Open Access
REVIEW
Endophytic Occupation in Nodules of Rhynchosia Plants from Semiarid Regions of Argentina
1 Facultad de Ciencias Exactas y Naturales, Universidad Nacional de La Pampa, Ruta Nacional 35, km 330, Santa Rosa, La Pampa, CP, 6300, Argentina
2 INTA EEA Anguil “Ing. Agr. Guillermo Covas” Ruta Nacional 5, km 580, Anguil, La Pampa, CC11, 6326, Argentina
* Corresponding Author: Graciela S. Lorda. Email:
(This article belongs to the Special Issue: Ecology of Rangelands in Argentina)
Phyton-International Journal of Experimental Botany 2024, 93(6), 1081-1099. https://doi.org/10.32604/phyton.2024.050762
Received 17 February 2024; Accepted 13 May 2024; Issue published 27 June 2024
Abstract
Beneficial microbes can improve soil health by promoting soil structure, nutrient cycling, and disease suppression. In addition, a wide array of rhizospheric microbes are responsible for producing metabolically active compounds including various types of plant growth regulators. So, microbial biodiversity studies could contribute to the improvement of agricultural practices in deprived areas, such as the Pampean semiarid region. The vast majority of studies conducted on endophytic microorganisms have focused on intensive crop legume species. In contrast, little attention has been paid to microorganisms of native legumes, whose ecology is not directly affected by human action. In this study, endophytic microorganisms isolated from root nodules of a selected native legume of the genus Rhynchosia were characterized. Viable isolates were studied with a focus on their plant growth-promoting rhizobacteria (PGPR) properties. Considering the edaphic characteristics of the Pampean semiarid region, the isolates obtained were evaluated for their ability to grow under three salt stress conditions (50, 100, and 200 mM NaCl) and four different pH values (6, 7, 8, and 9). Based on their PGPR activities, the selected strains were phylogenetically grouped using BOX-PCR. The results showed great variability among the isolates in terms of the characteristics studied. Native legumes manifested a wide endophytic variability and remarkable performance in PGPR activities. We conclude that they could be used as potential bioinoculants for legume cultivation, an excellent alternative to the use of chemical fertilizers that currently pollute the environment.Keywords
Arid and semiarid areas cover a large and growing portion of the land surface. Legumes are a significant component of these regions’ flora, and despite their high diversity, little is known about their endophytic microbiome [1]. In these semiarid regions, some particularly interesting legumes are currently developed for use in sustainable agriculture. The recent literature, which is extensive for agronomically temperate and subtropical species, shows how the legume-microorganism symbiosis is affected by environmental adversities such as drought, waterlogging, salinity, and low and high temperatures, as well as pH or low nutrient concentrations [1]. However, some or all of these adverse conditions are, and probably have been for several years, the natural environment of many legumes in arid and semiarid regions. Therefore, the microorganisms associated with them can establish symbiotic relationships under stressful environmental conditions [2]. For this reason, the selection of native strains that can promote plant growth and are well adapted to a wide range of adverse environmental conditions at a given site is the key to maximizing legume production [3]. Assessing plant-associated microbial diversity in semiarid regions could be an essential strategy for identifying plant growth-promoting bacteria (PGPB) that can successfully alleviate plant abiotic stress commonly present in such climates and support plant growth and development [4,5].
Perhaps now that the potential consequences of climate change are more widely understood and recognized, the vested interests of the developed world will turn to the study of plants that can live in harsh environments using more sustainable agricultural practices. The reintroduction and establishment of native herbaceous legumes in degraded and uncultivated areas is a valid alternative method for soil recovery and wind erosion prevention, in addition to providing good quality forage for ruminants, especially to maintain agricultural productivity in a future scenario of increased aridity [5]. New adapted forage species are needed in the central and southern semiarid regions of Argentina, as well as in many places around the world. Studies have been conducted on native legume species with valuable traits that could help ensure their persistence under intensive grazing [6]. Given this, the native legume of the genus Rhynchosia is highlighted as a promising candidate for this objective. The genus comprises about two hundred species, most of which are found in Africa, with about a quarter in America [7]. These woody species are not only useful as animal fodder and green manure but also have interesting medicinal uses for humans and animals [8].
There is a pressing need for a better understanding of the underlying factors that control soil-plant-microbe interactions. This knowledge will help to develop microbial strategies to improve crop yield and productivity. Some isolates may represent an alternative endosymbiotic partner to rhizobia, a field of knowledge that focuses on understanding microbial endophytes. Inside a healthy plant, there may be bacteria or fungi that are not necessarily pathogenic or associated with nodule formation. The literature on the diversity of endophytic microorganisms of Rhynchosia plants and their symbiotic effectiveness is still limited. Therefore, the strategy used to study the symbiotic partnerships for the different legumes has traditionally involved their isolation and cultivation from internal tissues of surface-sterilized nodules [4,9].
In this study, to gain a better understanding of the incidence and heterogeneity of bacterial groups present within nodules of natural legume-endophytic associations, we examined the microbial occupants inside nodules of wild legume species of the genus Rhynchosia collected in semiarid regions of Argentina, using the standard colony isolation method from nodules. In parallel, we analyzed the growth and development of this bacterial microflora under stress conditions and determined its plant growth-promoting properties.
2.1 Native Legume Selection for the Genus Rhynchosia
Samples of Rhynchosia legume were collected from four different locations in the central and southern semiarid regions of the Province of La Pampa, Argentina (Table 1).
2.2 Edaphic Characterization of Soils
Edaphic characterization was performed based on texture, phosphorus and nitrogen contents among the macronutrients. The Robinson method [10] was used to determine texture. Nitrogen was evaluated using the Kjeldahl method [11], while phosphorus was determined by the Bray-Kurtz N° 1 method [12].
2.3 Forage Capacity Assessment of Native Rhynchosia Legume
Forage capacity was evaluated based on both the total and aerial biomass by direct weight. Neutral detergent fiber (NDF) and acid detergent fiber (ADF) were determined using the sequential Goering and Van Soest method [13]. Dry matter digestibility (DMD) and metabolizable energy (ME) were calculated using Eqs. (1) and (2):
Protein content was measured by the Kjeldahl method [11].
2.4 Isolation of Culturable Endophytic Bacteria
Root segments bearing nodules were washed free of soil under running water, placed in a fine-mesh steel holder, and surface sterilized, as previously described with slight modifications [14]. Briefly, the nodules were sterilized by immersion in 70% ethanol for 1 min, followed by vigorous shaking in 5% sodium hypochlorite containing 0.01% Tween 80, and finally washed seven times with sterile distilled water. To verify correct surface sterilization, samples corresponding to the last wash water were seeded in Petri dishes with TY medium. Surface-sterilized root nodules were transferred to empty sterile plastic dishes and cut in half with a flamed scalpel. They were then squashed in 50–150 ml of sterile physiological saline solution, the volume varying in proportion to nodule size. The squashed nodule suspension was plated on TY agar (5 g/L tryptone, 3 g/L yeast extract, and 0.87 g/L CaCl2·2H2O). Plates were incubated at 28°C for up to 2 weeks. Each isolate strain was named according to the sampling area in La Pampa, Argentina (T = Toay; V = Victorica; S = Santa Rosa; R = Rucanelo).
2.5 Nodulation Ability of Bacterial Isolates
Rhynchosia seeds were surface sterilized by immersion in 70% ethanol for 30 s, followed by stirring in 2% sodium hypochlorite for 4 min, and rinsed in seven changes of sterile deionized water. Germinated seedlings were aseptically transferred to pots containing sterile carrier sand-vermiculite 1:2 mixtures, fed from the bottom, and watered regularly with sterilized distilled water containing nitrogen-free Fahraeus solution. Bacteria were inoculated by dispensing a 1 ml suspension of liquid culture in a TY broth medium (108 cells/ml). The assay was maintained in a greenhouse programmed for a 16-h daylight photoperiod at 23°C and 60% constant relative humidity. Plants were examined for nodule formation after 60 days. They were removed from the pots, the root systems were washed with water and the number of nodules per plant was determined. The experiment had a randomized complete block design with four replicates per tested isolate and one seedling per replicate. Three independent assays were performed. Pots were rotated every 4 days to avoid any border effects.
To identify isolates with the ability to form nodules, the nodC gene was amplified using the primers nodCF (5′-TGATYGAYATGGARTAYTGGCT-3′)/nodCR (5′-CGYGACAGCCANTCKCTATTG-3′) [15]. Colony polymerase chain reaction (colony PCR) was performed in a 20 µl reaction mixture containing 0.5 µM of each primer, 1X PCR buffer, 1.5 mM MgCl2, 0.04 mMdNTPs (Promega), and 1 U of Taq DNA polymerase (TransGen Biotech). To add the DNA, a small amount of bacterial colony was taken directly from the Petri dish using a sterile tip for each colony and placed in a PCR tube containing the master mix. The temperature profile was as follows: 95°C for 3 min; 35 cycles at 95°C for 1 min, 55°C for 1 min, 72°C for 2 min, and finally 72°C for 10 min. PCR amplification was performed using a thermal cycler (Ivema T21). PCR products were separated on 1.5% (w/v) agarose gels by horizontal electrophoresis and visualized using SYBR Safe staining (Invitrogen). The Ensifer meliloti B399 strain was used as the positive control.
2.6 Determination of PGPR Characteristics of the Isolated Bacteria
2.6.1 Indole-3-Acetic Acid Production
Production of indole-3-acetic acid (IAA) was colorimetrically determined by mixing 5 ml Salkowski reagent (0.01 M FeCl2 in HClO4) with 1 ml of culture supernatant and then monitoring the color changes [16]. Pure indole-3-acetic acid (Sigma, USA) was used as the standard.
Siderophore production was determined by the method of Schwyn et al. [17]; 10 µl of pure bacterial cultures grown in LB were inoculated onto plates containing Chrome Azurol S (CAS) agar. Plates were incubated at 30°C and observed daily for orange color formation around each colony for up to 4 days. Experiments were performed in triplicate. A positive control was made with Pseudomonas fluorescens strain P3 (provided by the Agricultural Collection Laboratory, IMYZA-INTA, Argentina), under similar culture conditions.
2.6.3 Phosphate Solubilization
Phosphate solubilization was determined by the methods of Katznelson et al. [18]. Plates containing NBRIP medium were inoculated with 10 µl of LB pure bacterial culture. The plates were incubated at 30°C and observed daily for 7 days until a transparent zone appeared around each colony. Experiments were performed in triplicate. Positive control was made with Pseudomonas fluorescens strain P3 (provided by the Agricultural Collection Laboratory, IMYZA-INTA, Argentina) under similar culture conditions.
2.7 Growth of Isolates under Stress Conditions: pH and Osmolarity
Liquid cell cultures were performed under different osmolarity and pH conditions. TY was used as the culture medium. Specific growth rate (μ) was determined in each culture and used as a kinetic parameter to characterize cell growth. The following treatments were used:
-Control treatments: Cultures were grown at pH 7 without NaCl.
-Stress for pH treatments: Different cultures were grown at pH 6, 8, and 9.
-Stress for osmolarity treatments: Cultures were grown under different salinity conditions as described by Abdelmoumen et al. [19], as follows: 50, 100, and 200 mM NaCl.
These cultures were kept under optimal temperature and agitation conditions (constant temperature of 28°C and 200 rpm, respectively) to determine growth under stress conditions.
2.8 Genotypic Characterization
Genotypic characterization of the 17 selected isolates was performed using BOX-PCR (repetitive sequence based-PCR), as previously reported by Versalovic et al. [20], with the primers BOX-AR1 (5′-CTACGGCAAGGCGACGCTGACG-3′). The fingerprints obtained were analyzed using the GelCompar II program (Applied Maths BV). The degree of similarity between them was determined using Pearson’s correlation coefficient. Dendrograms were constructed from the distance matrix using the unweighted pair group method with the arithmetic mean (UPGMA) algorithm.
2.9 Data Analysis and Evaluation of Diversity
Rhynchosia isolates were grouped according to the degree of similarity of their BOX-PCR banding patterns. A 90% cut-off value was considered. The Shannon et al. [21] index was applied to determine the diversity index (H) and estimated using Eq. (3):
where pi is the relative abundance of isolates of each OTU.
Data were analyzed using INFOSTAT software [22]. Variables were analyzed with ANOVA, and a comparison of means was performed using Fisher’s protected test (i.e., LSD), with a significance level of 0.05.
3.1 Edaphic Characterization of the Legume Sampling Area
Legume samples of the genus Rhynchosia were collected mainly from sandy loam-like soils (Table 2). Their main characteristic was the low amounts of nitrogen (N) and phosphorus (P) detected. Thus, alternative strategies to the use of agrochemicals could be developed to overcome the poor nutritional status of the soil. Moreover, the pH values detected in these soils showed a remarkable alkaline tendency, with calcium carbonate stones on the surface and in the hardened soil layer near the surface. These facts were further considered when establishing the conditions for cell culture at different pH levels.
3.2 Forage Capacity of Rhynchosia spp.
According to the results shown in Table 3, native Rhynchosia legumes could be well utilized for forage cultivation. It presented remarkably good biomass yields. Under cultivation, the biomass produced can be much higher than that observed (58–161 g/plant), as indicated by Porta Siota et al. [23].
Moreover, its nodulation capacity, determined by the symbiotic relationship established with rhizobacteria, could improve forage values through nitrogen fixation. Among the selected legume sampling areas, Rucanelo and Victorica showed the highest forage performances, as observed in the biomass values. Native legumes collected from Rucanelo had the best protein content.
Forage capacity results were similar to those obtained for standard legume crops, such as alfalfa (16%–20% protein and 58%–65% digestibility values) [24]. In some cases, lower DMD values were recorded for native Rhynchosia, probably due to the higher proportion of stems compared with leaves, which increases fiber values.
3.3 Nodule Examination and Isolation of Culturable Endophytic Bacteria
The root systems of all field-collected species bore nodules that varied in number and shape. Mature Rhynchosia nodules were small and elongated. They also showed indeterminate growth, consistent with the morphology described by other authors for Rhynchosia schimperi [25]. A total of 54 bacterial strains were isolated from inside the surface-sterilized nodules, from which liquid cultures were developed. Root nodule endophytic bacteria associated with legumes of the genus Rhynchosia have not been studied in detail.
3.4 Nodulation Ability of Bacterial Isolates
Garau et al. [26] described the nodules of several plants of the papilionoid legume Rhynchosia ferulifolia and reported the presence of species of the genus Burkholderia inside the nodules. The nodulation test performed in this study revealed that the strains isolated from nodules of Rhynchosia were unable to nodulate their original hosts effectively at the experiment times evaluated here. Subsequently, nodC genes were amplified to evaluate whether the isolates had the ability to nodulate. All isolates were negative for the amplification of this gene. In Fig. 1, the agarose gel is shown with the PCR product of five of the isolates. This indicates, therefore, that endophytic bacteria also establish a symbiotic relationship with the host plant as they exhibit growth-promoting properties. This result is consistent with other studies, in which no cultivable nodulating rhizobia was found [9]. The bacteria that cohabit in these Rhynchosia nodules belong to different taxonomic groups, which could be selected from some mechanism generated by the plant or by the rhizobium that initiates the nodule. This allows endophytic colonization based on the ability of the bacteria to cooperate in the difficult task of surviving in hostile environments such as the Pampean semiarid region [27].
Figure 1: Amplification of the nodC gene by colony-PCR. Lane 1: Positive control, Ensifer meliloti B399; lane 2: Isolation S54; lane 3: Isolation T22; lane 4: Isolation V45; lane 5: Isolation V48; lane 6: Isolation V50; lane 7: Negative control; lane 8: 100 pb molecular weight
3.5 PGPR Characteristics of the Isolated Bacteria
All evaluated isolates produced indole-3-acetic-like auxins, reaching a median value of 5.91 µg/ml (Table 4). It is important to note that these values were obtained without tryptophan supplementation in the culture media. Therefore, although the addition of this amino acid to the culture medium promotes IAA synthesis, it is unlikely to be present in large amounts in the soil.
The highest value (22.68 µg/ml) was observed for strain V77 (Table 4), which is considered a satisfactory result because the production of high amounts (more than 50 µg/ml) could be toxic for plants [28]. These values are in agreement with those reported for symbiotic rhizobial microorganisms [29]. Ahmad et al. [30] showed that Azotobacter and Pseudomonas isolates produce different plant hormones when they are grown in liquid media, with values between 5 and 20 µg/ml for the particular case of IAA production. In addition, it should be considered that the compounds secreted by the roots of the plant could regulate the production of IAA by bacteria in a different way [28].
Almost half (46.29%) of the evaluated strains produced siderophores. The color change from blue to clear yellow, as described in the traditional CAS assay, indicates the production of carboxylate-type siderophores. A representative example is shown in Fig. 2a. Siderophore production was estimated by measuring the solubilized area in the Petri dish (Table 4). Strain S54 exhibited the highest siderophore production.
Figure 2: Petri dish with CAS agar indicating siderophore production (a) and Petri dish with NBRIP medium and Petri dish indicating phosphate solubilization (b)
The ability to produce siderophores is considered an important trait to promote plant development under iron-limiting conditions. This ability would allow the strains to carry out biological control of plant pathogens as they would eliminate the available iron from the medium and deliver it to the plant [28].
3.5.3 Phosphate Solubilization
Only 14.81% of the selected strains tested for phosphate solubilization were positive for this assessment. The phosphate solubilization ability was determined based on the solubilized area on the Petri dish (Fig. 2b and Table 4). According to their solubilization abilities, two groups were observed: the best solubilizers (approximately 0.8–1 cm2 solubilization) were strains R10, T26, R4, and S37, while those showing the lowest solubilization ability were strains R1, T22, T27 and T31 (approximately 0.2–0.3 cm2). Considering that this phenomenon is often associated with a decrease in pH by microorganisms, it is promising that the growth of these strains was not affected by the acid pH stress condition evaluated (Fig. 3a).
Figure 3: Performance of isolates growing under pH stress conditions: GROUP 1 strain S68 (a), GROUP 2 strain T70 (b), GROUP 3 strain T66 (c), GROUP 4 strain V12 (d)
Phosphate solubilization has been considered an important trait that bacteria exhibit to promote plant growth since phosphate is often found as an insoluble salt, unavailable to the plant. Phosphate solubilization is usually associated with soil acidification, which dissolves the inorganic insoluble phosphate salts [28]. Phosphate solubilization ability has been reported for several rhizobacteria, such as Pseudomonas, Bacillus, Rhizobium, Burkholderia, Achromobacter, Agrobacterium, Microccocus, Aereobacter, Flavobacterium, Enterobacter, Pantotea, Klebsiella, Rhodobacter, and Serratia [28,31].
It is important to note that 57.41% of the isolates showed more than one PGPR activity. These results are consistent with those reported for both rhizobial and non-rhizobial bacteria regarding the common characteristic of root infection capacity in legumes [32,33]. Specifically, as shown in these results, the ability to solubilize phosphates and produce phytohormones has been reported for endophytic bacteria in legume nodules [34]. Hossain et al. [35] found non-rhizobial isolates in nodules of A. hypogaea L., D. lablab L., V. mungo L., and S. sesban L, which promoted the growth of the legumes studied. In addition to the plant growth-promoting characteristics studied in this work, some bacteria have additional properties that could support their application as bioinoculants in agriculture [1]. An example of this could be the ability to relieve plant stress through the production of ACC deaminase [36].
The use of these microorganisms for the formulation of bioinoculants could additionally contribute to the establishment of plants and the improvement of soil properties in degraded semiarid areas. This would help to ensure a sustainable increase in agricultural productivity in a future climate change scenario [5,37].
Studies indicate that the combined use of different microorganisms allows not only better growth but also effective protection of plants [38]. The microorganisms isolated in this study could be used in multicomponent bioformulations. Combining bacterial strains in a single formulation would improve efficacy and reliability, which appears to be very promising [39].
3.6 Performance of Isolates Growing under Stress Conditions: pH and Osmolarity
Based on the results of the stress conditions, isolates were grouped according to their growth kinetics behavior under the pH and osmolarity levels tested. Four groups were defined for pH stress, as follows:
GROUP 1: Isolates were not affected under the pH stress conditions evaluated: R1, R2, R3, R4, R5, R7, R8, R9, R10, T22, T25, T26, T27, T31, T35, T63, T77, S40, S54, S56, S58, S59, S68, S78, V11, V45, V48, V50, V51, V52, V62, V73, V74, V75, V76 and V77 (Fig. 3a).
GROUP 2: Little or no cell growth at pH 9: T28, T32, T34, T36, T70, S37, S41, S43, V16 and V61 (Fig. 3b).
GROUP 3: Isolates developed a “lag” stage of adaptation to pH 9 after which the levels of cellular concentration of the other conditions were equated: T66, T67, S55, and V60 (Fig. 3c).
GROUP 4: Isolate growth kinetics and/or cell concentration was negatively affected by alkaline pH levels: R6, T65, S39 and V12 (Fig. 3d).
Regarding osmolarity stress conditions, isolates were grouped as follows:
GROUP 1: Isolates were not affected by the different osmotic conditions: R1, R2, R3, R4, R5, R7, R8, R9, R10, T22, T26, T27, T28, T31, T32, T35, T63, T66, T67, T70, S37, S39, S40, S41, S43, S54, S55, S58, S59, S68, S78, V11, V12, V45, V48, V50, V52, V60, V73, V74, V75, V76 and V77 (Fig. 4a).
Figure 4: Performance of isolates growing under osmotic stress: GROUP 1 strain T32 (a), GROUP 2 strain T77 (b), GROUP 3 strain V16 (c) and GROUP 4 strain S56 (d)
GROUP 2: Isolates showed little or no cell growth in low and high osmolarity conditions (0 and 200 mM NaCl, respectively): T25 and T77 (Fig. 4b).
GROUP 3: Isolates developed a “lag” stage of adaptation with conditions of high osmolarity after which the cell concentration levels of the other conditions were equated: T36, T65, V16, V51, V61 and V62 (Fig. 4c).
GROUP 4: Isolates in which an increase in osmolarity favored cell growth: R6 and S56 (Fig. 4d).
To represent the behavior of each strain in relation with its response to the stress conditions, an index was calculated by considering the relationship between the specific growth rate (µ) obtained in each treatment and that observed in the control experiment. Analyzing these relationships (Table 5), we found that the isolates whose relationship values were 1 ± 0.05 overcame the corresponding stress condition and reached the same levels of cell concentration as the control treatment.
Of the 54 isolates subjected to different stress conditions, 57.41% were not affected concerning the cell concentration reached and growth kinetics (highlighted in black and marked with * in Table 5). The high tolerance of the strains to these conditions could be attributed to the fact that they were isolated from soils with pH values tending to alkalinity (Table 2). Based on these values, it could be inferred that they were saline soils since they exhibited pH values between approximately 7.5 and 8. Choudhury et al. [40] found variability in the behavior of microorganisms when they were exposed to variations in osmolarity and pH. In agreement with our results, Karagöz et al. [41] found isolates with plant growth-promoting abilities (N2 fixers, phosphate solubilizers, and siderophore producers) that were tolerant to high concentrations of NaCl in acidic and alkaline rhizospheric soils. The multiplicity and redundancy of homeostatic mechanisms are tags of stress conditions in bacteria [42].
The results show promise for restoring native vegetation on stressed soils and enhancing restoration benefits through plant-microorganism interactions. Öğütçü et al. [43] found that salinity adversely affected chickpea growth parameters, but inoculation with native strains improved its ability to thrive under saline conditions. Therefore, understanding native microbial flora and its adaptability is crucial for optimizing this association. Field experiments with plant growth-promoting rhizobacteria from stressed areas should be conducted [44].
Ventorino et al. [45] found that the reference strains used were less effective and competitive than the natural population and that no native isolate belonged to the reference strain. Nautiyal et al. [46] reported that phosphate solubilization activity decreased gradually with increasing concentration of NaCl, which emphasizes the importance of working with species that tolerate these conditions. Sachdev et al. [47] reported that the production of IAA by native strains under salt stress conditions correlated positively with greater root growth, thus contributing significantly to improving the plant tolerance to salt. Lebrazi et al. [48] also highlighted that IAA-producing rhizobacteria could be harnessed to improve plant growth.
3.7 Genetic Diversity of Isolates
To carry out genetic characterization analysis, 17 microorganisms were selected based on their ability to tolerate the studied stress conditions and considering whether they exhibited two or more PGPR properties. The dendrogram based on BOX fingerprint patterns (Fig. 5) showed that the genetic diversity of isolates occupying Rhynchosia nodules was high (H = 2.08) [3] although the selected isolates shared certain characteristics. Considering a similarity coefficient of 90%, the BOX patterns could be grouped into nine different operational taxonomic units (OTUs). Only 42 out of 17 isolates had the same genetic profile (V73 and V74). These results confirm the expected high level of diversity.
Figure 5: Similarity dendrogram of selected bacterial isolates of Rhynchosia based not only on the ability to tolerate the studied stress conditions but also on their PGPR properties, considering two or more growth-promoting activities
Based on these results, future trials are planned to select isolates that can be used in the formulation of biofertilizers, through inoculation tests of Rhynchosia plants. In this way, the ability to promote plant growth will be evaluated in vivo, by subjecting the plants to the different stress conditions studied in this work. This will allow a strict selection of strains suitable for biotechnological purposes, on which analyses will be carried out for identification, based on the 16S gene. Although these genomic approaches are of great taxonomic importance, the genes identified remain to be phenotypically characterized [1]. Other authors have characterized and grouped isolates from Rhynchosia nodules in the genus Burkholderia [49].
None of the isolates studied had the nodC gene in their genome. Therefore, we conclude that in Rhynchosia plant nodules, different endophytic microorganisms cohabit with the rhizobium that gave rise to the formation of this structure. Moreover, the rhizobacteria that participated in the formation of the nodules may have reached such a degree of bacteroid differentiation that it prevented the dedifferentiation to the state of vegetative cultivable cells, and therefore, they could not be isolated. The finding of endophytic strains with plant growth-promoting properties, including tolerance to stress conditions, is of great importance in promoting crop development in semiarid areas. The results obtained contribute to improving our knowledge of the benefits of using PGPR bacteria in the growth and development of native legumes with forage potential in arid and semiarid areas and to ensuring their implantation through sustainable agriculture. Research groups are engaged in an ongoing international effort to isolate and characterize plant growth-promoting microorganisms from native hosts, to identify elite microorganisms capable of competing in the soil, surviving harsh environmental conditions, and promoting plant growth, with the ultimate aim of using them in the formulation of bioinoculants.
Acknowledgement: This research was supported by Facultad de Ciencias Exactas y Naturales de la Universidad Nacional de La Pampa (FCEyN-UNLPam).
Funding Statement: The authors received no specific funding for this study.
Author Contributions: The authors confirm their contribution to the paper as follows: Lucero CT and Lorda GS designed the research study. Lucero CT and Ruiz MA conducted field sampling. Lucero CT, Castaño C, and Pagliero F conducted laboratory/greenhouse experiments. Lucero CT, Ambrosino ML, and Lorda GS analyzed data and wrote the manuscript. All authors reviewed the results and approved the final version of the manuscript.
Availability of Data and Materials: The datasets generated during and/or analyzed during the current study are available from the corresponding author upon reasonable request.
Ethics Approval: Not applicable.
Conflicts of Interest: The authors declare that they have no conflicts of interest to report regarding the present study.
References
1. Bellés-Sancho P, Beukes C, James EK, Pessi G. Nitrogen-fixing symbiotic Paraburkholderia species: current knowledge and future perspectives. Nitrogen. 2023;4(1):135–58. doi:10.3390/nitrogen4010010. [Google Scholar] [CrossRef]
2. Wei GH, Zhang ZX, Chen C, Chen WM, Ju WT. Phenotypic and genetic diversity of rhizobia isolated from nodules of the legume genera Astragalus, Lespedeza, and Hedysarum in Northwestern China. Microbiol Res. 2008;163:651–62. doi:10.1016/j.micres.2006.09.005. [Google Scholar] [PubMed] [CrossRef]
3. Alotaibi MM, Aljuaid A, Alsudays IM, Aloufi AS, AlBalawi AN, Alasmari A, et al. Effect of bio-fertilizer application on agronomic traits, yield, and nutrient uptake of barley (Hordeum vulgare) in saline soil. Plants. 2024;13(7):951. doi:10.3390/plants13070951. [Google Scholar] [PubMed] [CrossRef]
4. Ayangbenro AS, Babalola OO. Reclamation of arid and semi-arid soils: the role of plant growth-promoting archaea and bacteria. Curr Plant Biol. 2021;25:100173. doi:10.1016/j.cpb.2020.100173. [Google Scholar] [CrossRef]
5. Bonatelli ML, Lacerda-Júnior GV, dos Reis Junior FB, Fernandes-Júnior PI, Melo IS, Quecine MC. Beneficial plant-associated microorganisms from semiarid regions and seasonally dry environments: a review. Front Microbiol. 2021;11:553223. doi:10.3389/fmicb.2020.553223. [Google Scholar] [PubMed] [CrossRef]
6. Moir J, Jordan P, Moot D, Lucas D. Phosphorus response and optimum pH ranges of twelve pasture legumes grown in an acid upland New Zealand soil under glasshouse conditions. J Soil Sci Plant Nutr. 2016;16:438–60. doi:10.4067/S0718-95162016005000038. [Google Scholar] [CrossRef]
7. Turner BL. Systematics of the Rhynchosia senna complex (Fabaceae). Lundellia. 2011;14(1):27–31. doi:10.25224/1097-993X-14.1.27. [Google Scholar] [CrossRef]
8. Jia X, Zhang C, Qiu JF, Wang L, Bao J, Wang K, et al. Purification, structural characterization and anticancer activity of the novel polysaccharides from Rhynchosia minima root. Carbohydr Polym. 2015;132:67–71. doi:10.1016/j.carbpol.2015.05.059. [Google Scholar] [PubMed] [CrossRef]
9. Benhizia Y, Benhizia H, Benguedouar A, Muresu R, Giacomini A, Squartini A. Gamma proteobacteria can nodulate legumes of the genus hedysarum. Syst Appl Microbiol. 2004;27(4):462–8. doi:10.1078/0723202041438527. [Google Scholar] [PubMed] [CrossRef]
10. Gee GW, Bauder JW. Particle-size analysis. In: Klute A, editor. Methods of soil analysis, Part 1, physical and mineralogical methods. Madison, Wisconsin: American Society of Agronomy; 1986. p. 383–411. [Google Scholar]
11. Association of Official Analytical Chemists, Helrich, Kenneth. Official methods of analysis. 15th ed. Arlington, VA: Association of Official Analytical Chemists; 1990. [Google Scholar]
12. Bray RH, Kurtz LT. Determination of total, organic and available forms of phosphorus in soil. Soil Sci. 1945;59:39–45. doi:10.1097/00010694-194501000-00006. [Google Scholar] [CrossRef]
13. Goering HK, Van Soest PJ. Forage fiber analyses (apparatus, reagents, procedures, and some applications). Washington DC, USA: Agricultural Research Service; 1970. [Google Scholar]
14. Sirio AA, Ávalos Llano KR. Capítulo3: aislamiento, identificación y conservación de rizobios. In: Castelan M, Hack C, Porta M, Sotelo C, coord. Metodologías microbiológicas de indicadores ambientales de suelo. Corrientes: EUDENE; 2022. p. 38–56 (In Spanish). [Google Scholar]
15. Sarita S, Sharma PK, Priefer UB, Prell J. Direct amplification of rhizobial nodC sequences from soil total DNA and comparison to nodC diversity of root nodule isolates. FEMS Microbiol Ecol. 2005;54(1):1–11. doi:10.1016/j.femsec.2005.02.015. [Google Scholar] [PubMed] [CrossRef]
16. Costacurta A, Mazzafera Paulo, Rosato YB. Indole-3-acetic acid biosynthesis by Xanthomonas axonopodisp v. citri is increased in the presence of plant leaf extracts. FEMS Microbiol Lett. 1998;159(2):215–20. [Google Scholar]
17. Schwyn B, Neilands JB. Universal chemical assay for the detection and determination of siderophores. Anal Biochem. 1987;160(1):47–56. doi:10.1016/0003-2697(87)90612-9. [Google Scholar] [PubMed] [CrossRef]
18. Katznelson H, Bose B. Metabolic activity and phosphate-dissolving capability of bacterial isolates from wheat roots, rhizosphere, and non-rhizosphere soil. Can J Microbiol. 1959;5(1):79–85. doi:10.1139/m59-010. [Google Scholar] [PubMed] [CrossRef]
19. Abdelmoumen H, Filali-Maltouf A, Neyra M, Belabed A, El Idrissi MM. Effect of high salts concentrations on the growth of rhizobia and responses to added osmotica. J Appl Microbiol. 1999;86(6):889–98. doi:10.1046/j.1365-2672.1999.00727.x. [Google Scholar] [CrossRef]
20. Versalovic J, Schneider M, de Bruijn FJ, Lupski JR. Genomic fingerprinting of bacteria using repetitive sequence-based polymerase chain reaction. Methods Mol Cell Biol. 1994;5(1):25–40. [Google Scholar]
21. Shannon CE, Weaver W. The mathematical theory of communication. Urbana: University Of Illinois Press; 1949. [Google Scholar]
22. Di Rienzo JA, Casanoves F, Balzarini MG, González L, Tablada M, Robledo CW. Infostat-Statistical software. 2018. Available from: http://www.infostat.com.ar. [Accessed 2024]. [Google Scholar]
23. Porta Siota F, Ledesma G, Petruzzi HJ, Morici EFA. Acumulación de biomasa aérea en poblaciones de Rhynchosia senna Gillies ex Hook. en dos temporadas de crecimiento. In: VIII Congreso Nacional y IV Congreso del Mercosur sobre Manejo de Pastizales Naturales; 2018; Catamarca, Argentina, Ediciones. p. 48–9 (In Spanish). [Google Scholar]
24. Blain GL, Berone GD. Digestibilidad de la biomasa aérea de cultivares de alfalfa ante distintas alturas de corte. In: 41a Congreso Argentino de Producción Anima; 2018; Mar del Plata, Buenos Aires. (In Spanish). [Google Scholar]
25. Panwar D. Nodulation in Rhynchosia schimperi: a rare legume of indian thar desert. Int J Current Sci Res Rev. 2023;6(5):2790–7. [Google Scholar]
26. Garau G, Yates RJ, Deiana P, Howieson JG. Novel strains of nodulating Burkholderia have a role in nitrogen fixation with papilionoid herbaceous legumes adapted to acid, infertile soils. Soil Biol Biochem. 2009;41(1):125–34. doi:10.1016/j.soilbio.2008.10.011. [Google Scholar] [CrossRef]
27. Zakhia F, Jeder H, Willems A, Gillis M, Dreyfus B, de Lajudie P. Diverse bacteria associated with root nodules of spontaneous legumes in tunisia and first report for nifH-like gene within the genera Microbacterium and Starkeya. Microb Ecol. 2006;51(3):375–93. doi:10.1007/s00248-006-9025-0. [Google Scholar] [PubMed] [CrossRef]
28. Sánchez-Cruz R, Tpia Vázquez I, Batista-García RA, Méndez-Santiago EW, del Sánchez-Carbente MR, Leija A, et al. Isolation and characterization of endophytes from nodules of Mimosa pudica with biotechnological potential. Microbiol Res. 2019;218:76–86. doi:10.1016/j.micres.2018.09.008. [Google Scholar] [PubMed] [CrossRef]
29. Khalid A, Arshad M, Zahir ZA. Screening plant growth-promoting rhizobacteria for improving growth and yield of wheat. J Appl Microbiol. 2004;96(3):473–80. doi:10.1046/j.1365-2672.2003.02161.x. [Google Scholar] [PubMed] [CrossRef]
30. Ahmad F, Ahmad I, Khan MS. Screening of free-living rhizospheric bacteria for their multiple plant growth promoting activities. Microbiol Res. 2008;163(2):173–81. doi:10.1016/j.micres.2006.04.001. [Google Scholar] [PubMed] [CrossRef]
31. Khan MS, Zaidi A, Ahemad M, Oves M, Wani PA. Plant growth promotion by phosphate solubilizing fungi–current perspective. Arch Agron Soil Sci. 2010;56(1):73–98. doi:10.1080/03650340902806469. [Google Scholar] [CrossRef]
32. Gerding M, Oyarzúa P, García L, Fischer S, Norambuena C, Barahona V, et al. Diversity and symbiotic effectiveness of Adesmia spp. root nodule bacteria in Central and Southern Chile. Symbiosis. 2016;72(1):61–72. [Google Scholar]
33. Velázquez E, Martínez-Hidalgo P, Carro L, Alonso P, Trujillo ME, Martínez-Molina E. Nodular endophytes: an untapped diversity. Benef Plant-Microbial Interactions: Ecol Appl. 2013;215–35. [Google Scholar]
34. Kumar V, Pathak DV, Dudeja SS, Saini R, Giri R, Narula S, et al. Legume nodule endophytes more diverse than endophytes from roots of legumes or non legumes in soils of Haryana, India. J Microbiol Biotechnol Res. 2013;3(3):83–92. [Google Scholar]
35. Hossain MZ, Lundquist P. Nodule inhabiting non-rhizobial bacteria and their influence on growth of selected leguminous plants of Bangladesh. Biores Commun-(BRC). 2016;2(1):134–8. [Google Scholar]
36. Zafar-ul-Hye M, Shahzad Bhutta T, Shaaban M, Hussain S, Farooq Qayyum M, Aslam U, et al. Influence of plant growth promoting rhizobacterial inoculation on wheat productivity under soil salinity stress. Phyton-Int J Exp Bot. 2019;88(2):119–29. doi:10.32604/phyton.2019.06570. [Google Scholar] [CrossRef]
37. Chandran H, Meena M, Swapnil P. Plant growth-promoting rhizobacteria as a green alternative for sustainable agriculture. Sustainability. 2021;13:10986. doi:10.3390/su131910986. [Google Scholar] [CrossRef]
38. Kour D, Khan SS, Kaur T, Kour H, Singh G, Yadav A, et al. Drought adaptive microbes as bioinoculants for the horticultural crops. Heliyon. 2022;8(5):e09493. doi:10.1016/j.heliyon.2022.e09493. [Google Scholar] [PubMed] [CrossRef]
39. Mesquita A, Bandeira LL, Cavalcante FG, Ribeiro GAL, Martins SCS, Martins CM. In vitro co-inoculation of rhizobacteria from the semi-arid aiming at their implementation as bio-inoculants. Revista Brasileira De Ciências Ambientais (RBCIAMB). 2023;58(1):59–66. doi:10.5327/Z2176-94781481. [Google Scholar] [CrossRef]
40. Choudhury B, Azad P, Kalita MC. Variability in symbiotic effectiveness of native rhizobia in acid stress. Curr Microbiol. 2010;61(2):85–91. doi:10.1007/s00284-009-9579-4. [Google Scholar] [PubMed] [CrossRef]
41. Karagöz K, Ateş F, Karagöz H, Kotan R, Çakmakçı R. Characterization of plant growth-promoting traits of bacteria isolated from the rhizosphere of grapevine grown in alkaline and acidic soils. Eur J Soil Biol. 2012;50:144–50. doi:10.1016/j.ejsobi.2012.01.007. [Google Scholar] [CrossRef]
42. Ayuso-Calles M, Flores-Félix JD, Rivas R. Overview of the role of rhizobacteria in plant salt stress tolerance. Agronomy. 2021;11:1759. doi:10.3390/agronomy11091759. [Google Scholar] [CrossRef]
43. Öğütçü H, Kasimoğlu C, Elkoca E. Effects of rhizobium strains isolated from wild chickpeas on the growth and symbiotic performance of chickpeas (Cicer arietinum L.) under salt stress. Turk J Agric For. 2010;34(5):361–71. [Google Scholar]
44. Adeleke RA, Nunthkumar B, Roopnarain A, Obi L. Applications of plant-microbe interactions in agro-ecosystem. In: Kumar V, Prasad R, Kumar M, Choudhary DK, editors. Microbiome in plant health and disease. Singapore: Springer Nature; 2019. p. 1–34. [Google Scholar]
45. Ventorino V, Caputo R, De Pascale S, Fagnano M, Pepe O, Moschetti G. Response to salinity stress of Rhizobium leguminosarum bv. viciae strains in the presence of different legume host plants. Annals Microbiol. 2011;62(2):811–23. [Google Scholar]
46. Nautiyal CS, Bhadauria S, Kumar P, Lal H, Mondal R, Verma D. Stress induced phosphate solubilization in bacteria isolated from alkaline soils. FEMS Microbiol Lett. 2000;182(2):291–6. doi:10.1111/fml.2000.182.issue-2. [Google Scholar] [CrossRef]
47. Sachdev D, Chaudhari HG, Kasture VM, Dhavale DD, Chopade BA. Isolation and characterization of indole acetic acid (IAA) producing Klebsiella pneumoniae strains from rhizosphere of wheat (Triticum aestivum) and their effect on plant growth. Indian J Exp Biol. 2009;47(12):993–1000. [Google Scholar] [PubMed]
48. Lebrazi S, Fadil M, Chraibi M, Fikri-Benbrahim K. Screening and optimization of indole-3-acetic acid production by Rhizobium sp. strain using response surface methodology. J Genetic Eng Biotechnol. 2020;18(1):21. doi:10.1186/s43141-020-00035-9. [Google Scholar] [PubMed] [CrossRef]
49. De Meyer SE, Cnockaert M, Ardley JK, Trengove RD, Garau G, Howieson G, et al. Burkholderia rhynchosiae sp. nov, isolated from Rhynchosia ferulifolia root nodules. Int J Syst Evol Microbiol. 2013;63:3944–9. doi:10.1099/ijs.0.048751-0. [Google Scholar] [PubMed] [CrossRef]
Cite This Article
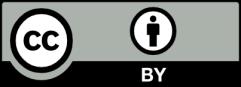
This work is licensed under a Creative Commons Attribution 4.0 International License , which permits unrestricted use, distribution, and reproduction in any medium, provided the original work is properly cited.