Open Access
REVIEW
Research Progress on Plant Anti-Freeze Proteins
College of Landscape and Tourism, Hebei Agricultural University, Baoding, 071000, China
* Corresponding Authors: Yinran Huang. Email: ; Ping Li. Email:
(This article belongs to the Special Issue: Abiotic Stress Impacts on Plant Physiology and Their Alleviation)
Phyton-International Journal of Experimental Botany 2024, 93(6), 1263-1274. https://doi.org/10.32604/phyton.2024.050755
Received 16 February 2024; Accepted 13 May 2024; Issue published 27 June 2024
Abstract
Plant antifreeze proteins (AFPs) are special proteins that can protect plant cells from ice crystal damage in low-temperature environments, and they play a crucial role in the process of plants adapting to cold environments. Proteins with these characteristics have been found in fish living in cold regions, as well as many plants and insects. Although research on plant AFPs started relatively late, their application prospects are broad, leading to the attention of many researchers to the isolation, cloning, and genetic improvement of plant AFP genes. Studies have found that the distribution of AFPs in different species seems to be the result of independent evolutionary events. Unlike the AFPs found in fish and insects, plant AFPs have multiple hydrophilic ice-binding domains, and their recrystallization inhibition activity is about 10–100 times that of fish and insect AFPs. Although different plant AFPs have the characteristics of low TH and high RI, their DNA and amino acid sequences are completely different, with small homology. With in-depth research and analysis of the characteristics and mechanisms of plant AFPs, not only has our understanding of plant antifreeze mechanisms been enriched, but it can also be used to improve crop varieties and enhance their freezing tolerance, yield, and quality through genetic engineering. In addition, the study of plant AFPs also contributes to our understanding of freezing resistance mechanisms in other organisms and provides new research directions for the field of biotechnology. Therefore, based on the analysis of relevant literature, this article will delve into the concepts, characteristics, research methods, and mechanisms of plant AFPs, summarize the latest research progress and application prospects of AFPs in plant, and provide prospects for the future development of AFP gene research.Keywords
Low temperature is an abiotic stress factor that plants face, which not only harms their growth and development but also restricts their geographical distribution and crop yield. In recent years, with global climate change and the frequent occurrence of extreme weather events, low-temperature stress has become a global natural disaster, posing a threat to crop growth and survival, causing significant losses in agricultural production, and presenting arduous consequences for the introduction and planting of plants in landscaping. Therefore, unraveling the cold tolerance mechanism is of acute significance in crop improvement via traditional plant breeding/genetic engineering approaches to confer cold resilience in susceptible genotypes. Low-temperature stress occurs when the environmental temperature remains consistently below the optimal temperature for plant growth, including chilling (0°C–15°C) and freezing stress (<0°C) [1]. Chilling stress refers to the threat posed by temperatures above zero degrees to plants, where ice does not form inside the cells, but it can cause membrane rigidification, destabilize protein complexes, and impair photosynthesis. Freezing stress refers to the damage or even death of cells caused by temperatures below zero degrees. Both types of stress have adverse effects on plant growth and development [2]. Different species have different tolerances to low temperatures. For cold-sensitive plants, even a slight temperature decrease can cause severe damage, and some may experience a reduction in yield of more than 50%. Low temperatures not only severely damage plant growth but can also lead to plant death, resulting in huge losses in production. Therefore, plants have developed various mechanisms to cope with low-temperature stress through morphological, physiological, biochemical, and molecular processes, including gene expression, enzyme activity, and metabolic homeostasis. To withstand the stress of cold, temperate plants have developed an adaptive response called cold acclimation, where their freezing tolerance is enhanced after exposure to lower non-freezing temperatures. Different plants also exhibit varying levels of freezing tolerance. To protect cells from the formation of ice within and between cells under freezing temperatures, plants produce various compounds, including plant AFPs.
AFPs were initially discovered as specific proteins in the serum of marine fish from the Antarctic and Arctic regions that can bind to ice crystals. They prevent the formation of ice nuclei in body fluids, enabling fish to adapt to low-temperature conditions [3]. Plant AFPs were discovered for the first time in winter rye, and research results indicate that AFPs extracted from rye leaves can alter the shape of ice crystals, similar to AFPs found in fish and insects. Currently, more than 60 different plant species have been found to possess AFPs, including monocotyledonous plants, dicotyledonous plants, and gymnosperms. AFP binds to small ice crystals, inhibiting their growth and recrystallization. By enhancing freeze tolerance and frost resistance, AFPs contribute to increasing species diversity in some of the most harsh and barren environments [4].
AFPs bind to ice crystals and alter their growth. These proteins exhibit significant diversity in their structures [5]. Plant AFPs also possess low thermal hysteresis (TH) and high recrystallization inhibition (RI) properties. The study of AFP proteins can be applied in areas such as cryopreservation and plant breeding, enhancing agricultural productivity and resilience to risks. This makes it highly valuable for research purposes. This article will provide a comprehensive review of the characteristics, structures, functions, and applications of plant AFPs. The article will also explore the regulatory mechanisms of plant AFPs, highlight the latest advancements in research, and discuss their applications across various fields. Furthermore, it will provide insights into the future directions of plant AFP research and development.
2 Molecular Characteristics of Plant Antifreeze Proteins
Unlike AFPs found in animals, plant AFPs do not function as cryoprotectants in cold adaptation. Instead, they serve as a part of the protein-ice interaction in plants. In general, plant AFPs have a lower TH temperature of approximately 0.1°C–0.5°C and a higher ice recrystallization inhibition (IRI) effect compared to fish, insect, and bacterial-type AFPs, which typically range from 2°C–13°C. However, there are also reports suggesting that plant AFPs can exhibit higher TH. (Dicentra cucularia; Daucus carota; Hemerocallis fulva; Populus deltoides; Quercus alba; and Triticum aestivum). AFP molecules with low TH and high IRI seem to possess evolutionary adaptability in plants. The low TH allows for more controlled growth of ice crystals, while the high RI enables AFPs to function even at very low concentrations [6].
Furthermore, AFPs are dual-function proteins in cells, as some AFPs are homologous to pathogenesis-related proteins and possess both antifreeze and hydrolytic activities [7]. Jasmonic acid (JA) and ethylene play important roles in regulating plant freeze tolerance. When plants are subjected to non-freezing stress conditions, the application of ethylene and jasmonic acid can still lead to the accumulation of antifreeze active substances (Fig. 1). Some AFPs have dual activities, both antifreeze and antimicrobial properties, which are related to their homology with certain pathogenesis-related proteins. Heterologous expression of AFP genes in freeze-sensitive plants can enhance the freezing tolerance of the host plants [8].
Figure 1: Mechanism of production of plant AFPs: These three pathways regulate gene expression so that AFPs accumulate and transport them to the apoplast
Research has revealed that the specificity of amino acid sequences, double-helical structures, and ice-binding units of AFPs in plants correlates with the observed freezing tolerance in plants. Cold acclimation of winter rye (Lolium perenne) leaves led to the isolation and purification of AFPs from the extracellular matrix, making them the earliest discovered plant-derived AFPs. After isolation and purification, these AFPs exhibited the ability to modify ice crystal growth. Sugars and AFPs isolated from wintergreen (Ammopiptanthus mongolicus) leaves are the primary substances involved in the freezing tolerance physiological process of winter plants. Wintergreen AFPs exhibit thermal hysteresis activity, allowing them to regulate ice crystal growth [9,10]. Obtaining AFPs from carrot (Daucus carota) roots and introducing their cDNA into tobacco (Nicotiana tabacum) allows for the cloning of genes that encode plant-derived AFPs and opens up avenues for studying these genes in the plant genome. This research holds great significance in the field of cloning genes involved in plant freezing resistance, contributing to a better understanding of plant anti-freezing mechanisms.
3 Mechanisms of Antifreeze Proteins in Regulating Plant Freezing Tolerance
Different plants may have different forms of AFPs, but they all share functional mechanisms such as thermal hysteresis activity (THA), modification of ice crystal growth morphology, and RI effects. They can regulate the state of the cytoplasmic solution and inhibit the growth activity of ice crystals in the solution. They achieve this by hindering the pathways of ice crystal growth, thus inhibiting the process of recrystallization. Different AFPs exhibit different TH values at the same concentration.
In general, the freezing point of a solution (such as NaCl, sucrose solution, etc.) is the temperature at which the vapor pressures of the solid and liquid phases are in equilibrium. Therefore, the freezing point should be equal to the melting point. Because AFPs primarily affect the process of ice formation and have little effect on the melting process, they lower the freezing point below the melting point. The difference between the freezing point and melting point is known as TH, Therefore, in the presence of AFPs, the freezing point of cellular fluid is further lowered, thereby avoiding the formation of ice crystals to some extent. This enhances the plant’s ability to withstand cold temperatures and improves its cold tolerance. AFPs are approximately 500 times more effective than any other known solute molecules in lowering the freezing temperature [11,12]. The property of AFPs is known as the thermal hysteresis effect.
3.2 The Effect of Modifying Ice Crystal Growth Morphology
In low-temperature environments, ice crystals change their growth morphology due to the influence of AFPs. They transition from growing as flattened elliptical shapes into hexagonal prisms. As the concentration of AFPs increases and the duration of their action lengthens, the morphology of ice crystals tends to become needle-like.
3.3 The Effect of Inhibiting Recrystallization
At the freezing point of a solution, small ice crystals in the solution gradually disappear and aggregate into larger ice crystals, causing damage to the structure of plants’ tissues. The addition of AFPs can inhibit the recrystallization phenomenon of ice crystals in the solution and promote uniform distribution of small ice crystals. The IRI effect of AFPs can protect cells from mechanical rupture and death. At very low concentrations, typically below 0.1 μg/mL, AFPs can inhibit ice recrystallization, resulting in smaller and more uniform ice crystal sizes. This helps to reduce damage to plants [13]. Additionally, in terms of protecting organisms from damage, the IRI effect may be more important than the TH effect, especially in freeze-tolerant plants and insects. Inhibiting ice recrystallization is somewhat easier than inhibiting ice crystal growth, and it requires lower concentrations of AFPs.
4 Identification and Expression Pattern Analysis of Plant Antifreeze Proteins
Plant antifreeze gene engineering primarily focuses on the extraction, purification, and identification aspects. For example, it involves the introduction of regulatory protein genes and studying their effects on plant growth and antifreeze activity. Most plant AFPs are distributed in the bark of woody plants, root tissues of herbaceous plants, and leaves. Traditional methods such as grinding and stirring are commonly used for extracting AFPs from bark, and root tissues. However, a permeation centrifugation method is typically employed for extracting AFPs from leaves. After extraction, AFPs can be purified using conventional techniques such as ultrafiltration (UF), column chromatography, ion exchange, ammonium salt precipitation, as well as classical methods like ice-affinity chromatography. Additionally, mass spectrometry can be employed for identification purposes. Recently, several AFPs have been isolated and characterized, and five full-length AFP cDNAs have been cloned in higher plants [14]. The antifreeze activity of AFPs is typically assessed using methods such as nanoliter osmometry, differential scanning calorimetry (DSC), sucrose sandwich plate assay, and capillary assay to evaluate their impact on ice crystal growth [15]. In a recent study, a colorimetric assay based on the color change of a solution containing freeze-unstable gold nanoparticles (AuNPs) was developed to investigate the characteristics of AFPs. This method utilizes the interaction between AFPs and AuNPs to modulate the color of the solution.
To predict and analyze AFPs based on different principles, experts have developed various computer tools such as AFPredictor, AFP-Pred, TargetFreeze, iAFP-Ense, afpCOOL, AFP-CMBPred, and AFP-LSE [14]. For instance, the substantial differences in the sequence and structure of AFPs pose challenges for researchers in their identification. afpCOOL, as a tool, enables rapid computer-based detection of AFPs. Users only need to provide the relevant protein sequence in FASTA format and Position-Specific Scoring Matrix (PSSM) to utilize afpCOOL [16]. The ability to accurately record the temperature at which ice nucleation occurs is crucial for studying biological ice nucleation. Recently, a novel computational method called AFP-LXGB has been proposed. This method utilizes the composition of dipeptides (DPC), grouped amino acid composition (GAAC), segmented position-specific scoring matrix-autocorrelation transform (Sg-PSSM-ACT), and pseudo-position-specific scoring matrix three-slice (PseTS-PSSM) to predict AFPs. Compared to the state-of-the-art methods, AFP-LXGB has shown an improvement in accuracy ranging from 3.70% to 4.09%. These results confirm the accuracy of AFP-LXGB in predicting AFP gene expression and regulatory mechanisms [17].
While the discovery of plant AFPs occurred around the same time as insect AFPs, the low TH activity of plant AFPs has not received sufficient attention from researchers. As a result, research on their structure and ice-binding sites has almost stagnated. The discovery of the carrot (Daucus carota) antifreeze protein (DcAFP) has brought hope to researchers. DcAFP exhibits a TH activity that is not very low and also possesses strong RI activity. Researchers have attempted to conduct in-depth studies on plant AFPs using DcAFP as a starting point. However, the relatively large molecular weight of DcAFP has posed limitations on the progress of these studies. With the discovery of another plant AFP, Lolium perenne antifreeze protein (LpAFP), research on plant AFPs has regained momentum (Table 1).
LpAFP exhibits similar characteristics of low TH activity and high RI activity. Moreover, it has a relatively small molecular weight, consisting of only 118 amino acid residues. This is comparable in size to fish AFPs and insect AFPs, making it suitable for studying its structure and ice-binding sites. The theoretical three-dimensional structure of LpAFP is a highly regular right-handed β-helix composed of circular repeats. Each circular repeat on the helix contains 14 to 15 amino acid residues. The interior of the helix consists of a hydrophobic core composed of conserved Val residues and a bend formed by conserved Asn residues. The “double-sided adhesive mode” was first proposed to explain the high RI activity of LpAFP. LpAFP possesses two ice-binding sites located on opposite sides of the β-helix structure. This allows LpAFP to simultaneously bind to two adjacent ice crystals, thereby inhibiting the growth of both crystals and ultimately preventing recrystallization from occurring. LpAFP functions like a double-sided adhesive, simultaneously binding to two ice crystals and preventing their growth. Since the discovery of LpAFP, a series of studies have been conducted on it. The coding gene of DcAFP was cloned from a cultivated carrot species in China [18,19]. Compared to the sequence published in GenBank, there were four nucleotide differences, but only one amino acid difference. The in vitro expression product exhibited high THA. The predicted theoretical three-dimensional structure of DcAFP consists of a highly regular right-handed β-helix. Each β-loop of the helix is composed of 24 amino acid residues from the leucine-rich repeat (LRR) motif, with two β-folds and a short 310-helix. This arrangement of secondary structures, known as “fold-fold-helix,” is consistent with the overall structure of DcAFP [19].
5 Molecular Mechanisms of Plant Antifreeze Proteins
Different AFPs act in different ways. AFPs inhibit the formation of ice crystals by binding to the water-ice interface. There are roughly three common mechanisms of action of AFPs: mechanism of rigid-body energetics, mechanism of ice crystal adsorption inhibition, and mechanism of thermal hysteresis kinetics. In the mechanism of rigid-body energetics, AFPs can fill the pathways of ice crystal growth, causing them to stop growing. In the mechanism of ice crystal adsorption inhibition, AFPs interact with the surface of ice crystals, inhibiting their growth. Winter plants produce AFPs that can adsorb onto the surface of ice crystals and alter their growth. The thermal hysteresis kinetics refers to the phenomenon where the higher the concentration of AFP in solution, the greater the TH effect (Fig. 2).
Figure 2: Mechanism of action of AFPs: AFPs rely on binding at the water-ice interface to inhibit the formation of ice crystals, and the specific mechanism of action is shown in the figure
5.1 Mechanism of Rigid-Body Energetics
AFPs exist as tiny particles in solution and interact with ice crystals and water during the process of ice crystal growth. Due to the difference in surface energy between AFPs-ice and AFPs-water, water molecules tend to reduce the surface area to minimize interfacial energy. Therefore, when AFPs bind to the surface of ice crystals, the ice-water interface area decreases, leading the system to reach equilibrium. Water molecules push AFPs on the surface of ice crystals, causing them to form an ice lattice in the solution. When the temperature drops below the supercooling point of the water solution, significant crystallization pressure is generated. However, due to the matching of AFPs with the ice crystal structure, the crystallization pressure exerted by water molecules keeps the AFPs in balance on the surface of the ice crystal, preventing them from advancing further. Therefore, ice crystal growth only occurs in the supercooled state where it can engulf these particles, leading to a decrease in the freezing point.
5.2 Mechanism of Ice Crystal Adsorption Inhibition
Under low-temperature conditions, AFPs exhibit selective adsorption and bind to the surfaces of ice crystals after mixing with them. AFP molecules bind to water molecules on the surface of ice crystals, causing the covered areas to cease growing, while the uncovered regions continue to advance, resulting in a circular surface and increased surface curvature. When the ratio of the ice crystal surface area to its volume exceeds the thermodynamic value for spontaneous ice growth, the growth of ice crystals is inhibited, resulting in a decrease in the freezing point. In this process, AFPs continuously adsorb onto the surface of ice crystals until the ice crystals are melted due to external influences, at which point AFPs detach from the ice crystal surface. The higher the concentration of AFPs, the better their effectiveness. Research has shown that the accumulation rate of AFPs is not limited by diffusion but rather by adsorption kinetics. Mass transfer limitations do not affect the biological function of AFPs [38]. The adsorption inhibition mechanism has been widely accepted in current AFP research. Recent studies have utilized super-resolution techniques combined with cryogenic imaging to investigate the interfacial dynamics between individual AFPs and ice crystal surfaces, providing direct evidence for the adsorption and inhibition mechanisms of AFPs [39]. Indeed, this mechanism can be used to calculate the extent of freezing point depression caused by AFPs adsorbing onto the surface of ice crystals.
5.3 Mechanism of Thermal Hysteresis Kinetics
Research has shown that THA is correlated with the concentration of AFPs. AFPs can interact with ice crystal planes and adsorb onto the surface of ice crystals, lowering the freezing point on the ice crystal surface and raising the melting point within the ice crystal, thereby generating TH. The increase in TH is directly proportional to the square of protein concentration. Different types of AFPs generate different levels of TH at the same concentration. AFPs also can shape the crystal morphology of ice surfaces and influence the growth structure of ice crystals in solution. Under the influence of active AFPs, ice crystals tend to grow along the c-axis direction. In the absence of AFPs in the solution, ice crystals tend to grow larger and exhibit a flattened disc shape as the temperature decreases. The freezing point and melting point of the ice are equal under these conditions.
6 Applications of Antifreeze Proteins in Everyday Life
6.1 Application of Antifreeze Proteins in Agricultural Breeding
Plant AFPs are commonly used in agriculture as biostimulants and germination promoters [40]. Additionally, due to their ability to exhibit TH, they can also be used to prevent damage from mild frosts that commonly occur in early autumn and late spring to frost-sensitive crops [41,42]. Therefore, the expression of highly active AFPs allows them to remain frozen at temperatures close to −5°C. Over the past two decades, the effectiveness of this concept has been tested in various studies involving the production of transgenic plants (such as Arabidopsis and several crop plants) expressing different AFPs. Similarly, some AFPs derived from transgenic plants may expand the growth season and geographical range of crops like potatoes (Solanum tuberosum), rapeseed (Brassica rapa) leaves, and wheat (Triticum aestivum). However, various studies have reported limited effects of AFPs on plant crops, thus further research is greatly needed.
6.2 Application of Antifreeze Proteins in the Food Industry
Common cryoprotectants such as glycerol, sugars, and Dimethyl sulfoxide (DMSO) can be toxic to certain plant tissues and can lower the freezing point depending on their concentration. The reduction of freezing points by AFPs is non-colligative and does not significantly alter melting points regardless of concentration [43]. Previous studies have reported that low concentrations of AFPs effectively inhibit ice recrystallization. AFPs are highly sought after for their unique abilities and environmentally friendly nature, making them suitable for cryopreservation, biotechnology, and food industries. Adding AFPs to cells, organs, and tissues of plants and animals has been proven to improve cryopreservation efficiency. In terms of food applications, AFPs enhance the texture of ice cream and the quality of cured meat [44]. Plant-based meat (PBM), as a new substitute for animal meat, often encounters quality issues during commercial transportation and home storage due to freeze-thaw cycles. To minimize the degradation of PBM performance caused by these freeze-thaw cycles, recent research involves the heterologous expression of DcAFP and its deglycosylated mutant DcAFP-n294g in Pichia pastoris ×33 yeast, where recombinant antifreeze proteins (rAFPs) pretreatment results in a smoother and flatter surface of high-moisture protein extrudates. Deglycosylated DcAFP as a novel and effective cryoprotectant for meat substitutes shows potential for future applications [45].
6.3 Application of Antifreeze Proteins in Biotechnology
Introducing genes encoding AFPs through metabolic engineering is another promising strategy for enhancing the freezing tolerance of transgenic animals and plants [38]. Numerous studies have attempted to develop freezing-tolerant plants and animals by overexpressing AFP genes in other cold-sensitive organisms. It has been reported that many species have produced transgenic plants overexpressing fish AFPs, including Arabidopsis, tobacco, tomato, and potato. Recently, success in this field has significantly increased and has been used to alter plant genetics for the development of higher-quality foods and fruits [46]. Similarly, some AFPs derived from transgenic plants may expand the growth season and geographical range of crops like potatoes, rapeseed, and wheat. These genetically modified plants may provide food security for humans shortly after climate change. Therefore, further research is urgently needed. In the medical field, AFPs are useful for cryopreservation of organs, embryos, and oocytes, improving the efficiency of cryogenic storage [47]. Compared to synthetic cryoprotectants like DMSO, using AFP as a cryoprotectant can reduce damage and death rates of stored organs, cells, and tissues [48].
AFPs are a group of proteins expressed in vertebrates, invertebrates, plants, bacteria, and fungi that protect organisms from the effects of deep freezing temperatures. Indeed, AFPs have potential industrial, medical, and agricultural applications in various fields. However, their application is hindered by high costs [49]. Plant AFPs can be classified into two main types: structural and non-structural AFPs. The molecular structure and functional mechanisms of structural AFPs have been extensively studied and explored. In contrast, research on non-structural AFPs is still in its early stages.
In recent years, one of the primary challenges in AFP research has been how to produce a large quantity of AFPs while maintaining their stability. Currently, research in this area is mainly focused on the following directions: (1) Enhancing the natural production of AFPs in plants through genetic engineering techniques. (2) Expressing exogenous AFP proteins in microbes or other hosts and purifying AFPs through methods like engineered bacterial fermentation. (3) Utilizing chemical synthesis methods to produce AFP proteins. The future research directions mainly include the following aspects: (1) Further optimizing the production and extraction techniques of AFPs to reduce costs and increase yield. (2) Utilizing modern gene editing technologies to develop novel and efficient AFPs for widespread applications. (3) Exploring the applications of AFPs in medicine and biomaterials, such as in the development of artificial organs and biomedical materials.
In addition to the technical challenges and future directions mentioned above, AFP gene research also faces the following issues: (1) The functionality and regulatory mechanisms of AFP genes may vary among different plant species. Therefore, it is necessary to research multiple species to gain a more comprehensive understanding of the functions and regulatory mechanisms of AFP genes. (2) Although AFP genes have been proven to enhance plant freezing tolerance, the extent of improvement is still relatively small. Therefore, there is a need to search for more effective cryoprotective genes and strategies, as well as explore how to combine multiple cryoprotective genes to enhance freezing tolerance. (3) The roles and regulatory mechanisms of AFP genes in various aspects of plant growth and development still require further investigation. (4) Despite significant progress in AFP gene research over the past few decades, there is still a need for broader international collaboration to accelerate research progress and improve the level and quality of research. Research on these issues will help explain the mechanisms of AFPs in plant freezing tolerance and provide a solid foundation for utilizing plant physiology and genetic engineering techniques to enhance plant freezing resistance.
Acknowledgement: Not applicable.
Funding Statement: This research was funded by the Fundamental Research Funds for the Central Universities under Grant No. BFUKF202309, Special Scientific Research Fund of Talents Introduced into Hebei Agricultural University under Grant No. YJ2022025, Basic Scientific Research Projects of Provincial Universities in Hebei Province under Grant No. KY202203, Hebei Agriculture Research System under Grant No. HBCT2024200101, S&T Program of Hebei under Grant No. 21326301D.
Author Contributions: Conceptualization, P.L.; writing—original draft preparation, Z.Z. and W.L.; writing—review and editing, Y.H. and P.L.; supervision, Y.H.; project administration, P.L.; funding acquisition, Y.H. and P.L. All authors have read and agreed to the published version of the manuscript.
Availability of Data and Materials: Data sharing does not apply to this article.
Ethics Approval: Not applicable.
Conflicts of Interest: The authors declare that they have no conflicts of interest to report regarding the present study.
References
1. Chen SQ, Sun JS, Ma WJ, Wang JH, Zhao XY, Hu RY. Regulation mechanism of low temperature stress on plants: research progress. Chin Agric Sci Bull. 2022;38(17):51–61 (In Chinese). [Google Scholar]
2. Ding Y, Shi Y, Yang S. Molecular regulation of plant responses to environmental temperatures. Mol Plant. 2020;13(4):544–64. doi:10.1016/j.molp.2020.02.004. [Google Scholar] [PubMed] [CrossRef]
3. Yu H, Zheng H, Liu Y, Yang Q, Li W, Zhang Y, et al. Antifreeze protein from Ammopiptanthus nanus functions in temperature-stress through domain A. Sci Rep. 2021;11(1):8458. doi:10.1038/s41598-021-88021-0. [Google Scholar] [PubMed] [CrossRef]
4. Ewart KV, Lin Q, Hew CL. Structure, function and evolution of antifreeze proteins. Cell Mol Life Sci. 1999;55(2):271–83. doi:10.1007/s000180050289. [Google Scholar] [PubMed] [CrossRef]
5. Yeh Y, Feeney RE. Antifreeze proteins: structures and mechanisms of function. Chem Rev. 1996;96(2):601–18. doi:10.1021/cr950260c. [Google Scholar] [PubMed] [CrossRef]
6. Satyakam, Zinta G, Singh RK, Kumar R. Cold adaptation strategies in plants-An emerging role of epigenetics and antifreeze proteins to engineer cold resilient plants. Front Genet. 2022;13:909007. doi:10.3389/fgene.2022.909007. [Google Scholar] [PubMed] [CrossRef]
7. Gupta R, Deswal R. Antifreeze proteins enable plants to survive in freezing conditions. J Biosci. 2014;39(5):931–44. doi:10.1007/s12038-014-9468-2. [Google Scholar] [PubMed] [CrossRef]
8. Wang R, Li R, Sun Z, Ren Y, Yue W. Anti-freezing proteins and plant responses to low temperature stress. Ying Yong Sheng Tai Xue Bao. 2006;17(3):551–6 (In Chinese). [Google Scholar] [PubMed]
9. Fei Y, Wei L, Gao S, Lu M, Wang B, Lin Z, et al. Isolation, purification and characterization of secondary structure of antifreeze protein from Ammopiptanthus mongolicus. Chin Sci Bull. 2001;46(6):495–8 (In Chinese). doi:10.1007/BF03187266. [Google Scholar] [CrossRef]
10. Fei YB, Cao PX, Gao SQ, Wang B, Wei LB, Zhao J, et al. Purification and structure analysis of antifreeze proteins from Ammopiptanthus mongolicus. Prep Biochem Biotechnol. 2008;38(2):172–83. doi:10.1080/10826060701885126. [Google Scholar] [PubMed] [CrossRef]
11. Urrutia ME, Duman JG, Knight CA. Plant thermal hysteresis proteins. Biochim Biophys Acta. 1992;1121(1–2):199–206. [Google Scholar] [PubMed]
12. Zachariassen KE, Kristiansen E. Ice nucleation and antinucleation in nature. Cryobiology. 2000;41(4):257–79. doi:10.1006/cryo.2000.2289. [Google Scholar] [PubMed] [CrossRef]
13. Baskaran A, Kaari M, Venugopal G, Manikkam R, Joseph J, Bhaskar PV. Anti freeze proteins (Afpproperties, sources and applications-A review. Int J Biol Macromol. 2021;189:292–305. doi:10.1016/j.ijbiomac.2021.08.105. [Google Scholar] [PubMed] [CrossRef]
14. Atici O, Nalbantoglu B. Antifreeze proteins in higher plants. Phytochemistry. 2003;64(7):1187–96. doi:10.1016/S0031-9422(03)00420-5. [Google Scholar] [PubMed] [CrossRef]
15. Lee JC, Hansen T, Davies PL. Droplet freezing assays using a nanoliter osmometer. Cryobiology. 2023;113(11):104584. [Google Scholar] [PubMed]
16. Eslami M, Shirali Hossein Zade R, Takalloo Z, Mahdevar G, Emamjomeh A, Sajedi RH, et al. afpCOOL: a tool for antifreeze protein prediction. Heliyon. 2018;4(7):e00705. doi:10.1016/j.heliyon.2018.e00705. [Google Scholar] [PubMed] [CrossRef]
17. Khan A, Uddin J, Ali F, Ahmad A, Alghushairy O, Banjar A, et al. Prediction of antifreeze proteins using machine learning. Sci Rep. 2022;12(1):20672. doi:10.1038/s41598-022-24501-1. [Google Scholar] [PubMed] [CrossRef]
18. Kuiper MJ, Davies PL, Walker VK. A theoretical model of a plant antifreeze protein from Lolium perenne. Biophys J. 2001;81(6):3560–5. doi:10.1016/S0006-3495(01)75986-3. [Google Scholar] [PubMed] [CrossRef]
19. Fan Y, Liu B, Wang H, Wang S, Wang J. Cloning of an antifreeze protein gene from carrot and its influence on cold tolerance in transgenic tobacco plants. Plant Cell Rep. 2002;21(4):296–301. doi:10.1007/s00299-002-0495-3. [Google Scholar] [CrossRef]
20. Wisniewski M, Webb R, Balsamo R, Close T, Yu X, Griffith M. Purification, immunolocalization, cryoprotective, and antifreeze activity of PCA60: a dehydrin from peach (Prunus persica). Physiol Plant. 1999;105(4):600–8. doi:10.1034/j.1399-3054.1999.105402.x. [Google Scholar] [CrossRef]
21. Smallwood M, Worrall D, Byass L, Elias L, Ashford D, Doucet CJ, et al. Isolation and characterization of a novel antifreeze protein from carrot (Daucus carota). Biochem J. 1999;340(2):385–91. doi:10.1042/bj3400385. [Google Scholar] [CrossRef]
22. Gupta R, Deswal R. Low temperature stress modulated secretome analysis and purification of antifreeze protein from Hippophae rhamnoides, a Himalayan wonder plant. J Proteome Res. 2012;11(5):2684–96. doi:10.1021/pr200944z. [Google Scholar] [PubMed] [CrossRef]
23. Simpson DJ, Smallwood M, Twigg S, Doucet CJ, Ross J, Bowles DJ. Purification and characterisation of an antifreeze protein from Forsythia suspensa (L.). Cryobiology. 2005;51(2):230–4. doi:10.1016/j.cryobiol.2005.06.005. [Google Scholar] [PubMed] [CrossRef]
24. Jarzabek M, Pukacki PM, Nuc K. Cold-regulated proteins with potent antifreeze and cryoprotective activities in spruces (Picea spp.). Cryobiology. 2009;58(3):268–74. doi:10.1016/j.cryobiol.2009.01.007. [Google Scholar] [PubMed] [CrossRef]
25. Middleton AJ, Marshall CB, Faucher F, Bar-Dolev M, Braslavsky I, Campbell RL, et al. Antifreeze protein from freeze-tolerant grass has a beta-roll fold with an irregularly structured ice-binding site. J Mol Biol. 2012;416(5):713–24. doi:10.1016/j.jmb.2012.01.032. [Google Scholar] [PubMed] [CrossRef]
26. Jin ZY, Liu BL. Purification and physicochemical properties of antifreeze protein in wheat bran. Sci Technol Food Ind. 2015;20:159–63. [Google Scholar]
27. Zhang SH, Wei Y, Liu J, Yu HM, Yin JH, Pan H. An apoplastic chitinase CpCHT1 isolated from the corolla of wintersweet exhibits both antifreeze and antifungal activities. Biol Plant. 2011;55(1):141–8. doi:10.1007/s10535-011-0019-5. [Google Scholar] [CrossRef]
28. Duman JG. Purification and characterization of a thermal hysteresis protein from a plant, the bittersweet nightshade Solanum dulcamara. Biochim Biophys Acta. 1994;1206(1):129–35. doi:10.1016/0167-4838(94)90081-7. [Google Scholar] [PubMed] [CrossRef]
29. Dong X, Liu Z, Mi W, Xu C, Xu M, Zhou Y, et al. Overexpression of BrAFP1 gene from winter rapeseed (Brassica rapa) confers cold tolerance in Arabidopsis. Plant Physiol Biochem. 2020;155(1):338–45. [Google Scholar] [PubMed]
30. Cai Y, Liu S, Liao X, Ding Y, Sun J, Zhang D. Purification and partial characterization of antifreeze proteins from leaves of Ligustrum lucidum Ait. Food Bioprod Process. 2011;89(2):98–102. doi:10.1016/j.fbp.2010.04.002. [Google Scholar] [CrossRef]
31. Zhang M, Lai ZX, Cai YQ. Cloning and analysis of chiitin antifreeze protein gene (ChiI.2) in wild banana. J Longyan Univ. 2016;34(5):120–4 (In Chinese). [Google Scholar]
32. Qiu H, Zhang L, Liu C, He L, Wang A, Liu HL, et al. Cloning and characterization of a novel dehydrin gene, SiDhn2, from Saussurea involucrata Kar. et Kir. Plant Mol Biol. 2014;84(6):707–18. doi:10.1007/s11103-013-0164-7. [Google Scholar] [PubMed] [CrossRef]
33. Kim M, Gwak Y, Jung W, Jin E. Identification and characterization of an isoform antifreeze protein from the antarctic marine diatom, Chaetoceros neogracile and suggestion of the core region. Mar Drugs. 2017;15(10):318. doi:10.3390/md15100318. [Google Scholar] [PubMed] [CrossRef]
34. Nakamura T, Ishikawa M, Nakatani H, Oda A. Characterization of cold-responsive extracellular chitinase in bromegrass cell cultures and its relationship to antifreeze activity. Plant Physiol. 2008;147(1):391–401. doi:10.1104/pp.106.081497. [Google Scholar] [PubMed] [CrossRef]
35. Kawahara H, Fujii A, Inoue M, Kitao S, Fukuoka J, Obata H. Antifreeze activity of cold acclimated Japanese radish and purification of antifreeze peptide. Cryo Letters. 2009;30(2):119–31. [Google Scholar] [PubMed]
36. Lu CF, Jian LI, Kuang TY. Secretory antifreeze proteins produced in suspension culture cells of rhodiola algida var. tangutica during cold acclimation. Prog Biochem Blophys. 2000;27(5):558–9 (In Chinese). [Google Scholar]
37. Hon WC, Griffith M, Chong P, Yang D. Extraction and isolation of antifreeze proteins from Winter Rye (Secale cereale L.) Leaves. Plant Physiol. 1994;104(3):971–80. doi:10.1104/pp.104.3.971. [Google Scholar] [PubMed] [CrossRef]
38. Thosar AU, Shalom Y, Braslavsky I, Drori R, Patel AJ. Accumulation of antifreeze proteins on ice is determined by Adsorption. J Am Chem Soc. 2023;145(32):17597–602. doi:10.1021/jacs.3c02705. [Google Scholar] [PubMed] [CrossRef]
39. Tas RP, Hendrix MMRM, Voets IK. Nanoscopy of single antifreeze proteins reveals that reversible ice binding is sufficient for ice recrystallization inhibition but not thermal hysteresis. Proc Natl Acad Sci U S A. 2023;120(2):e2212456120. doi:10.1073/pnas.2212456120. [Google Scholar] [PubMed] [CrossRef]
40. Jardin PD. Plant biostimulants: definition, concept, main categories and regulation. Sci Hortic. 2015;196:3–14. doi:10.1016/j.scienta.2015.09.021. [Google Scholar] [CrossRef]
41. Wisniewski M, Willick IR, Duman JG, Livingston D, Newton SS. Plant antifreeze proteins. In: Ramløv H, Friis D, editors. Antifreeze proteins. Cham: Springer; 2020. vol. 1, p. 189–226. [Google Scholar]
42. Cutler A, Saleem M, Kendall E, Gusta L, Georges F, Fletcher G. Winter flounder antifreeze protein improves the cold hardiness of plant tissues. J Plant Physiol. 1989;135(3):351–4. doi:10.1016/S0176-1617(89)80131-2. [Google Scholar] [CrossRef]
43. Venketesh S, Dayananda C. Properties, potentials, and prospects of antifreeze proteins. Crit Rev Biotechnol. 2008;28(1):57–82. doi:10.1080/07388550801891152. [Google Scholar] [PubMed] [CrossRef]
44. Białkowska A, Majewska E, Olczak A, Twarda-Clapa A. Ice binding proteins: diverse biological roles and applications in different types of industry. Biomolecules. 2020;10(2):274. doi:10.3390/biom10020274. [Google Scholar] [PubMed] [CrossRef]
45. Wu X, Zhang C, Yu S, Chen J, Zhou J. Improving the cryoprotective effect of antifreeze proteins from Daucus carota on plant-based meat by eliminating N-glycosylation. Food Res Int. 2023;164(4):112392. [Google Scholar] [PubMed]
46. Eskandari A, Leow TC, Rahman MBA, Oslan SN. Antifreeze proteins and their practical utilization in industry, medicine, and agriculture. Biomolecules. 2020;10(12):1649. doi:10.3390/biom10121649. [Google Scholar] [PubMed] [CrossRef]
47. Robles V, Valcarce DG, Riesco MF. The use of antifreeze proteins in the cryopreservation of gametes and embryos. Biomolecules. 2019;9(5):181. doi:10.3390/biom9050181. [Google Scholar] [PubMed] [CrossRef]
48. Naing AH, Kim CK. A brief review of applications of antifreeze proteins in cryopreservation and metabolic genetic engineering. 3 Biotech. 2019;9(9):329. doi:10.1007/s13205-019-1861-y. [Google Scholar] [PubMed] [CrossRef]
49. Cheung RCF, Ng TB, Wong JH. Antifreeze proteins from diverse organisms and their applications: an overview. Curr Protein Pept Sci. 2017;18(3):262–83. doi:10.2174/1389203717666161013095027. [Google Scholar] [PubMed] [CrossRef]
Cite This Article
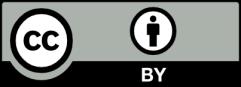
This work is licensed under a Creative Commons Attribution 4.0 International License , which permits unrestricted use, distribution, and reproduction in any medium, provided the original work is properly cited.