 4,8-dimethylnona-1,3,7-triene (bio #15), 2,6-dimethylocta-3,5,7-trien-2-ol (#20), and caryophyllene (#25) (Figs. 3B, 4). Any change in this community as a result of adding biotic variables affected the chemosphere profile, as noted in other communities (Fig. 3A).
3.5 Grapevine-Aphid-Ant Interaction (TPC1)
The presence of T. magnum in this community was indicated by the availability of bio benzenoids (bio #4, #5, #9), an aldehyde (#6), monoterpenes (#3, #12), sesquiterpenes (#26, #28, and #31), and esters (#17, #23) in the chemosphere (Table 1, Figs. 2A, 3B, 4). This unique VOC composition of this group can be attributed to the combined effect of grapevine, A. illinoisensis, and T. magnum, and was never detected in other communities even in low concentrations except for bio #17 (Table 1).
3.6 Grapevine-Aphid–Ladybird Interaction (TPC2)
The interaction between predators and prey is exemplified by the attack on A. illinoisensis by C. undecimpunctata, which could be attributed to the only significant biomarker, nonanal (bio #14) (Figs. 3, 4).
3.7 Grapevine-Aphid-Ant-Ladybird Interaction (QPC1/QPC2)
The quadripartite communities consist of all four organisms of the study’s interest regardless of the adding order of T. magnum or C. undecimpunctata (QPC1, QPC2) were considered as one group by PCA (Fig. 3) and characterized by a ketone (bio#2), an aldehyde (#19), one monoterpene (#24), and sesquiterpenes (#30, #33), an ester (#22), and an alkane (#13). Despite the nonsignificant differences observed by the heatmap (Fig. 2A) and the column chart (Fig. 2B) show differences between the two quadrant communities.
4.1 Understanding the Chemical Ecology of A. Illinosensis-Infested Grapevine
In this complex network of interactions in the grapevine-aphid-ant-ladybird system, the exact balance between ecological dynamics and chemical signaling is important in structuring the ecosystem’s stability and functioning [35]. The intricate relationships among these organisms reflect their complex interplay from ecological and chemical perspectives.
The significant alternation of chemosphere among grapevine treatments as was noticed in Table 1, Fig. 2 demonstrated that VOCs play many roles in plant defense mechanisms, and attract insects [36,37]. Benzenoids and esters were detected as VOCs in the chemosphere of intact V. vinifera leaves (G1) as predominant classes. Similar results were seen in Rodríguez-Declet et al. [38], with alcohol and hydrocarbon as the most prominent class emitted from leaves of grapevine cv. Isabella. Exposure to various biotic variables, like A. illinosensis, T. magnum, and C. undecimpunctata, resulted in significant qualitative and quantitative alterations in the emitted VOCs in the chemosphere (Table 1, Fig. 2). Aphid, proficient sap feeders, initiate an ongoing response in grapevine plants by emitting VOCs into the chemosphere [1,39] as clearly witnessed in all other groups (G2–G6) (Table 1, Fig. 2). After infestation with A. illinosensis (G2), the presence of sesquiterpenes including the alarm pheromone (bio #27: β-farnesene), alkenes, and the absence of benzenoids distinguished this community. These VOCs act as chemical signals, alerting both the plant and other organisms to the presence of a stressor. The plant’s release of these compounds is an immune response to herbivore attacks, stimulating chemical defenses and attracting natural enemies, including the ladybird [40]. Initially, A. illinosensis-infested grapevine (G2) was the site of detection for the alarm pheromone (β-farnesene-bio #27), and it persisted in TPC1(G3), and TPC2 (G4) after T. magnum, and C. undecimpunctata addition, respectively, whereas β-farnesene was absent in both quadripartite communities (QC1,2) (Table 1, Fig. 2A,C, Fig. 3B). In addition, the emissions of another farnesene isomer (α-farnesene, bio #30), and its hydroxyl derivative (7-hydroxy farnesene, bio #33) were exclusively observed in all C. undecimpunctata-associated treatments (G4–G6), and QPCs (G5, G6), respectively (Table 1, Fig. 2A,C, Fig. 4). Although the reproductive organs of grapes emit α-farnesene, which acts as a cue for grape moth oviposition [41], further investigations are required to determine its biological functions under the study’s conditions. β-farnesene can be produced by attacked plants as a semiochemical that attracts natural enemies, or it can be generated by aphids as an alarm pheromone [42]. Aphids release β-farnesene that is too low for predators to detect over a certain distance because each aphid releases nanograms, from 1 to 2, and up to 50 [42]. Therefore, the predator’s ability to detect this volatility from a distance and before aphids are disturbed is limited due to β-farnesene emissivity in the presence of danger [43]. Thus, we can argue that the detected β-farnesene in TPC2 (Table 1, Fig. 2A) is an A. illinosensis-induced grapevine volatile, as is also the case in G2, and TPC1. Notably, a study by Verheggen et al. [44] revealed that the ant Lasius niger utilizes β-farnesene in low amounts to find aphid colonies and facilitate communication between aphids and ants, which could be a reflection of the situation between A. illinosensis-T. magnum in TPC1. The absence of β-farnesene in QPC1, and QPC2 (Table 1, Fig. 2A,C) is likely to be attributed to (1) the experiment’s workflow depicted in Fig. 1 involved collecting VOCs from quadripartite communities after 96 h, which is long enough for β-farnesene volatilization to decline exponentially, as discussed comprehensively by Vandermoten and coauthors [45], (2) larger predator, such as ladybird, consumes its prey in its entirety, it can effectively stop an aphid’s alarm signal (β-farnesene), and it’s important to keep in mind that different predators feed differently, and this could affect the duration and strength of the signal as reported by Schwartzberg et al. [43]. These results support the notion that β-farnesene is a biomarker for A. illinoisensis-infested grapevine. β-farnesene was recently identified as a biomarker of A. illinoisensis due to exposure to mechanical stress [25]. Caryophyllene (bio #25) was detected in all infested groups regardless of the existence or the absence of ant and ladybird (G2–G4), but with less amount in QPCs (G5, G6) (Table 1, Fig. 2A,C). According to Alotaibi and coauthors [25], caryophyllene is present in the headspace profile of A. illinoisensis after mechanical stress. A study by Howard et al. [46] confirmed that plant caryophyllene has a detrimental effect on ant species. This is a sesquiterpene that is widely present in plants [47] and produced by multiple terpene synthases [48]. It is notable for its contribution to plant defense, which involves repelling spider mites and attracting herbivorous enemies either above or below ground [49]. The increase in aphid parasitoids in damaged tomato plants was attributed to one of the highest concentrations of caryophyllene released in aphid-infested tomatoes [50]. Similarly, in maize, caryophyllene attracts natural enemies of herbivores [48], and Aphidius colemani, a parasitic wasp of Aphis gossypii, was attracted to caryophyllene, and it was observed that it could have negative impacts on aphid fitness [51]. According to Fig. 2C, the decrease in caryophyllene release in QPCs (Fig. 2C) is probably due to aphid effectors that could alter multiple plant defense tools, including the emission of VOCs, as demonstrated in other plant-aphid interactions [52]. β-farnesene can be suppressed by the other VOCs in the chemosphere, including caryophyllene and germacrene D [53]. In our study, germacrene D (bio #29) was detected only in the chemosphere of A. illinosensis-infested grapevine (G2) (Fig. 2A,C). The separation of G3 was significantly influenced by the sesquiterpenes α-cubebene (bio #26), copaene (bio #28), and α-cadinene (bio #31) (Fig. 2A,C, Figs. 3, 4). Staudt et al. [54] identified α-cubebene as an aphid-induced VOC. Cadinene and copaene were reported as induced sesquiterpenes that deter whitefly Bemisia tabaci from settling on its host plants [55]. Germacrene D, α-cubebene, copaene,α-farnesene, α-cadinene, and 7-hydroxy farnesene were neither detected in the headspace profile nor as significant biomarkers of mechanically stressed A. illinoisensis [25].
Green leaf volatiles (GLVs) have a variety of biological functions, including herbivore defense, plant priming, pathogen defense, aroma, and flavor according to a review by Ul Hassan et al. [56]. Hex-3-enyl acetate (bio #10), and nonanal (bio #14) were two GLVs detected in the current study (Table 1, Fig. 2A). Hex-3-enyl acetate was identified in high concentration in intact grapevine (G1), and all treatments except for group-associated T. magnum, i.e., TPC1, QPC1 (very low concentration), and QPC2 (Table 1, Fig. 2A). Many studies shed light on the repelling effect of alkyl acetate esters on certain species of ants such as Tetramorium tsushimae [57], and Solenopsis invicta [58]. The disappearance of this compound in ant-containing treatments in this study and the anti-ant detection observed in previous studies provide a new avenue of research into this relationship. Nonanal was determined after 24 h of treating infested groups by C. undecimpunctata, represented by TPC2, QPC1, unlike TPC1, where C. undecimpunctata remained for 48 h (Table 1, Fig. 2A). Nonanal is a source of attraction for ladybird species (Harmonia axyridis) as outlined by Xiu et al. [59].
The phenylpropanoid pathway product methyl salicylate (bio #17) was detected in TPC1 (Table 1, Fig. 2A), as a significant biomarker (Figs. 3, 4), whereas benzaldehyde (bio #6) was detected in TPC1, 2 (Table 1). These two volatiles were also detected in the headspace profile of mechanically stressed A. illinoisensis by Alotaibi et al. [25]. Plant defense against pathogens and certain herbivores, particularly aphids, can be induced by methyl salicylate [60]. Lacewing, an aphid predator, is attracted to benzaldehyde [61].
4.2 Diagnosis of Grapevine Infestation by A. illinoisensis
The use of a specific ecological statistic tool (Fig. 3) simplified our understanding of complex relationships that occur during grapevine infestation by A. illinoisensis. This comprehension applies to non-destructive early-stage diagnosis of the aphid organism. Furthermore, it can aid entomologists in tracking the status of infestation chemically and precisely by detecting the significant biomarkers that resulted from PCA analysis (Fig. 3), as explained in Fig. 4. Focusing on the significant biomarkers in each chemosphere and the differences in their profile emissions can be beneficial for aphid management. If there are three esters (i.e., hex-3-enylacetate, 2,2,4-trimethyl-1,3-pentanedioldiisobutyrate, and cis-3-hexenylisobutyrate) and one benzene derivative (mesitylene) in abundance (Fig. 4), it can be concluded that the grapevine is noninfested by aphid (G1). Benzenoids and certain esters are crucial for plant signaling and defense mechanisms [62]. In comparison, the appearance of germacrene D, (E)-4,8-dimethylnona-1,3,7-triene, and (3E,5E)-2,6-dimethylocta-3,5,7-triene-2-ol (Fig. 4) is the rapid and most reliable indicator of A. illinoisensis infestation in grapevine (G2). The presence of T. mangum in the infested grapevine can be indicated by two monoterpenes, three specific sesquiterpenes, and six benzene derivatives, including three alkyl benzenes, 2-phenylethyl benzeneacetate, benzaldehyde, and methyl salicylate (Fig. 4). Aphids emit honeydew, a sugary secretion relayed by ants as a food source [63]. In response, ants protect aphids [64,65]. The chemical cues emitted by aphids and their honeydew guide ants to aphid colonies, establishing a chemical dialogue that coordinates this symbiotic relationship [66,67]. The detection limit could be the cause of the lack of n-alkanes in TPC1, as ants rely on aphid cuticular n-alkanes to identify aphid species [68]. In that regard, complex mutualism between honeydew-producing hemipterans and ants, manifesting both ecologically and chemically is important for ecology and evolution in terrestrial habitats [69], but they can also lead to pest infestations in agriculture [69,70]. The chemical signals between these two organisms serve as communication channels vital for maintaining this mutualism [71]. Simultaneously, ants are usually active predators of various agricultural pests, can enhance the quality of soil, and can manage certain plant pathogens [70,72]. Due to the mutualistic interaction between T. magnum and A. illinoisensis, the deterrent interaction between grapevine and A. illinoisensis, in addition to the interaction between grapevine and T. magnum, TPC1 was significantly separated from the other groups (Fig. 3A). The sole evidence of C. undecimpunctata presence in the infested system (TPC2) is nonanal (Figs. 3B, 4). As exemplified by C. undecimpunctata, ladybirds act as significant aphid predators, playing a crucial role in natural pest control [73]. Chemical signals released by aphid-infested plants attract ladybirds to the scene, optimizing their predatory efficiency [74]. This interplay between chemical signals and predator-prey interactions emphasizes the importance of chemical communication in regulating aphid populations and structuring ecosystem dynamics [75]. The quadripartite communities (QPCs) were distinguished by three aldehydes (citral, decanal), two hepta-ketones (2-methyl-4-heptanone, 6-methyl-5-hepten-2-one), alarm pheromone isomer and its derivative (α-farnesene, 7-hydroxyfarnesene), an ester (3-methyl hexyl butanoate), and a methyl alkane (4,5-dimethylnonane) (Fig. 4). The coexistence of aphids, ants, and ladybirds further increases the complexity of the interactions, due to the multi-relationships which take place within this community [76,77]. Chemical communication intricately shapes aphid feeding behavior, custodial actions, and ladybird predation [78]. The resulting modulation of volatile compounds creates a subtle and intricate volatile profile within the ecosystem [79]. The presence of both ladybirds and ants appeared to affect the composition of these compounds, possibly signifying their correlated impact on plant-insect interactions [80]. By combining the interactions, a dynamic equilibrium defines the grapevine-aphid-ant-ladybird complex. The response of grapevine to aphid attack triggers a cascade of chemical signals, influencing ladybird behavior and altering ant activities in response to the chemically transformed environment [81]. This intricate interplay of ecological and chemical interactions emphasizes the system’s resilience and the complexity of its defense mechanisms [82]. Despite the discussed interactions which are portrayed by grapevine-aphid (G2), aphid-ant (TPC1), and aphid-ladybird (TPC2), the ant-ladybird interactions have a significant impact on the VOC emissions and can manifest both directly and indirectly as demonstrated by Majerus et al. [77]. Ants’ behavior towards ladybirds may impact ladybird predation activities, influencing their efficiency in controlling aphid populations [83]. In contrast, ants might perceive ladybirds as potential disruptors of their mutualistic association with aphids, prompting them to deter or eliminate ladybirds [84]. These interactions emphasize the complex interplay of chemical cues in shaping community dynamics.
For confirmation, biomarkers have to be evaluated through electrophysiological and behavioral studies to determine their bioactivity. It’s worth considering that the combination of VOCs can result in a unique behavioral reaction to specific compounds. For example, (Z)-3-hexen-1-ol was only attractive in the olfactory sensor during the compound testing, despite being less active than the complete mixture [85]. At levels close to the natural release rates, each compound was capable of repelling according to the dose-response measurements in the olfactometer for all 15 compounds. The complete mixture was once again attractive when these levels were used to reassemble it.
We collected the VOCs in an enclosed space with limited natural airflow, which could lead to a faster rate of volatile emission into the chemosphere, compared to an open, natural system where air currents could cause a faster rate of volatile emission into the chemosphere. In our opinion, the natural dynamics are represented by the observed dynamics of VOC volatilization in this study. Our design enabled a nonbiased qualitative and quantitative comparison of VOC emissions between the chemosphere of six different treatments of grapevine V. vinifera, despite any slight differences in natural dynamics. These findings will greatly enhance our understanding of the chemical ecology of A. illinoisensis, and thus contribute to aphid management by getting use of the characteristic biomarkers of each infestation status that the A. illinoisensis-infested grapevine goes through. Variations in climate, soil, and different aphid species in other areas could impact the observed interactions. Increasing the sample size and replication will be considered in further studies to enhance the statistical robustness of the results. More ecological and long-term researches are required to improve and develop sustainable management strategies.
This study revealed notable changes in volatile compound profiles due to aphids, and the associated organisms during the grapevine infestation. The distinct biomarkers are a key determinant of the infestation status of grapevine by A. illinoisensis. The chemosphere often differs between the five statuses of the grapevine. Thus, the study results can determine the status of the grapevine, whether it is intact, infested by A. illinoisensis, infested in the presence of ants, infested in the presence of ladybirds, or infested in the presence of ants and ladybirds. These differences in chemosphere would ideally be used for (1) non-destructive early-stage diagnosis of the aphid, (2) sustainable management of ant-aphid associations, and (3) understanding of the success of predator-prey dynamics for biological control. This study provides valuable insight into grapevine protection and effective aphid management approaches which are decisive in constructing biomarker-based decision support systems for the control of aphids.
Acknowledgement: The authors extend their appreciation to the Deputyship for Research and Innovation, Ministry of Education in Saudi Arabia, for funding this research through Project Number 1/441/119.
Funding Statement: The authors would like to acknowledge the Deputyship for Research and Innovation, Ministry of Education in Saudi Arabia, for funding this research through Project Number 1/441/119.
Author Contributions: Conceptualisation: Taghreed Alsufyani, Noura J. Alotaibi, Nour Houda M’sakni; methodology: Taghreed Alsufyani, Noura J. Alotaibi, Nour Houda M’sakni, Mona A. Almalki; data analysis: Taghreed Alsufyani, Noura J. Alotaibi, Nour Houda M’sakni, Mona A. Almalki, Eman M. Alghamdi; draft preparation: Taghreed Alsufyani, Noura J. Alotaibi, Nour Houda M’sakni, Mona A. Almalki, Eman M. Alghamdi; writing–review and editing: Taghreed Alsufyani, Noura J. Alotaibi, Nour Houda M’sakni, Mona A. Almalki, Eman M. Alghamdi; project administration: Taghreed Alsufyani, Noura J. Alotaibi; funding acquisition: Taghreed Alsufyani. All authors reviewed the results and approved the final version of the manuscript.
Availability of Data and Materials: Sequencing data were deposited at the National Center for Biotechnology Information: NCBI repository (https://www.ncbi.nlm.nih.gov/nucleotide/, accessed on 05/04/2022).
Ethics Approval: Not applicable.
Conflicts of Interest: The authors declare that they have no conflicts of interest to report regarding the present study.
References
1. Singh S, Acevedo FE. Grapevine plant defense responses associated with arthropod herbivory: a review. Crop Prot. 2024;177:10655. [Google Scholar]
2. Fahmi AI, Nagaty MA, El-Shehawi AM. Fruit quality of Taif grape (Vitis vinifera L.) cultivars. J Am Sci. 2012;8(5):590–9. [Google Scholar]
3. Filocamo A, Bisignano C, Mandalari G, Navarra M. In vitro antimicrobial activity and effect on biofilm production of a white grape juice (Vitis vinifera) extract. Evid Based Complement Alternat Med. 2015:856243. [Google Scholar]
4. Goufo P, Singh RK, Cortez I. A reference list of phenolic compounds (including stilbenes) in grapevine (Vitis vinifera L.) roots, woods, canes, stems, and leaves. Antioxidants. 2020;9(5):398. [Google Scholar] [PubMed]
5. Walton VM, Daane KM, Addison P. Biological control of arthropods and its application in vineyards. In: Arthropod management in vineyards: pests, approaches, and future directions. Heidelberg, Germany: Springer; 2012. p. 91–117. [Google Scholar]
6. Venios X, Korkas E, Nisiotou A, Banilas G. Grapevine responses to heat stress and global warming. Plants. 2020;9(12):1754. [Google Scholar] [PubMed]
7. Guzun NI, Nedov PN, Usatov VT, Kostrikin IA, ML F. Grape selection for resistance to biotic and abiotic environmental factors. J Grapevine Res. 1990;29:219–22. [Google Scholar]
8. Yactayo-Chang JP, Tang HV, Mendoza J, Christensen SA, Block AK. Plant defense chemicals against insect pests. Agronomy. 2020;10(8):1156. doi:10.3390/agronomy10081156. [Google Scholar] [CrossRef]
9. Zvereva EL, Vc Lanta, Kozlov MV. Effects of sap-feeding insect herbivores on growth and reproduction of woody plants: a meta-analysis of experimental studies. Oecologia. 2010;163:949–60. doi:10.1007/s00442-010-1633-1. [Google Scholar] [PubMed] [CrossRef]
10. Croteau R. Biosynthesis of benzaldehyde, benzyl alcohol and benzyl benzoate from benzoic acid in cranberry (Vaccinium macrocarpon). J Food Biochem. 2007;1:317–26. doi:10.1111/j.1745-4514.1978.tb00190.x. [Google Scholar] [CrossRef]
11. Bernays E, Chapman R. The role of food plants in the survival and development of Chortoicetes terminifera (Walker) under drought conditions. Aust J Zool. 1973;21(4):575–92. doi:10.1071/ZO9730575. [Google Scholar] [CrossRef]
12. Cooper PD, Truong TT, Keszei A, Neeman T, Webster KW. The effect of scale insects on growth parameters of cv. Chardonnay and cv. Sauvignon blanc grapevines grown in a greenhouse. Int J Mol Sci. 2023;24(2):1544. doi:10.3390/ijms24021544. [Google Scholar] [PubMed] [CrossRef]
13. Zhou S, Lou YR, Tzin V, Jander G. Alteration of plant primary metabolism in response to insect herbivory. Plant Physiol. 2015;169(3):1488–98. doi:10.1104/p.15.01405. [Google Scholar] [CrossRef]
14. Meyer GA, Whitlow TH. Effects of leaf and sap feeding insects on photosynthetic rates of goldenrod. Oecologia. 1992;92(4):480–9. doi:10.1007/BF00317839. [Google Scholar] [PubMed] [CrossRef]
15. Norkute M, Olsson U, Ninkovic V. Aphids-induced plant volatiles affect diel foraging behavior of a ladybird beetle Coccinella septempunctata. Insect Sci. 2020;27(6):1266–75. doi:10.1111/1744-7917.12734. [Google Scholar] [PubMed] [CrossRef]
16. Goggin FL. Plant-aphid interactions: molecular and ecological perspectives. Current Opin Plant Biol. 2007;10(4):399–408. doi:10.1016/j.pbi.2007.06.004. [Google Scholar] [PubMed] [CrossRef]
17. Vela J, Montiel EE, Mora P, Lorite P, Palomeque T. Aphids and ants, mutualistic species, share a mariner element with an unusual location on aphid chromosomes. Genes. 2021;12(12):1966. [Google Scholar] [PubMed]
18. Koutsoula G, Stamkopoulou A, Pekas A, Wäckers F, Broufas G, Pappas ML. Predation efficiency of the green lacewings Chrysoperla agilis and C. mutata against aphids and mealybugs in sweet pepper. Bull Entomol Res. 2023;113(2):162–8. doi:10.1017/S0007485322000426. [Google Scholar] [PubMed] [CrossRef]
19. Riddick EW. Identification of conditions for successful aphid control by ladybirds in greenhouses. Insects. 2017;8(2):38. doi:10.3390/insects8020038. [Google Scholar] [PubMed] [CrossRef]
20. Hodek I, Honek A, Van Emden HF. Ecology and behaviour of the ladybird beetles (Coccinellidae). New York City, USA: John Wiley & Sons; 2012. [Google Scholar]
21. Ghosh SK. Chapter-3 population of lady bird beetle on vegetable crops and use of safe pesticides for biodiversity conservation. Entomology. 2022;27:41. [Google Scholar]
22. Rashed H. Efficiency of the convergent ladybird beetle Hippodamia convergens against the legume aphid Aphis craccivora in laboratory and semi-felid conditions. Annals Agric Sci, Moshtohor. 2020;58(3):655–64. doi:10.21608/assjm.2020.131640. [Google Scholar] [CrossRef]
23. Lawo NC, Weingart GJ, Schuhmacher R, Forneck A. The volatile metabolome of grapevine roots: first insights into the metabolic response upon phylloxera attack. Plant Physiol Biochem. 2011;49(9):1059–63. [Google Scholar] [PubMed]
24. Filgueiras CC, Martins AD, Pereira RV, Willett DS. The ecology of salicylic acid signaling: primary, secondary and tertiary effects with applications in agriculture. Int J Mol Sci. 2019;20(23):5851. [Google Scholar] [PubMed]
25. Alotaibi NJ, Alsufyani T, M’Sakni NH, Almalki MA, Alghamdi EM, Spiteller D. Rapid identification of aphid species by headspace GC-MS and discriminant analysis. Insects. 2023;14(7):589. [Google Scholar] [PubMed]
26. Gerós HN, Chaves MM, Gil HM, Delrot S. Grapevine in a changing environment: a Molecular and ecophysiological perspective. New York City, USA: John Wiley & Sons; 2015. [Google Scholar]
27. Takabayashi J, Dicke M, Posthumus MA. Volatile herbivore-induced terpenoids in plant-mite interactions: variation caused by biotic and abiotic factors. J Chem Ecol. 1994;20:1329–54. [Google Scholar] [PubMed]
28. Vosteen I, Weisser W, Kunert G. Is there any evidence that aphid alarm pheromones work as prey and host finding kairomones for natural enemies? Ecol Entomol. 2016;41:1–12. [Google Scholar]
29. Basu S, Clark RE, Fu Z, Lee BW, Crowder DW. Insect alarm pheromones in response to predators: ecological trade-offs and molecular mechanisms. Insect Biochem Mol Biol. 2021;128:103514. [Google Scholar] [PubMed]
30. Alsufyani T, Al-Otaibi N, Alotaibi NJ, M’sakni NH, Alghamdi EM. GC analysis, anticancer, and antibacterial activities of secondary bioactive compounds from endosymbiotic bacteria of pomegranate aphid and its predator and protector. Molecules. 2023;28(10):4255. [Google Scholar] [PubMed]
31. Kunert M, David A, Becher J, Boland W. Volatile sampling from biological sources by the closed-loop-stripping technique. Cold Spring Harb Protoc; 2009. doi:10.1101/pdb.prot5233. [Google Scholar] [PubMed] [CrossRef]
32. Chehab E, Kaspi R, Savchenko T, Dehesh K. Hexenyl acetate mediates indirect plant defense responses. Biol Sci. 2010;65:145–51. [Google Scholar]
33. Alsufyani T, Weiss A, Wichard T. Time course exo-metabolomic profiling in the green marine macroalga Ulva (Chlorophyta) for identification of growth phase-dependent biomarkers. Mar Drugs. 2017;15(1):14. [Google Scholar] [PubMed]
34. Prieto N, Manful C, Pham TH, Stewart P, Keough D, Thomas R. The use of XLSTAT in conducting principal component analysis (PCA) when evaluating the relationships between sensory and quality attributes in grilled foods. MethodsX. 2020;7:100835. doi:10.1016/j.mex.2020.100835. [Google Scholar] [PubMed] [CrossRef]
35. Lindroth RL. Impacts of elevated atmospheric CO2 and O3 on forests: phytochemistry, trophic interactions, and ecosystem dynamics. J Chem Ecol. 2010;36:2–21. doi:10.1007/s10886-009-9731-4. [Google Scholar] [PubMed] [CrossRef]
36. Alem H, Rigou P, Schneider RM, Ojeda HN, Torregrosa L. Impact of agronomic practices on grape aroma composition: a review. J Sci Food Agric. 2019;99(3):975–85. doi:10.1002/jsfa.9327. [Google Scholar] [PubMed] [CrossRef]
37. Bezerra RHS, Sousa-Souto L, Santana ANEBG, Ambrogi BG. Indirect plant defenses: volatile organic compounds and extrafloral nectar. Arthropod-Plant Interaction. 2021;15(4):467–89. doi:10.1007/s11829-021-09837-1. [Google Scholar] [CrossRef]
38. Rodríguez-Declet A, Castro-Marín A, Moretti Conti L, Lombini A, Chinnici F, Rombolà AD. Characterization of VOCs emitted by foliage of grapevine cv. isabella for prospecting innovative cropping systems. Agronomy. 2022;12(2):272. [Google Scholar]
39. Markheiser A, Rid M, Biancu S, Gross J, Hoffmann C. Tracking short-range attraction and oviposition of european grapevine moths affected by volatile organic compounds in a four-chamber olfactometer. Insects. 2020;11(1):45. doi:10.3390/insects11010045. [Google Scholar] [PubMed] [CrossRef]
40. Hammerbacher A, Coutinho TA, Gershenzon J. Roles of plant volatiles in defence against microbial pathogens and microbial exploitation of volatiles. Plant, Cell Environ. 2019;42(10):2827–43. doi:10.1111/pce.13602. [Google Scholar] [PubMed] [CrossRef]
41. Chalal M, Winkler JB, Gourrat K, Trouvelot S, Adrian M, Schnitzler JP, et al. Sesquiterpene volatile organic compounds (VOCs) are markers of elicitation by sulfated laminarine in grapevine. Front Plant Sci. 2015;6:1118. doi:10.3389/fpls.2015.00350. [Google Scholar] [PubMed] [CrossRef]
42. Wang B, Dong W, Li H, D’Onofrio C, Bai P, Chen R, et al. Molecular basis of (E)-β-farnesene-mediated aphid location in the predator Eupeodes corollae. Current Biol. 2022;32(5):951–62.e7. doi:10.1016/j.cub.2021.12.054. [Google Scholar] [PubMed] [CrossRef]
43. Schwartzberg EG, Kunert G, Stephan C, David A, Röse USR, Gershenzon J, et al. Real-time analysis of alarm pheromone emission by the pea aphid (Acyrthosiphon Pisum) under predation. J Chem Ecol. 2008;34(1):76–81. doi:10.1007/s10886-007-9397-8. [Google Scholar] [PubMed] [CrossRef]
44. Verheggen FJ, Diez L, Sablon L, Fischer C, Bartram S, Haubruge E, et al. Aphid alarm pheromone as a cue for ants to locate aphid partners. PLoS One. 2012;7(8):e41841. [Google Scholar] [PubMed]
45. Vandermoten S, Mescher MC, Francis F, Haubruge E, Verheggen FJ. Aphid alarm pheromone: an overview of current knowledge on biosynthesis and functions. Insect Biochem Mol Biol. 2012;42(3):155–63. [Google Scholar] [PubMed]
46. Howard JJ, Green TP, Wiemer DF. Comparative deterrency of two terpenoids to two genera of attine ants. J Chem Ecol. 1989;15(9):2279–88. [Google Scholar] [PubMed]
47. Cai Y, Jia JW, Crock J, Lin ZX, Chen XY, Croteau R. A cDNA clone for β-caryophyllene synthase from Artemisia annua. Phytochemistry. 2002;61(5):523–9. [Google Scholar] [PubMed]
48. Köllner TG, Held M, Lenk C, Hiltpold I, Turlings TCJ, Gershenzon J, et al. A maize (E)-β-caryophyllene synthase implicated in indirect defense responses against herbivores is not expressed in most american maize varieties. Plant Cell. 2008;20(2):482–94. [Google Scholar]
49. da Câmara C, Akhtar Y, Isman M, Seffrin R, Born F. Repellent activity of essential oils from two species of Citrus against Tetranychus urticae in the laboratory and greenhouse. Crop Prot. 2015;74:110–15. [Google Scholar]
50. Guerrieri E, Digilio MC. Aphid-plant interactions: a review. J Plant Interactions. 2008;3:223–32. [Google Scholar]
51. Vitiello A, Molisso D, Digilio MC, Giorgini M, Corrado G, Bruce TJA, et al. Zucchini plants alter gene expression and emission of (E)-β-caryophyllene following Aphis gossypii infestation. Front Plant Sci. 2020;11:592603. [Google Scholar] [PubMed]
52. Schwartzberg EG, Böröczky K, Tumlinson JH. Pea aphids, Acyrthosiphon pisum, suppress induced plant volatiles in broad bean, Vicia faba. J Chem Ecol. 2011;37(10):1055–62. [Google Scholar] [PubMed]
53. Bruce TJ, Birkett MA, Blande J, Hooper AM, Martin JL, Khambay B, et al. Response of economically important aphids to components of Hemizygia petiolata essential oil. Pest Manage Sci. 2005;61(11):1115–21. [Google Scholar] [PubMed]
54. Staudt M, Jackson B, El-Aouni H, Buatois B, Lacroze JP, Poëssel JL, et al. Volatile organic compound emissions induced by the aphid Myzus persicae differ among resistant and susceptible peach cultivars and a wild relative. Tree Physiol. 2010;30(10):1320–34. [Google Scholar] [PubMed]
55. Saad KA, Mohamad Roff MN, Hallett RH, Idris AB. Aphid-induced defences in chilli affect preferences of the whitefly, Bemisia tabaci (Hemiptera: Aleyrodidae). Sci Rep. 2015;5:13697. [Google Scholar] [PubMed]
56. Ul Hassan MN, Zainal Z, Ismail I. Green leaf volatiles: biosynthesis, biological functions and their applications in biotechnology. Plant Biotechnol J. 2015;13(6):727–39. [Google Scholar] [PubMed]
57. Ohba S-Y, Maeda A. Paternal care behaviour of the giant water bug Kirkaldyia deyrolli (Heteroptera: Belostomatidae) against ants: paternal care by the giant water bug. Ecol Entomol. 2017;42:402–10. [Google Scholar]
58. Du Y, Zhou A, Chen J. Olfactory and behavioral responses to acetate esters in red imported fire ant, Solenopsis invicta. Pest Manage Sci. 2021;77(3):1371–82. [Google Scholar] [PubMed]
59. Xiu C, Xu B, Pan HS, Zhang W, Yang YZ, Lu Y. Volatiles from Sophora japonica flowers attract Harmonia axyridis adults (Coleoptera: coccinellidae). J Integr Agric. 2019;18:873–83. [Google Scholar]
60. Ninkovic V, Glinwood R, Ünlü AG, Ganji S, Unelius CR. Effects of methyl salicylate on host plant acceptance and feeding by the Aphid Rhopalosiphum padi. Front Plant Sci. 2021;12:710268. [Google Scholar] [PubMed]
61. Badra Z, Larsson Herrera S, Cappellin L, Biasioli F, Dekker T, Angeli S, et al. Species-specific induction of plant volatiles by two aphid species in apple: real time measurement of plant emission and attraction of lacewings in the wind tunnel. J Chem Ecol. 2021;47(7):653–63. [Google Scholar] [PubMed]
62. Bhavaniramya S, Vishnupriya S, Al-Aboody MS, Vijayakumar R, Baskaran D. Role of essential oils in food safety: antimicrobial and antioxidant applications. Grain Oil Sci Technol. 2019;2(2):49–55. [Google Scholar]
63. McCallum S. The secret life of an arable field: plants, animals and the ecosystem. White Owl; 2021. https://www.pen-and-sword.co.uk/The-Secret-Life-of-an-Arable-Field-Hardback/p/19262. [Accessed 2024]. [Google Scholar]
64. Tegelaar K, Hagman M, Glinwood R, Pettersson J, Leimar O. Ant-aphid mutualism: the influence of ants on the aphid summer cycle. Oikos. 2011;121:61–6. [Google Scholar]
65. Tizado E, Tinaut A, Nieto Nafría JM. Relationships between ants and aphids in the province of Leon (Spain) (Hym: formicidae; Hom: aphididae). Vie et Milieu. 1993;43:63–8. [Google Scholar]
66. Eaton ER. Insectpedia: a brief compendium of insect lore. New Jersey, USA: Princeton University Press; 2022. [Google Scholar]
67. Senft M, Clancy M, Weisser W, Schnitzler JP, Zytynska S. Additive effects of plant chemotype, mutualistic ants and predators on aphid performance and survival. Funct Ecol. 2018;33:139–51. [Google Scholar]
68. Lang C, Menzel F. Lasius niger ants discriminate aphids based on their cuticular hydrocarbons. Anim Behav. 2011;82(6):1245–54. [Google Scholar]
69. Lescano MN, Quintero C, Farji-Brener AG, Balseiro E. Excessive nutrient input induces an ecological cost for aphids by modifying their attractiveness towards mutualist ants. Funct Ecol. 2022;36(10):2661–72. [Google Scholar]
70. Schifani E, Giannetti D, Grasso D. Toward sustainable management of ant-hemipteran mutualism in agricultural settings: a comparison of different approaches. Crop Prot. 2024;175:106468. [Google Scholar]
71. Xu T, Chen L. Chemical communication in ant-hemipteran mutualism: potential implications for ant invasions. Curr Opin Insect Sci. 2021;45:121–29. [Google Scholar] [PubMed]
72. Rico-Gray V, Oliveira PS. The ecology and evolution of ant-plant interactions. Chicago, USA: University of Chicago Press; 2008. [Google Scholar]
73. Serrão JE, Plata-Rueda A, MartÃnez LC, Zanuncio JC. Side-effects of pesticides on non-target insects in agriculture: a mini-review. Sci Nat. 2022;109(2):17. [Google Scholar]
74. Pervez A, Yadav M. Foraging behaviour of predaceous ladybird beetles: a review. Eur J Environ Sci. 2018;8(2):102–8. [Google Scholar]
75. Draper AM, Weissburg MJ. Impacts of global warming and elevated CO2 on sensory behavior in predator-prey interactions: a review and synthesis. Front Ecol Evol. 2019;7:72. [Google Scholar]
76. Silva E, Perfecto I. Coexistence of aphid predators in cacao plants: does ant-aphid mutualism play a role? Sociobiology. 2013;60:259–65. [Google Scholar]
77. Majerus MEN, Sloggett JJ, Godeau JF, Hemptinne JL. Interactions between ants and aphidophagous and coccidophagous ladybirds. Popul Ecol. 2007;49(1):15–27. [Google Scholar]
78. Price PW, Denno RF, Eubanks MD, Finke DL, Kaplan I. Insect ecology: behavior, populations and communities. Cambridge, UK: Cambridge University Press; 2011. [Google Scholar]
79. Ninkovic V, Markovic D, Rensing M. Plant volatiles as cues and signals in plant communication. Plant, Cell Environ. 2021;44(4):1030–43. [Google Scholar] [PubMed]
80. Srisakrapikoop U. Consequences of host quality variation for the behaviour, life histories and ecological interactions of insects at higher trophic levels. Berkshire, England: University of Reading; 2021. [Google Scholar]
81. Vale PF, Siva-Jothy J, Morrill A, Forbes MR. The influence of parasites on insect behavior. In: Insect behavior: from mechanisms to ecological and evolutionary consequences. Oxford, England: Oxford Academic; 2018. p. 274–91. [Google Scholar]
82. Gunderson LH, Allen CR, Holling CS. Foundations of ecological resilience. Washington, DC, USA: Island Press; 2012. [Google Scholar]
83. Devegili ASM, Lescano MAN, Gianoli E, Farji-Brener AG. Defence variation within a guild of aphid-tending ants explains aphid population growth. Ecol Entomol. 2020;45(5):1180–9. [Google Scholar]
84. Moura RF, Colberg E, Alves-Silva EO, Mendes-Silva I, Fagundes R, Stefani V, et al. Biotic defenses against herbivory. In: Plant-animal interactions: source of biodiversity. London, UK: Springer; 2021. p. 93–118. [Google Scholar]
85. Pickett JA, Aradottir GI, Birkett MA, Bruce TJA, Chamberlain K, Khan ZR, et al. Aspects of insect chemical ecology: exploitation of reception and detection as tools for deception of pests and beneficial insects. Physiol Entomol. 2012;37(1):2–9. [Google Scholar]
Cite This Article
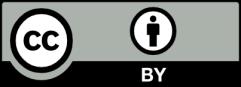
This work is licensed under a Creative Commons Attribution 4.0 International License , which permits unrestricted use, distribution, and reproduction in any medium, provided the original work is properly cited.