Open Access
REVIEW
The Correlation between Nutrition and Transport Mechanism under Abiotic Stress in Plants: A Comprehensive Review
1 Joint International Research Laboratory of Agriculture and Agri-Product Safety, The Ministry of Education of China, Jiangsu Key Laboratory of Crop Genomics and Molecular Breeding, Yangzhou University, Yangzhou, 225009, China
2 Microelement Research Center, College of Resources and Environment, Huazhong Agricultural University, Wuhan, 430070, China
3 Department of Agronomy, Faculty of Agriculture and Environment, The Islamia University of Bahawalpur, Bahawalpur, 63100, Pakistan
* Corresponding Author: Li Song. Email:
(This article belongs to the Special Issue: Abiotic and Biotic Stress Tolerance in Crop)
Phyton-International Journal of Experimental Botany 2024, 93(6), 1325-1344. https://doi.org/10.32604/phyton.2024.048493
Received 09 December 2023; Accepted 13 May 2024; Issue published 27 June 2024
Abstract
Variations in the nutrients and water that plants require for metabolism, development, and the maintenance of cellular homeostasis are the main causes of abiotic stress in plants. It has, however, hardly ever been studied how these transporter proteins, such as aquaporin which is responsible for food and water intake in cell plasma membranes, interact with one another. This review aims to explore the interactions between nutrient transporters and aquaporins during water and nutrient uptake. It also investigates how symbiotic relationships influence the plant genome’s responses to regulatory processes such as photoperiodism, senescence, and nitrogen fixation. These responses are observed in reaction to various abiotic stresses. For instance, plasma membrane transporters are upregulated during macronutrient insufficiency, tonoplast transporters are overexpressed, and aquaporins are downregulated in micronutrient deficiency. Additionally, tolerant plants often exhibit increased expression of nutrient transporters and aquaporins in response to drought, salt, and cold temperatures. To better comprehend plant stress tolerance to abiotic challenges including starvation, K famine, salt, and freezing temperatures, both classes of nutrient and water transporters should be considered at the same time.Keywords
Plants are commonly impacted by a variety of abiotic stresses, including salt, metal toxicity, drought, temperature extremes, and nutrient deficiency. These stresses have the potential to impair a plant’s capacity to grow or die [1]. Nutrient deficiency is one of the most common abiotic stresses encountered by plants, which can exacerbate the effects of other stresses. The majority of research over the last few centuries has focused on the connection between abiotic stress and plant nutrition. Studies have demonstrated that several crucial macro- and micro-nutrients support plants’ ability to survive abiotic stress [2]. Nitrogen (N) is one of the nutrients with the greatest influence on plant response against abiotic stresses. It is important for the growth and ontogeny of plants, in addition to being a key component of plant proteins and other critical components [3,4]. Lack of N can result in slower plant growth, fewer fruits and vegetables, and plants are more vulnerable to abiotic stress. In drought-stressed plants, N deficit can direct to a decrement in water usage efficiency (WUE), making them more susceptible to drought stress [5]. Numerous studies have shown that N addition to soil can boost plant tolerance to drought stress by enhancing WUE, thereby reducing water loss caused by transpiration.
Phosphorus (P) is another macro-nutrient that affects how plants respond to abiotic stress. It is essential for energy production, metabolism, cell division, and other vital cellular processes [6]. Phosphorus deficiency can impair plant growth, reduce yields, and make plants more vulnerable to abiotic stress. Consumption of P can enhance plant resistance to abiotic stresses [7]. For instance, supplementing plants under salt stress with P can encourage growth while minimizing the negative effects of the salts through ion balance and osmotic adjustment. Similarly, P addition to soils with high amounts of toxic metals can hasten plant growth and development [8]. Similarly, calcium (Ca) is vital for cell wall production, the activation of enzymes, and many other vital cellular processes [9]. Plants deficient in Ca may grow slowly, produce fewer fruits and vegetables, and be more vulnerable to abiotic stresses. Multiple research investigations have demonstrated that adding Ca to soil improves plant resistance to abiotic stress [10]. In heat-stressed plants, for example, Ca application can improve plant development and reduce the making of reactive oxygen species, which can lead to oxidative harm. Similar to this, Ca therapy can promote plant growth and mitigate the negative effects of salt on plants under salt stress [11]. Many investigations have been reported on the impacts of macro- and micro-nutrient deficiency on plant growth and productivity in the form of stresses. The available information about the relationship between nutrient availability and their transport mechanisms affecting abiotic stress in plants was missing. This comprehensive review elucidates a state-of-the-art review of the literature about the same to fill this gap.
2 Macro-Nutrient Deficiencies Affecting Physiological Attributes of Plant
The macronutrients required for robust plant development are more densely packed in plant tissue than 0.1% based on the dried matter [12]. Macro-nutrient perniciousness stress is unique in the environment since macro-nutrients are needed in high quantities [13]. However, a lack of one or more macro-nutrients has a significant negative impact on several physiological traits of plants, including transporters and aquaporins.
Nitrogen is absorbed alternately as the nitrate (NO3−), which is the major type of intake, or as the ammonium (NH4+) [14]. The majority of the characterized NO3− transporters belong to the large protein family NRT. They are made up of transporters that carry both low-and high-affinity molecules, such as NRT1 and NRT2, respectively [15]. However, it has been discovered that several NRT1 transporters, including AtNRT1;1 (also known as CHL1), exhibit a dual affinity for NO3−. As a result, in low NO3− states, the NRT2 transporters absorb around 95% of the sum quantity of this anion, being the largest impart. N deficit enhanced the amount of NRT2.2, NRT2.3, NRT2.5, and NRT2.6 scripts in sorghum (Sorghum vulgare Pers, syn), but NRT2.5 has been demonstrated to be the only transcript elevated in wheat plants under NO3− deficiency [16]. T Plants lacking nitrogen exhibit a significant reduction in the hydraulic conductivity of their roots, reflective of the roots’ crucial role in water transport from the root zone to the stem xylem through a water-tensioned slope [17]. N deficiency decreases aquaporin activity or the manifestation of low-N conditions, as evidenced by the significant proportion of aquaporins to the root value of Lp. Furthermore, earlier studies on the Arabidopsis plant found that after 6 days of N shortage, the expression of the water channels PIP2.1, PIP2.2, PIP2.4, PIP1.2, and PIP1.3 in the root plasma membrane decreased [18]. These results suggest that aquaporins may be subject to complex transcriptional and posttranscriptional regulation by the NO3− sensor NRT2.1, which may modulate the N-dependent root Lp. Despite these results, additional study is necessary to completely comprehend the control mechanism [19]. The principal NH4+ transporters used by roots to absorb NH4+ are Ammonium Transporters (AMT), which are found in the plasma membrane. The movement of iron from the cytosol to the vacuole is necessary to store N and stop plant toxicity [20]. A. thaliana cells most likely facilitate this transport in the vacuolar membrane water channels AtTIP2.1 and AtTIP2.3 [21]. As a result, it could be immobilized using facilitated diffusion as needed. Aquaporins of type TIP may frequently be implicated in the description of low-affinity transport pathways. Several TIPs, including ZmTIP1.1 and ZmTIP1.2, are engaged in NH4+ captivation in corn plants. The plasma membrane, members of the PIP and NIP subfamilies, as well as the tonoplast TIP subfamily [19], all aid in the transport of urea across membranes. ZmTIP4.4 expression has considerably increased in roots and enlarged leaves under conditions of N deprivation, showing that ZmTIP4.4 is outflow to the cytoplasm required for urea. This information suggests that the aquaporins function as a urea transporter by mobilizing reversion pools in the vacuole during N shortage [22].
The majority of the potassium (K) routes are transporters passively. However, other K transporters frequently act as protein transporters with H+ and sodium ion (Na+) transporters [23]. In A. thaliana, researchers have identified a sum of 71 potassium transporters, channels, and carrier proteins [24]. They are further split into 3 channel families and 4 transporter families. In research using the A. thaliana plant, were demonstrated to be upgraded and triggered by a K deficiency. K deficiency in rice plants led to the formulation of OsAKT1, OsHAK1, and OsPIP2.7 [25,26]. An increase in aquaporin activity or density may have contributed to the rice root Lp increment that was shown by the same researchers to occur in response to K deficiency. Plants with K deficiency have altered membrane potential, turgor pressure, and pH control, affecting aquaporin function and gene expression. Stomatal dysfunction, ROS generation, and cellular signaling pathways influence water transport dynamics. Nutrient availability affects aquaporin transcription and post-translational regulation, altering plant water balance (4). Other researchers who similarly asserted that aquaporins genes were noticeably enhanced following K render reported that A. thaliana plants possessed 12 aquaporins genes in the shoots and 15 in the roots [19]. In low K conditions, barley plants saw both increases and decreases in the number of gene transcripts. Additionally, they found that root Lp had decreased. The unique reactivity of the PIP isoforms or the different testing techniques [27]. Plant responses might be a result of both the plant’s immediate response to changes in K availability and the long-term impacts of K famine. K channels and aquaporins were operationally co-regulated during the control of cell turgor; this could be an indication of the co-regulation of transporters in roots, opening the door to the potential action of identifying an unidentified regulation component for ion and water mediums [28].
Phosphorus is typically co-transported from the soil in its amorphous state (Pi), which demands energy [29]. As a result, we can recognize Pi transporters with up and down affinity whose actions are mainly regulated by the P concentrations in the rhizosphere [30]. In A. thaliana and gene plants, 5 excessive-affinity Pi transporters have since been identified. Particularly, it has been shown that Pi deficiency predominates among PHT1 group members. AtPHT1.4 could become dominant by upregulating in response to Pi scarcity, as shown in the plant species A. thaliana and wheat [31]. However, under low P conditions, the majority of the PHT1 genes in soybean (Glycine max L.) plants showed greater transcript degree. In addition, P deprivation may alter the way the aquaporins and Lp genes are expressed in roots and cause the establishment of Casparian strips in roots [32]. Additionally, PHT1 genes are increased during lower-P concentration conditions in soybean plants to increase phosphate absorption. Aquaporins and Lp genes, which carry water and nutrients, are also affected. The plant optimizes PHT1 expression to increase P absorption and aquaporins and Lp gene expression to promote water and nutrient transport [23]. As an illustration. Leymus chinesis L. has six distinct PIP water channels as a result of decreased phosphorus accessibility [33]. Certainly, P starvation may alter aquaporin function due to changes in the phosphorylation state of aquaporins. The NIP-type water channel ZmNIP2.1 and the excessive-affinity Pi transporters ZmPHT4.2 and ZmPHT4.6 were found to be correlated in corn plants, suggesting a potential macrocosm mechanism.
Sulfate ion (SO42−) is a family of excessive-affinity transmembrane ions, and SO42− transporters [34], such as SULTR1.1, SULTR1.3, SULTR1.2, SULTR3.1, SULTR3.4, SULTR3.5, SULTR3.6, and SULTR4.1, transport sulfur (S). When A. thaliana is deprived of S, the formulation of the SO42− genes carrier AtSultr1.1, AtSultr1.2, and AtSultr4.1 increases, highlighting the significance of these excessive-affinity transporters. Despite the lack of evidence connecting PIP aquaporin activity or expression to S transporters [35]. The ZmSULTR2.1 exhibited comparable formulation techniques to ZmTIP2.2, whereas ZmSULTR3.4 demonstrated a similar pattern. This finding provides evidence that the transportation of S from the vacuolar sulfur pool to the cytoplasm is facilitated by aquaporins [36,37]. This could serve as a potential foundation for future research exploring the correlation between S transporters and aquaporins in plants experiencing stress.
Calcium deficiency is uncommon due to how common and accessible Ca is in soils [38]. However, severe weathering and soil leaching can lead to Ca deficiencies [39]. Ca ion (Ca2+) channels, which can be Ca2+-selective or non-selective ion mediums, allow Ca2+ to enter the roots. The efflux of Ca2+ into the cytosol is mediated by 3 distinct kinds of channels [40]. In counterpoint, Ca2+ efflux from the cytosol is carried out by Ca2+-ATPases, which are split into 2 classifications (P-type ATPase IIA and IIB), or H+/Ca2+-anti-porters, which require vigorous transport activity. Cation exchangers (CAXs). Under Ca deprivation, amaranth plants specifically showed an elevation of the AhCAX and calmodulin genes. On the other hand, Ca deficiency often results in the de-regulation of water channel gene formulation, even though it has little to no effect on water relations throughout the entire plant [41]. The transport of Ca2+ across roots was largely apoplastic. Therefore, it has been proposed that a reduction in symplastic water permeability, which depends on aquaporin functions, may be required when Ca is limited [42], leading to a transition to apoplastic water flow. It was discovered that the plasma membrane’s aquaporin function improved when Ca2+ was given to the protoplasts [42]. In actuality, aquaporin activity was not detected in protoplasts isolated from pepper plants growing below Ca deficiency. Additionally, a Ca2+ channel blocker did not affect aquaporin functionality. Additionally, the corn gene ACA4 (calmodulin-regulated Ca2+ pump in the endoplasmic reticulum) revealed a similar pattern to NIP2;2 [43]. The activity of aquaporins can be directly impacted by divalent cations like Ca2+. Additionally, rather than only altering the integrity of the plasma membrane, Ca2+ appears to be intricated in the regulation of the plasma membrane water channel through several cellular procedures [44]. Even if a connection between the Ca2+ transporters and aquaporins has not been demonstrated, these data may point to an interaction between aquaporins and Ca2+ transporters that must be further investigated. The Ca regulates aquaporins through phosphorylation, cytoskeleton dynamics, intracellular signaling, vesicle trafficking, direct binding, ROS signaling, and cellular water homeostasis. These processes allow plants to dynamically adjust water permeability, responding to environmental cues and maintaining optimal water balance in varying conditions [37].
The bioavailability of magnesium (Mg) is decreased by a variety of factors, including acidic soils, heavy rainfall in equatorial regions, aluminum toxicity, heat stress, soils impacted by drought, and excessive concentrations of ionic components (K, Ca, NH4+, and Na) [45]. Mg transporters, likewise, AtMHX (magnesium ion (Mg2+)/H+ inter-changer) in A. thaliana cells, maintain the Mg content in balance. The CorA family, belonging to the superfamily of Mg transporters, includes up-affinity, down-affinity, and dual-affinity transporters. These transporters play a key role in the transportation of Mg2+ in plants and are essential for maintaining Mg2+ levels, distribution, and cellular balance [46]. Under Mg-deficiency circumstances, Brassica napus L. plants significantly raised their levels of the Mg2+ transporters BnMGT1.2 and BnMGT6.1, which are associated with Mg2+ from root-to-shoot translocation. The BnMGT6.1 and BnMGT1.2 genes are linked to the translocation of Mg2+ from the root to the shoot. Its deficiency affects aquaporin function, which affects the movement of water and nutrients. Evidence points to the changed aquaporins phosphorylation and expression, emphasizing the function of aquaporins in Mg2+ transport within plants and connecting Mg2+ deprivation to variations in root hydraulic conductivity [46]. The fact that ZmPIP1.5 and the CorA-like family protein (MRS2-2) exhibit similar formulation methods in maize plants recommends a connection linking Mg deficiency and aquaporin action [47]. However, there is not any concrete evidence linking an Mg deficiency to decreased aquaporin activity.
3 The Influence of Micro-Nutrient Deficiencies on Physiological Attributes of Plant
The concentrations of micro-nutrients in most agricultural soils are deficient as indicated by the crop growth stages. This deficiency is replenished through adaptive mechanisms in plants as well as their application to soils [37]. Even though the amount of micro-nutrients in the soil may be enough overall, factors in the soil such as pH, microorganisms, and water status influence how easily those nutrients are absorbed by plants [48]. As mentioned below, it is necessary to study how transporters and aquaporins interact with one another. The micro-nutrients are transported through the major transporter families. The main efflux transporters are CAX and HMA, while the main inflow transporters are ZIP, NRAMP, and YSL [49]. The concentration of the nutrient and the cell’s location affect the precise tasks that they do. Every family has a member that can transport a variety of micro-nutrients, but the isoforms are quite specific [50]. However, under insufficient circumstances, certain over-expressed transporters can transport other similar cations, increasing their concentration.
Iron is a widely distributed metal in soil and is generally found in non-assessable (insoluble) patterns [51]. Alternatively, plants can conduct chelation in the rhizosphere or convert insoluble Fe3+ to soluble Fe2+ to absorb Fe from the soil [52]. The association linking Fe and water movement hasn’t been specifically looked at yet. Water flow in plants is influenced by Fe, and increased expression of AtNRAMP3 promotes Fe absorption. Water flow is therefore impacted as a result of variations in root hydraulic conductivity. Furthermore, certain hormones, like as abscisic acid, may alter stomatal closure, which affects water flow and plays a role in the complex control of Fe and water dynamics in plant cells [37]. During Fe deficiency, plants seem to exhibit a sign that may have an impact on how water flows by the plant [53]. The A. thaliana plants in Fe-deficient, the expression of tonoplast AtNRAMP3 and the levels of certain hormones, likewise Abscisic Acid, rise which removes Fe from vacuolar pools. Additionally, pericycle and vascular cells had the highest expression of the AtFRD3 plasma membrane transporter [54].
The transfer of Fe over long distances was enhanced by increasing AtYSL2 and AtNAS1. Additionally, a drop in PIP2.2 abundance in maize roots in response to Fe deprivation, but they were unable to find any relationships linking the 2 groups of transporters. Even though Fe capture and absorption have already been well deliberated, future studies on Fe mobility can start by examining the roles of water channels as plasma membrane proteins [55].
Because these minerals decrease Mn bioavailability, manganese (Mn) shortages are frequently observed in plants in soils with excessive concentrations of minerals [56], likewise Fe, Mg, Ca, or P. Manganese may be used as a substrate by a variety of membrane transporters that have been connected to manganese effluence. The transporter families can thus be separated based on their role in Mn outflow or inflow [57]. Therefore, NRAMP, ZIP, and YSL are Mn influx transporters. Mn is taken up by NRAMP1, the main element of the broad family of plasma membrane-located transporters known as NRAMP. Additionally, it has been discovered that elements of the ZIP family, which are typically present in the plasma membrane and contain 8 transmembrane constructs, transport metals in plants. AtZIP1, AtZIP2, AtZIP5, AtZIP6, AtZIP7, and AtZIP9 have all been connected to Mn absorption in A. thaliana investigations; AtZIP1 is decentralized in the vacuolar membrane transfer Mn from the vacuole to the cytoplasm, indicating that it is active [58]. A few YSL transporters, in the distance path and intra-cellular capture of metals which are proteins, have also been connected to Mn incursion. YSL 4 and 6 have been decentralized in chloroplast and vacuole membranes of A. thaliana, moving Mn to the cytoplasm from the organelles [59]. Moreover, the plant’s NRAMP and ZIP families of Mn transporters are essential for maintaining Mn homeostasis. Plants activate these transporters in response to stress to improve Mn absorption, enable root-to-shoot translocation, and control intracellular concentrations. Adaptive responses are facilitated by the root exudation of organic acids and interactions with stress-signaling pathways [53].
Copper (Cu) is transported by membrane transporters from numerous families, including COPT, ZIP, HMA, and YSL. COPT 1 and 2 as well as ZIP 2 and 4 are elevated in Cu deficiency [60]. These genes produce decentralized plasma membrane proteins that are mostly located in the root surface and the region of distinction. Furthermore, the tonoplast-specific COPT5 promotes incursion transport to increment the copper concentration in the cytosol. Additionally, the affluence of YSL 2 increments under Cu deficiency [61]. However, some important transporters that depend on the plant, such as HMA5, have a distinct function. Therefore, HMA5 is enhanced in rice to support long-distance Cu transport because it makes it easier for Cu to exit via the plasma membrane. Although HMA5 mediates Cu deluge to the vacuole in A. thaliana, Cu deficiency inhibits HMA5 formulation [62]. According to certain studies, Cu hirsutum L. plants have inadequate stomatal conductance, which causes the water flow to be reduced [63]. However, an excess of Cu may impair the ability of aquaporins to transport water across the plasma membrane. The blue Cu-binding protein is delineated to limit cotton GhPIP2.6 activeness, recommending that Cu extent may be able to regulate this aquaporin’s function by lowering the flow of water over it [64]. An excess of copper led to a reduction in the activation of genes involved in maintaining metal balance and the creation of aquaporins in A. thaliana vegetation. The arrangement of aquaporin genes was reduced in response to high Cu levels, which reduced water intake [65]. Plants deficient in Cu have reduced water flow due to altered stomatal conductance. Because Cu is necessary for aquaporins function, a lack of it may reduce aquaporins activity by causing improper protein folding, which in turn affects water transport. This demonstrates how important Cu is for controlling water flow and stomatal dynamics in plants [48]. Additionally, according to these researchers, the lower water flow was brought about by harm to the membrane’s structural integrity due to the aquaporins diminished capacity and expression in this circumstance. Travel and water consumption when under stress. It has been demonstrated that water flow was decreased in the leaves of Cu-deficiency cotton and safflower plants because these plants had weak stomatal conductivity [66].
Plants demonstrate zinc (Zn) assimilation using six transporter families, which encompass ZIP and proteins enabling cation diffusion. Elements of the ZIP family are the main Zn transporters in soil uptake because they proceed Zn from the apoplast to the cytosol [67]. During Zn deficiency, plants control Zn uptake and translocation via regulating cation diffusion facilitators (CDF) and natural resistance-associated macrophage proteins (NRAMP). By promoting Zn absorption from the soil and transferring it to different plant tissues, ZIP4 and other ZIP transporters aid in Zn assimilation and provide vital functions even in the face of low Zn availability [68]. The cytosolic Zn concentration is significantly regulated by CDF (outflow) and NRAMP (inflow), which control Zn transport at the vacuolar level. Under conditions of zinc deficiency, a significant increase in the expression level of ZIP transporters is observed, particularly in ZIP4, a transporter predominantly located in the plasma membrane of the root surface and epidermal cells [20]. Since various species of inflated plants include the transcription aspects that result in an increment in these ZIP transporters, it also indicates that higher plants commonly respond to Zn deficiency. The tonoplast’s NRAMP transporters are also upregulated, whilst CDF are de-regulated, to convey vacuolar Zn to the cytosol [68]. In addition, during periods of deficiency, HMA transporters, particularly HMA2, situated in the root vascular cell membrane, impact the Zn loading into the xylem for its subsequent transportation to the shoot. Moreover, these transporters work to facilitate the Zn transport to the plasma membrane, enabling its subsequent transportation to the xylem and, ultimately, the seedlings [69].
Lack of boron (B) is among the most common micronutrient deficits in crops. There are numerous ways that plants can assimilate B. It was originally believed that the sole way for B to enter cells and roots was by passive diffusion. This is most likely the prime procedure under ideal or excessive B conditions [70]. However, the particular outflow transporter BOR1, which is found in the inside construct of root cells, can enable this element to be actively taken up at shallow extraneous B concentrations [71]. B can also be transported by a few aquaporins, notably NIP5.1. Xylem damage brought on by a B deficit could lessen water transport to the leaves, which would affect how other elements are distributed [71]. Aquaporin NP5.1 expression in these conditions was about 15-fold higher in A. thaliana plants. NIP6.1, predominantly found in the phloem of stems, exhibited a two-fold rise in expression levels when exposed to a deficiency of B. Other NIPs, likewise, NIP3.1 in rice and NIP2.1 in scarcely have also shown increases in expression [72]. B transport in participating, highlighting the importance of the protein medium in B transport and absorption. B transporters, such as BOR1, should also be engaged nether similar circumstances to accelerate B motion between root cells to the stele due to its opposing localizations with NIP on the plasma membrane, which encourages B motion. The transportation of B from symplast to apoplast is facilitated by a distinct transporter known as BOR2. This transporter is involved in the process of B release to ensure the growth of the cell wall in root cells (Table 1), which is of utmost importance to plant cells [73]. While aquaporins help in the transportation of B throughout plants, BOR1 promotes B absorption in roots. OsPIP2.4 and OsPIP2.7 aquaporins in rice contribute to the plant’s adaptation mechanisms against B stress by controlling water intake, preserving cellular homeostasis, and participating in B toxicity responses [74]. OsPIP2.4 and OsPIP2.7 are shown to be more abundant in rice, even though BOR1 normally degrades quickly in the presence of B toxicity to prevent excessive B delivery to the shoot.
The key to B tolerance starts from increased B outflow from the symplast to the apoplast, followed by B outflow from leaf hydathodes [74]. In the cells of the leaves and roots of scarce plants, efflux transporters were more strongly expressed. Additionally, it has been claimed that the tonoplast-located TIP aquaporin AtTIP5.1 contributes to B transport by effluxing it to the vacuole, increasing the B toxicity tolerance in A. thaliana. Finally, because of B’s importance to plants, a variety of aquaporins play a role in its homeostasis by conciliating the inflow and outflow mechanisms. It is tightly controlled to continue the adequate B translocation in plant cells and tissues for their normal functioning [75].
4 Drought Impact on Nutrient Transporters
The primary outcome of drought is a decrease in crop development and production, up to 90% [90]. Less cell turgor results from less water availability, which also inhibits cell elongation and promotes stomatal closure. This also reduces nutrient and water uptake as well as CO2 uptake and photosynthetic efficiency. The majority of the genes encoding nitrate transporters and the enzymes in charge of NO3− absorption and immobilization are de-regulated in reaction to drought stress [91]. However, it was discovered that within one hour of exposure to various abiotic stresses, the formulation of BjNRT1.1 and BjNRT1.5 was increased in Brassica juncea L. plants, showing an early reaction of N accretion on recognition of the initiation of stress. However, de-regulation occurred after 24 h, impacting the majority of N transporters, including BjNRT2.1. However, different plant species and subspecies within the same plant species have distinct management strategies for NRTs. However, an N-efficient variety maintained nearly consistent levels of NO3− transporter expression, including key sensors such as NRT1.1, NRT2.1, and NRT2.2, suggesting an enhanced capacity for NO3− consumption [92]. The high-octane N cultivar displayed a curiosity for AMT1.2 (NH4+ transporter) overexpression, suggesting its potential to detect changes in soil N forms and optimize resource utilization under drought conditions. Phosphate (Pi) absorption serves as an indicator of drought tolerance in plants [92]. It is hypothesized that differences in the composition of the PHT group of carriers are associated with Pi uptake, as demonstrated by individual instances like PtPHO9 and PtPHT1.2 in poplar, MdPHT1.5, MdPHT1.7, MdPHT4.7, MdPHT3.7, and MdPHT4.3 in apple. Interestingly, no genes were downregulated under drought stress in this context. Regarding K+ transporters, drought stress significantly influences the expression of the grapevine K+ transporter VvK1.1, which is analogous to the AKT1 channel in A. thaliana. Notably, VvK1.1 expression is pronounced in berries, particularly within the phloem vasculature, indicating its importance in facilitating K+ uptake into berry tissues, particularly under drought conditions. Furthermore, under drought stress conditions, there was an increase in the expression of CarAKT1,2, a potassium transporter responsible for K+ influx, and CarGORK, which plays a role in facilitating K+ efflux, in chickpeas. Although CarKAT1.1 and HvAKT1 are expressed less in chickpea and barley, respectively, this shows that the alteration in the transcript degree of a few AKT1 family elements may not be about drought tolerance. The reaction appears to be dependent on time- and genotype. The findings illustrate that precise management of K+ transporters is required to formulate a plan to counteract the stress of drought. In grapevines, drought stress increases the expression of VvK1.1, which promotes K+ build-up in berry tissues, which is essential for osmotic adjustment. Drought stress in chickpeas affects K+ uptake and efflux by inducing CarAKT1.2 and inhibiting CarGORK, exposing the intricate regulatory network of ion transporters in response to water constraints [93]. The Ca2+ is essential since it is a signaling molecule. Depending on the degree and length of the stress, transient Ca2+ peaks in the cytoplasm regulate the activity of several genes and proteins in plants in response [94]. As a result, depending on the tissues and specific tasks, the regulation of calcium transporters beneath drought stress varies substantially. Plant Ca2+ pumps play a role in removing Ca2+ from the cytosol. The expression of P-IIB Ca-ATPase PCA1 increased in Physcomitrella patens, B&S, in reaction to lack of water. Some self-inhibited calcium ATPases (ACAs) and ER-type ATPases (ECAs) pumps were found to respond to short-term dehydration stress in rice. ACA5 and ACA7 were significantly increased in roots and seedlings, meanwhile, ACA3, ACA5, and ACA7 were upregulated in mature leaves. During dehydration stress, the levels of BoACA4 and BoACA11 in levels of Brassica oleracea L. remained elevated for 24 h [19]. For the Ca2+ exchanger’s A. thaliana and rice CAX, transcript levels decreased in response to a water deficit. The transportation of B from the symplast to the apoplast is facilitated by a distinct transporter known as BOR2. This transporter accelerates the process of B release to ensure the growth of the cell wall in root cells, which is of utmost importance to plant cells [94]. Furthermore, B. oleracea BoCAX1 expression levels in leaf tissues steadily increased in response to drought stress [95]. Similar to how different cultivars of similar plant species or even different portions of the same tissue exhibit distinct drought tolerance strategies, distinct aquaporin homologs in different plant species also react differently to drought stress [96]. Different PIPs, TIPs, and NIPs homologs were up- and down-regulated in response to drought stress [97], according to root transcriptome analysis. Additionally, various homologs react differently depending on the degree and type of stress application. The aquaporins subgroup most susceptible to drought stress is PIPs, which results in a downregulation of their total transcription, particularly in roots. It was found that only a small subset of PIP genes, predominantly from the PIP1 subgroup, were upregulated in select tissues and during drought, especially in leaves. This increase of particular PIP1 subgroup isoforms in leaves suggests that they participate in gas and water exchange as well as stomatal behavior beneath stress [98].
5 Salinity and Its Effects on Nutrient Transporters
Salinity is among most of the prevalent abiotic stresses on plants around the globe. Around 800 million hectares (6%) of the world’s land area is impacted by salinity [99]. Saline soil is characterized by an osmotic pressure of approximately 0.2 MPa and an electrical conductivity (EC) greater than 4 dS m−1. The prime major crops are glycophytes, which are vulnerable to saline stress, and this stress causes considerable decreases in agricultural yield even at moderate environmental salt levels [100]. The salinization of soil has been a problem as a result of both natural and human-caused changes [101], and it is predicted that climate change will make it worse. As a result, there has been an increase in scientific investigation into the mechanisms underpinning plant’s ability to tolerate salt. The establishment of halophytic plants as fresh crops or the betterment of the primary crop species’ tolerance through breeding with or transferring genes from halophytes are only two of the many solutions to the salinity issue that have been identified. Two stages to the salt buildup in the soil cause plant stress: progressive ionic and nutritional stress is caused by the competition between ionic varieties of nutrients and saline ions (Na+, Cl−) and a decrease in water consumption, which induces rapid osmotic and water stress [102]. As a result, there have been modifications to the primary carbon and nitrogen metabolism in plant cells. The levels of several signaling molecules, the reduction of protein synthesis, and the change of water’s transmembrane mobility are all specifically impacted, as well as NO3− uptake and transport via roots [103]. There are three basic types of plant responses or tolerances for coping with this stress: (1) Osmotic stress tolerance, with the main response being a decrease in Lp and a suppression of root water uptake; (2) Na+ exception from leaf blades to prevent Na from building up to dangerous levels, and (3) intracellular Na compartmentalization to prevent toxic Na buildup in the cytoplasm.
The efficiency of these tolerance processes varies depending on the type of plant, being either halophytic or glycophytic. The impact of saline stress is directly connected to nutrient stress caused by inadequate absorption of necessary nutrients from the soil due to the excessive presence of Na+ and Cl–, leading to the hindrance of beneficial ion uptake [104]. The ratio of K+ to Na+ in inner metabolically dynamic tissue and a competent Ca2+ state, which is a component of the Na+ expulsion signaling procedure, play a crucial role in salt tolerance. To counteract salt stress, response, and tolerance mechanisms rely heavily on nutrient transporters. Extensive research has focused on the regulation of cellular ion homeostasis and salt tolerance is regulated through ion-specific transporters such as Na+ and K+. The genes for these transporters are either high- or low-regulated in response to salt stress, depending on the kind of plant or degree of resistance [105]. The plant’s reaction to this issue is just as crucial for developing salt tolerance, as salty stress can also disrupt the balance of other ions, such as Ca2+, Mg2+, or NO3−. Accordingly, numerous studies, mostly in A. thaliana, have established that the regulatory mechanisms of CAX transporters H+/Ca2+ antiporters to promote tolerance are finely tuned during exposure to salt stress [106]. Specifically, the response of CAX formulation to salinity is dependent on the isoform. The activity of OsHKT1.5 in the root autumnal region was notably enhanced due to the Mg2+ transported by OsMGT1, the Mg transporter. Because it prevents Na+ from building up in the shoots by keeping it out of the xylem, OsHKT1.5 is essential for this stress tolerance. Mg2+ may be needed for the stereo-composition of the OsHKT1.5 protein, even though the precise procedure of its activation is unrecognized. For the nitrate iron (NO3−), specific transporters known as NRT play a crucial role [107]. The altered NO3– absorption during saline stress will be caused by nitrate transporters, with a focus on NRT2, whose expression is mainly observed in the roots, as mentioned earlier. For instance, the NRT2.1 gene is de-regulated in plants with high salinity. Because NRT2.1 is intricated in encouraging lateral roots, a decrease in its mRNA expression may potentially have a detrimental effect on nitrate uptake when subjected to salt stress.
As mentioned earlier, salinity stress is closely linked to the plant’s water status; in particular, there is a rapid decrease in water transport rates. Thus, a water channel is essential for both the mechanisms of toleration and the response to this stress. Salinity modifies the expression of aquaporin genes and results in biochemical alterations in these proteins [108]. These adjustments are made to manage water transport and preserve water homeostasis. A broad pattern for aquaporin behavior under salt stress is difficult to pin down. Some studies make broad generalizations, such as the finding that PIP and TIP transcript quantities in A. thaliana drop by 60% to 75% over the means to endless following salt treatment (100 mM NaCl). The impact differs depending on the aquaporin type, stress duration or intensity, and plant and tissue tolerance [109]. PIP2.2 and PIP2.3 were increased in aerial portions following brief exposure to NaCl, but PIP2.6 was downregulated. PIP1.5, on the other hand, was downregulated in the roots of A. thaliana seedlings, whereas PIP1.1, PIP1.2, PIP1.3, and PIP2.7 were upregulated. The primary difference between this study and the one previously advertised was the age of the plants, which was 2 weeks in the first study and only 8 days in the second. The PIP2.7 formulations were suppressed in A. thaliana seedlings beneath salt stress. Similarly, it has been demonstrated that following 15 days of NaCl treatment, the expression of PIP1 and PIP2 was reduced in broccoli plant roots, despite an increase in PM levels of both proteins. Interestingly, the roots’ ability to transport water was compromised after prolonged exposure to saline stress for 15 days. To learn more about how these proteins respond to salinity, it is routine practice to overexpress several aquaporin genes. In this regard, numerous studies have demonstrated that transgenic plants with specific aquaporin genes overexpressed had greater salinity tolerance, such as PdPIP1.2 in date palms [110]. It has also been suggested that other aquaporin subfamilies, such as the NIP subfamily, contribute to salt tolerance. For example, TaNIP expression in winter wheat was enhanced in salinity, at the same time transgenic Arabidopsis plants overexpressing the TaNIP gene have excessive salt tolerance. Various mechanisms may be involved in the enhanced salt tolerance: the accretion of simpatico solutes to amend osmotic adjustment, the antioxidant defense system is strengthened, and K+/Na+ concentrations decreased while the K+/Na+ ratio is preserved [111]. Regardless of how salinity affects aquaporin gene formulation, this stress alters the function and status of aquaporins at the protein level. For instance, changes in the cytosolic pH and Ca2+ levels result in alterations to the aquaporins. Because of a pH-sensitive His residue that assists keep the aquaporins shut, salt-sensitive plants undergo a decrease in cytosolic pH, which results in aquaporins closure. The inhibition of aquaporins and the decrease in cytosolic Ca2+ brought on by salt stress. Specific nutrient transporters and aquaporins likely play a vital role in preserving cell homeostasis and improving salt tolerance in plants [112] given the necessity of nutrition and water transport for healthy plant development under saline stress. By suppressing the formulation of Na-specific transporter (SOS2), Arabidopsis transgenic plants were able to produce an abundance of salt-tolerant plants. A H+/Ca2+ antiporter, CAX1, may be negatively regulated by SOS2, which raises the possibility that NIP is mired in Ca2+ homeostasis, a salt stress reaction that regulates the cytosolic pH. Another example of this is the interaction between PIP aquaporins and NRT2.1 (a NO3− transporter) in A. thaliana. NO3− and NRT2.1 influence the alteration of Lp, exhibiting a positive relationship between Lp and NO3− concentration [113]. The regulation of PIP aquaporins plays a crucial role in water transport, as they are primarily responsible for altering Lp, thus forming a connection between PIPs and water transport. As we indicated before, NRT2.1 regulates aquaporins at the transcriptional and posttranscriptional stages, and it has been observed that roots exposed to salinity have decreased NRT2.1 abundance. As a result, PIP aquaporins may contain fewer molecules or function less effectively. A decline in PIP aquaporins function would lower Lp, a plant reaction to salt stress (Fig. 1). The existing literature and research on saline stress and plant responses suggest that the control of Lp when exposed to salinity may be influenced by both internal and external NO3− concentrations, as well as the actions of NRT2.1, via the regulation of PIP aquaporins [114].
Figure 1: Effects of salinity stress on nutrient transporters and their cross-talk with ion-gated channels
6 Temperature Effect on the Interaction of Nutrient Transporters
The major environmental aspect that hinders plant development, growth, and dispersion of vegetation is extreme heat, which is a significant abiotic stressor for plants [1]. Weather is characterized by extreme temperature events as a result of climate change will have an impact on agricultural production in the future. A drop in yield is a common consequence of abruptly low temperatures in agricultural areas nowadays. Understanding how temperature may affect how nutrients and water are transported by plants is essential for maximizing productivity (Fig. 2).
Figure 2: Effects of heat on nutrient transporters and the signaling for heat acclimation
The major significant nutrient whose transportation is influenced by down temperatures is N for a variety of reasons. First off, low temperatures alter the forms of N (NH4+ or NO3+) in soil [115]. Low temperatures render nitrifying bacteria inactive, which raises the NH4+/NO3+ ratio because NH4+ is less mobile. The ability of NH4+ to be absorbed via the excessive-affinity transport channel is also restricted at lower temperatures. Furthermore, an investigation into the bacterium B. juncea revealed that at reduced temperatures [116], the formulation of the prime NO3+ transporters and an NH4+ transporter (AMT2) decreases. A reduction in temperature may also alter intracellular transportation, such as by increasing the expression of the vacuolar transporter CAX1, resulting in an elevation of cytosolic-free Ca2+. It has been reported that the uptake of micronutrients is influenced by low temperatures due to decreased expression of their transporters. This was observed with Mn in A. thaliana using the AtNRAMP1 transporter. Sweet pepper, has a reduction in Fe and Zn as a result of low temperatures [117]; even though there are numerous studies on the concentrations of micronutrients in plants exposed to low temperatures, there are none on their transporters. Additionally, the hydraulic conductivity of the leaves and roots is significantly altered by low temperatures; this property is governed directly by aquaporins. Recent research has examined the utilization of aquaporins to enhance resistance to low temperatures through overexpression, exemplified by the overexpression of a wheat PIP2 gene (TaAQP7) in tobacco plants, or overexpressing an aquaporin gene PIP2.7 in tobacco and banana. This finding underscores the significance of PIP2.7 in the context of cool stress response. An alternative investigation about rice plants posits that the reduced water absorption observed during frigid temperatures might be predominantly attributable to the inhibition of aquaporin activity, as opposed to an accumulation of aquaporin in the root membrane. An additional crucial factor seems to be the post-translational regulation of aquaporins. However, there is uncertainty regarding the interplay between aquaporins, other nutrient transporters, and abiotic stresses such as high temperatures. This is true even though aquaporins can transport some components. Additionally, plants often produce reactive oxygen species such as H2O2 and reduce nutrient delivery in response to high temperatures [118]. Recent, groundbreaking research has focused on the interaction between aquaporins and various nutrient transporters under conditions of low-temperature stress. One study suggests that the calcium-dependent protein kinase OsCPK17 may regulate aquaporins in response to cold stress. Aquaporins may therefore be associated with an increase in the formulation of the Ca2+ transporter-like CAX1 in vacuoles [119]. In another instance, the B remained constant despite a reduction of BOR1 protein (a B transporter), whereas NIP5.1 levels stayed consistent. This finding implies that the B order was maintained despite the stress due to the aquaporin’s stability. The P deficiency that is normally caused by cold temperatures can be remunerated by increasing the Si dispersion, which may be carried by some aquaporins as silicic acid. This was demonstrated in studies utilizing tomatoes. Previous research has shown that the transportation of Si in the form of silicic acid, along with Se, can lead to a reduction in As uptake due to competitive inhibition. The presence of Si and Se can alleviate the toxicity of As by utilizing the same transporter, Lsi1, which is an aquaporin [120].
For plants to effectively adapt to environmental stresses such as nutrient deficiency, drought, high salinity, and low temperatures, it is necessary to regulate the expression of genes responsible for nutrient transporters and aquaporins. The primary stimulus that triggers subsequent responses must thus be related to water, notwithstanding the need for more investigation into the interactions of both kinds of transporters. To understand the synchronized physiological reactions, it is important to take into account the role of aquaporins in the first detection of stress. The results outlined in this analysis emphasize many common responses. For example, a deficiency in each macro-nutrient enhances the activity of its transporter but reduces the activity of aquaporins in the plasma membrane. The absence of micronutrients leads to the relocation of tonoplast transporters from the vacuole and a reduction in the abundance of aquaporins. Furthermore, it is crucial to take into account the data indicating that some aquaporins transport essential minerals such as NH4+, B, and urea to ensure the availability of nutrients under abiotic stress. The response of plants to salt and drought depends on the unique mechanism and amount of tolerance of each species. However, it can be generally observed that plants with drought tolerance tend to have increased expression of transporters and aquaporins. The intricate nature of salinity in all its facets of stress highlights the significance of several transport channels in terms of tolerance, namely reducing Na absorption while enhancing K and N intake. Aquaporins have also been shown to be essential for the transportation of water and the appropriate growth of plants in the presence of high salt levels. Hence, it is crucial to examine the collaborative functioning of nutrient transporters and aquaporins to maintain cellular homeostasis under various circumstances. Under low-temperature stress, there has been a significant decrease in the expression of aquaporin. However, it is noteworthy that PIP2.5 or PIP2.7 was consistently shown to be raised in all the studies, indicating their important role in the response. By understanding the interactions between aquaporins and mineral nutrients under environmental stress, we may enhance the efficiency of water and nutrient utilization in plants by regulating water and mineral nutrient absorption and assimilation. Further investigation is necessary to better understand the signaling and regulation of aquaporins and nutrient transport together since only modifying their combined response would enhance tolerance.
Acknowledgement: Not applicable.
Funding Statement: This research was supported by the Natural Science Foundation of Jiangsu Higher Education Institutions of China (23KJA210003), the Open Project Program of Joint International Research Laboratory of Agriculture and Agri-Product Safety, the Ministry of Education of China, Yangzhou University (JILAR-KF202202).
Author Contributions: Muhammad Salee: writing-original draft; Jianhua Zhang, Rashid Iqbal, and Muhammad Qasim: review and editing; Li Song: conceptualization, funding, and supervision. All authors reviewed the results and approved the final version of the manuscript.
Availability of Data and Materials: Not applicable.
Ethics Approval: Not applicable.
Conflicts of Interest: The authors declare that they have no conflicts of interest to report regarding the present study.
References
1. Fadiji AE, Yadav AN, Santoyo G, Babalola OO. Understanding the plant-microbe interactions in environments exposed to abiotic stresses: an overview. Microbiol Res. 2023;(271):127368. [Google Scholar]
2. Riyazuddin R, Singh K, Iqbal N, Nisha N, Rani A, Kumar M, et al. Iodine: an emerging biostimulant of growth and stress responses in plants. Plant Soil. 2022;486:119–33. [Google Scholar]
3. Yang X, Lu M, Wang Y, Wang Y, Liu Z, Chen S. Response mechanism of plants to drought stress. Horticulturae. 2021;7(3):50. doi:10.3390/horticulturae7030050. [Google Scholar] [CrossRef]
4. Zhang T, Yu S, Pan Y, Li H, Liu X, Cao J. Properties of texturized protein and performance of different protein sources in the extrusion process: a review. Food Res Int. 2023;174:113588. doi:10.1016/j.foodres.2023.113588. [Google Scholar] [PubMed] [CrossRef]
5. Ullah A, Zhao C, Zhang M, Sun C, Liu X, Hu J, et al. Nitrogen enhances the effect of pre-drought priming against post-anthesis drought stress by regulating starch and protein formation in wheat. Physiol Plant. 2023;175(2):e13907. doi:10.1111/ppl.v175.2. [Google Scholar] [CrossRef]
6. Austin S, Mayer A. Phosphate homeostasis-a vital metabolic equilibrium maintained through the INPHORS signaling pathway. Front Microbiol. 2020;11:1367. doi:10.3389/fmicb.2020.01367. [Google Scholar] [PubMed] [CrossRef]
7. Raza A, Tabassum J, Mubarik M, Anwar S, Zahra N, Sharif Y, et al. Hydrogen sulfide: an emerging component against abiotic stress in plants. Plant Biol. 2022;24(4):540–58. doi:10.1111/plb.13368. [Google Scholar] [PubMed] [CrossRef]
8. Huang J, Wang C, Qi L, Zhang X, Tang G, Li L, et al. Phosphorus is more effective than nitrogen in restoring plant communities of heavy metals polluted soils. Environ Pollut. 2020;266:115259. doi:10.1016/j.envpol.2020.115259. [Google Scholar] [PubMed] [CrossRef]
9. Tewari RK, Yadav N, Gupta R, Kumar P. Oxidative stress under macronutrient deficiency in plants. J Soil Sci Plant Nutr. 2021;21(1):832–59. doi:10.1007/s42729-020-00405-9. [Google Scholar] [CrossRef]
10. Godoy F, Olivos-Hernández K, Stange C, Handford M. Abiotic stress in crop species: improving tolerance by applying plant metabolites. Plants. 2021;10(2):186. doi:10.3390/plants10020186. [Google Scholar] [PubMed] [CrossRef]
11. Shahid MA, Sarkhosh A, Khan N, Balal RM, Ali S, Rossi L, et al. Insights into the physiological and biochemical impacts of salt stress on plant growth and development. Agronomy. 2020;10(7):938. doi:10.3390/agronomy10070938. [Google Scholar] [CrossRef]
12. Bekele D, Birhan M. The impact of secondary macro nutrients on crop production. Int J Res Stud. 2021;7(5):37–51. [Google Scholar]
13. Fan X, Zhou X, Chen H, Tang M, Xie X. Cross-talks between macro- and micronutrient uptake and signaling in plants. Front Plant Sci. 2021;12:663477. doi:10.3389/fpls.2021.663477. [Google Scholar] [PubMed] [CrossRef]
14. Shilpha J, Song J, Jeong B. Ammonium phytotoxicity and tolerance: an insight into ammonium nutrition to improve crop productivity. Agronomy. 2023;13(6):1487. doi:10.3390/agronomy13061487. [Google Scholar] [CrossRef]
15. Lizama-Gasca MG, Estrada-Tapia G, Escalante-Magaña CA, Martínez-Estévez M, Zepeda-Jazo I, Medina-Lara F, et al. Cloning and molecular characterization of CcNRT2.1/CcNAR2, a putative inducible high affinity nitrate transport system in Capsicum chinense Jacq. Roots. Trop Plant Biol. 2020;13:73–90. doi:10.1007/s12042-019-09248-w. [Google Scholar] [CrossRef]
16. Shi X, Cui F, Han X, He Y, Zhao L, Zhang N, et al. Comparative genomic and transcriptomic analyses uncover the molecular basis of high nitrogen-use efficiency in the wheat cultivar Kenong 9204. Mol Plant. 2022;15(9):1440–56. doi:10.1016/j.molp.2022.07.008. [Google Scholar] [PubMed] [CrossRef]
17. de Bang TC, Husted S, Laursen KH, Persson DP, Schjoerring JK. The molecular-physiological functions of mineral macronutrients and their consequences for deficiency symptoms in plants. New Phytol. 2021;229(5):2446–69. doi:10.1111/nph.17074. [Google Scholar] [PubMed] [CrossRef]
18. Rahman A, Kawamura Y, Maeshima M, Rahman A, Uemura M. Plasma membrane aquaporin members PIPs act in concert to regulate cold acclimation and freezing tolerance responses in Arabidopsis thaliana. Plant Cell Physiol. 2020;61(4):787–802. doi:10.1093/pcp/pcaa005. [Google Scholar] [PubMed] [CrossRef]
19. Barzana G, Rios JJ, Lopez-Zaplana A, Nicolas-Espinosa J, Yepes-Molina L, Garcia-Ibañez P, et al. Interrelations of nutrient and water transporters in plants under abiotic stress. Physiol Plant. 2021;171(4):595–619. doi:10.1111/ppl.13206. [Google Scholar] [PubMed] [CrossRef]
20. Kaur H, Garg N. Zinc toxicity in plants: a review. Planta. 2021;253(6):129. doi:10.1007/s00425-021-03642-z. [Google Scholar] [PubMed] [CrossRef]
21. Sudhakaran S, Thakral V, Padalkar G, Rajora N, Dhiman P, Raturi G, et al. Significance of solute specificity, expression, and gating mechanism of tonoplast intrinsic protein during development and stress response in plants. Physiol Plant. 2021;172(1):258–74. doi:10.1111/ppl.v172.1. [Google Scholar] [CrossRef]
22. Brownlee C, Helliwell KE, Meeda Y, McLachlan D, Murphy EA, Wheeler GL, et al. Regulation and integration of membrane transport in marine diatoms. Semin Cell Dev Biol. 2023;134:79–89. doi:10.1016/j.semcdb.2022.03.006. [Google Scholar] [PubMed] [CrossRef]
23. He MY, Ren TX, Jin ZD, Deng L, Liu HJ, Cheng YY, et al. Precise analysis of potassium isotopic composition in plant materials by multi-collector inductively coupled plasma mass spectrometry. Spectrochim Acta Part B At Spectrosc. 2023;209:106781. doi:10.1016/j.sab.2023.106781. [Google Scholar] [CrossRef]
24. Sharma P, Ngo HH, Khanal S, Larroche C, Kim SH, Pandey A. Efficiency of transporter genes and proteins in hyperaccumulator plants for metals tolerance in wastewater treatment: sustainable technique for metal detoxification. Environ Technol Inno. 2021;23:101725. doi:10.1016/j.eti.2021.101725. [Google Scholar] [CrossRef]
25. Khan MIR, Palakolanu SR, Chopra P, Rajurkar AB, Gupta R, Iqbal N, et al. Improving drought tolerance in rice: ensuring food security through multi-dimensional approaches. Physiol Plant. 2021;172(2):645–68. doi:10.1111/ppl.v172.2. [Google Scholar] [CrossRef]
26. Gao P, Li M, Wang X, Xu Z, Wu K, Sun Q, et al. Identification of elite R-gene combinations against blast disease in Geng rice varieties. Int J Mol Sci. 2023;24(4):3984. doi:10.3390/ijms24043984. [Google Scholar] [PubMed] [CrossRef]
27. Groszmann M, de Rosa A, Chen W, Qiu J, McGaughey SA, Byrt CS, et al. A high-throughput yeast approach to characterize aquaporin permeabilities: profiling the Arabidopsis PIP aquaporin sub-family. Front Plant Sci. 2023;14:1078220. doi:10.3389/fpls.2023.1078220. [Google Scholar] [PubMed] [CrossRef]
28. Ragel P, Raddatz N, Leidi EO, Quintero FJ, Pardo JM. Regulation of K+ nutrition in plants. Front Plant Sci. 2019;10:281. doi:10.3389/fpls.2019.00281. [Google Scholar] [PubMed] [CrossRef]
29. Xiong L. Tailoring hydroxyapatite (HA) nanoparticles as a phosphorus (P) fertiliser in soils (Ph.D. Thesis). The University of Queensland: Australia; 2019. [Google Scholar]
30. Dissanayaka D, Ghahremani M, Siebers M, Wasaki J, Plaxton WC. Recent insights into the metabolic adaptations of phosphorus-deprived plants. J Exp Bot. 2021;72(2):199–223. doi:10.1093/jxb/eraa482. [Google Scholar] [PubMed] [CrossRef]
31. Peng Z, Tian J, Luo R, Kang Y, Lu Y, Hu Y, et al. MiR399d and epigenetic modification comodulate anthocyanin accumulation in Malus leaves suffering from phosphorus deficiency. Plant Cell Environ. 2020;43(5):1148–59. doi:10.1111/pce.v43.5. [Google Scholar] [CrossRef]
32. Armand T, Cullen M, Boiziot F, Li L, Fricke W. Cortex cell hydraulic conductivity, endodermal apoplastic barriers and root hydraulics change in barley (Hordeum vulgare L.) in response to a low supply of N and P. Ann Bot. 2019;124(6):1091–107. [Google Scholar] [PubMed]
33. Li L, Pan S, Melzer R, Fricke W. Apoplastic barriers, aquaporin gene expression and root and cell hydraulic conductivity in phosphate-limited sheepgrass plants. Physiol Plant. 2020;168(1):118–32. doi:10.1111/ppl.v168.1. [Google Scholar] [CrossRef]
34. Ferrari M, Cozza R, Marieschi M, Torelli A. Role of sulfate transporters in chromium tolerance in Scenedesmus acutus M. (Sphaeropleales). Plants. 2022;11(2):223. doi:10.3390/plants11020223. [Google Scholar] [PubMed] [CrossRef]
35. Quiroga G, Erice G, Ding L, Chaumont F, Aroca R, Ruiz-Lozano JM. The arbuscular mycorrhizal symbiosis regulates aquaporins activity and improves root cell water permeability in maize plants subjected to water stress. Plant Cell Environ. 2019;42(7):2274–90. doi:10.1111/pce.v42.7. [Google Scholar] [CrossRef]
36. Xu X, Ren Y, Wang C, Zhang H, Wang F, Chen J, et al. OsVIN2 encodes a vacuolar acid invertase that affects grain size by altering sugar metabolism in rice. Plant Cell Rep. 2019;38:1273–90. doi:10.1007/s00299-019-02443-9. [Google Scholar] [PubMed] [CrossRef]
37. Nie S, Mo S, Gao T, Yan B, Shen P, Kashif M, et al. Coupling effects of nitrate reduction and sulfur oxidation in a subtropical marine mangrove ecosystem with Spartina alterniflora invasion. Sci Total Environ. 2023;862:160930. doi:10.1016/j.scitotenv.2022.160930. [Google Scholar] [PubMed] [CrossRef]
38. Kim N, Watmough SA, Yan ND. Wood ash amendments as a potential solution to widespread calcium decline in eastern Canadian forests. Environ Rev. 2022;30(4):485–500. doi:10.1139/er-2022-0017. [Google Scholar] [CrossRef]
39. Zhang T, Li Y, Li H, Zhang S, Zhou Y. Optimal soil calcium for the growth of mulberry seedlings is altered by nitrogen addition. Forests. 2023;14(2):399. doi:10.3390/f14020399. [Google Scholar] [CrossRef]
40. Qi Z, Li L, Xu C, Liu M, Wang Y, Zhang L, et al. The sodium/calcium exchanger PcNCX1-mediated Ca2+ efflux is involved in Cinnamaldehyde-induced cell-wall defects of phytophthora capsici. Agronomy. 2022;12(8):1763. doi:10.3390/agronomy12081763. [Google Scholar] [CrossRef]
41. Barati B, Zeng K, Baeyens J, Wang S, Addy M, Gan SY, et al. Recent progress in genetically modified microalgae for enhanced carbon dioxide sequestration. Biomass Bioenergy. 2021;145:105927. doi:10.1016/j.biombioe.2020.105927. [Google Scholar] [CrossRef]
42. Li H, Testerink C, Zhang Y. How roots and shoots communicate through stressful times. Trends Plant Sci. 2021;26(9):940–52. doi:10.1016/j.tplants.2021.03.005. [Google Scholar] [PubMed] [CrossRef]
43. Vens CS. Calmodulins and their confederates drive defense and touch responses in Arabidopsis Thaliana (Ph.D. Thesis). The University of Wisconsin-Madison: USA; 2019. [Google Scholar]
44. Tuomivaara ST, Teo CF, Jan YN, Jan LY, Wiita AP. SLAPSHOT reveals rapid dynamics of extracellularly exposed proteome in response to calcium-activated plasma membrane phospholipid scrambling. bioRxiv. 2023. doi:10.1101/2023.03.26.534250. [Google Scholar] [CrossRef]
45. Ramírez-Builes VH, Küsters J, de Souza TR, Simmes C. Calcium nutrition in coffee and its influence on growth, stress tolerance, cations uptake, and productivity. Front Agron. 2020;2:590892. doi:10.3389/fagro.2020.590892. [Google Scholar] [CrossRef]
46. Ishijima S. Magnesium and Mg2+ transport proteins in cells magnesium and Mg2+ transport proteins in cells. Int J Biol Macromol. 2021;21(2):55–67. doi:10.14533/jbm.21.55. [Google Scholar] [CrossRef]
47. Devi MJ, Reddy VR. Stomatal closure response to soil drying at different vapor pressure deficit conditions in maize. Plant Physiol Biochem. 2020;154:714–22. doi:10.1016/j.plaphy.2020.07.023. [Google Scholar] [PubMed] [CrossRef]
48. Michael PS. Role of organic fertilizers in the management of nutrient deficiency, acidity, and toxicity in acid soils-a review. J Global Agric Ecol. 2021;12(3):19–30. [Google Scholar]
49. Gupta N, Ram H, Cakmak I. Soil to seed. In: Kumar S, Dikshit HK, Mishra GP, Singh A, editors. Biofortification of staple crops. Singapore: Springer Singapore; 2022. p. 519–49. [Google Scholar]
50. Schiavon M, Nardi S, Dalla Vecchia F, Ertani A. Selenium biofortification in the 21st century: status and challenges for healthy human nutrition. Plant Soil. 2020;453(1–2):245–70. doi:10.1007/s11104-020-04635-9. [Google Scholar] [PubMed] [CrossRef]
51. Liu CA, Liang MY, Nie Y, Tang JW, Siddique KH. The conversion of tropical forests to rubber plantations accelerates soil acidification and changes the distribution of soil metal ions in topsoil layers. Sci Total Environ. 2019;696(1–3):134082. doi:10.1016/j.scitotenv.2019.134082. [Google Scholar] [CrossRef]
52. de Souza SB, Bertolazi AA, Eutrópio FJ, Dutra AM, Brandão AB, Lopes JVSR, et al. Iron toxicity and its relation to nitrogen and phosphorus availability in ectomycorrhizal fungi. In: Cruz C, Vishwakarma K, Choudhary DK, Varma A, editors. Soil nitrogen ecology. Cham: Springer; 2021. p. 459–79. [Google Scholar]
53. Martín-Esquinas A, Hernández-Apaolaza L. Rice responses to silicon addition at different Fe status and growth pH. Evaluation of ploidy changes. Plant Physiol Biochem. 2021;163(13):296–307. doi:10.1016/j.plaphy.2021.04.012. [Google Scholar] [PubMed] [CrossRef]
54. Upadhyay N, Kar D, Deepak Mahajan B, Nanda S, Rahiman R, Panchakshari N, et al. The multitasking abilities of MATE transporters in plants. J Exp Bot. 2019;70(18):4643–56. doi:10.1093/jxb/erz246. [Google Scholar] [PubMed] [CrossRef]
55. Nile SH, Thiruvengadam M, Wang Y, Samynathan R, Shariati MA, Rebezov M, et al. Nano-priming as emerging seed priming technology for sustainable agriculture-recent developments and future perspectives. J Nanobiotechnol. 2022;20(1):1–31. doi:10.1186/s12951-022-01423-8. [Google Scholar] [PubMed] [CrossRef]
56. Faria JM, Teixeira DM, Pinto AP, Brito I, Barrulas P, Carvalho M. Aluminium, iron and silicon subcellular redistribution in wheat induced by manganese toxicity. Appl Sci. 2021;11(18):8745. doi:10.3390/app11188745. [Google Scholar] [CrossRef]
57. Shulga N, Abramov S, Klyukina A, Ryazantsev K, Gavrilov S. Fast-growing Arctic Fe-Mn deposits from the Kara Sea as the refuges for cosmopolitan marine microorganisms. Sci Rep. 2022;12(1):21967. doi:10.1038/s41598-022-23449-6. [Google Scholar] [PubMed] [CrossRef]
58. Surgun-Acar Y, Zemheri-Navruz F. Exogenous application of 24-epibrassinolide improves manganese tolerance in Arabidopsis thaliana L. via the modulation of antioxidant system. J Plant Growth Regul. 2022;41(2):546–57. doi:10.1007/s00344-021-10320-7. [Google Scholar] [CrossRef]
59. Chen X, Zhao Y, Zhong Y, Chen J, Qi X. Deciphering the functional roles of transporter proteins in subcellular metal transportation of plants. Planta. 2023;258(1):17. doi:10.1007/s00425-023-04170-8. [Google Scholar] [PubMed] [CrossRef]
60. Lutsenko S. Dynamic and cell-specific transport networks for intracellular copper ions. J Cell Sci. 2021;134(21):jcs240523. doi:10.1242/jcs.240523. [Google Scholar] [PubMed] [CrossRef]
61. Chen X, Yang S, Ma J, Huang Y, Wang Y, Zeng J, et al. Manganese and copper additions differently reduced cadmium uptake and accumulation in dwarf Polish wheat (Triticum polonicum L.). J Hazard. 2023;448:130998. doi:10.1016/j.jhazmat.2023.130998. [Google Scholar] [PubMed] [CrossRef]
62. Hunter CR. A new woody perspective on copper homeostasis: systemic copper transport and distribution, Effect of copper on lignification, and water transport in hybrid poplar (Ph.D. Thesis). Colorado State University: USA; 2022. [Google Scholar]
63. Javornik T, Poljak M, Carović-stanko K, Lazarević B. Common bean (Phaseolus vulgaris L.) gas exchange capacity under nutrient deficiency. J Cent Eur Agric. 2023;24(1):216–24. doi:10.5513/JCEA01/24.1.3667. [Google Scholar] [CrossRef]
64. Tyerman SD, McGaughey SA, Qiu J, Yool AJ, Byrt CS. Adaptable and multifunctional ion-conducting aquaporins. Annu Rev Plant Biol. 2021;72:703–36. doi:10.1146/arplant.2021.72.issue-1. [Google Scholar] [CrossRef]
65. Setiawan GD, Treesubsuntorn C, Krobthong S, Yingchutrakul Y, Thiravetyan P. Using multi-omics approach to investigate the effect of a moon soil simulant on Vigna radiata seedling root and shoot growth, stress responses, and photosynthesis. Acta Astronaut. 2023;202:550–63. doi:10.1016/j.actaastro.2022.11.022. [Google Scholar] [CrossRef]
66. Dhaliwal SS, Sharma V, Shukla AK. Impact of micronutrients in mitigation of abiotic stresses in soils and plants-A progressive step toward crop security and nutritional quality. Adv Agron. 2022;173:1–78. doi:10.1016/bs.agron.2022.02.001. [Google Scholar] [CrossRef]
67. Gong F, Qi T, Hu Y, Jin Y, Liu J, Wang W, et al. Genome-wide investigation and functional verification of the ZIP family transporters in wild emmer wheat. Int J Mol Sci. 2022;23(5):2866. doi:10.3390/ijms23052866. [Google Scholar] [PubMed] [CrossRef]
68. Narayanan M, Ma Y. Metal tolerance mechanisms in plants and microbe-mediated bioremediation. Environ Res. 2023;222:115413. doi:10.1016/j.envres.2023.115413. [Google Scholar] [PubMed] [CrossRef]
69. Meng SF, Zhang B, Tang RJ, Zheng XJ, Chen R, Liu CG, et al. Four plasma membrane-localized MGR transporters mediate xylem Mg2+ loading for root-to-shoot Mg2+ translocation in Arabidopsis. Mol Plant. 2022;15(5):805–19. doi:10.1016/j.molp.2022.01.011. [Google Scholar] [PubMed] [CrossRef]
70. Nejad SAG, Etesami H. The importance of boron in plant nutrition. In: Deshmukh R, Durgesh KT, Guerriero G, editors. Metalloids in plants: Advances and future prospects. Canada: John Wiley & Sons, Ltd.; 2020. p. 433–49. [Google Scholar]
71. Brodribb T, Brodersen CR, Carriqui M, Tonet V, Rodriguez Dominguez C, McAdam S. Linking xylem network failure with leaf tissue death. New Phytol. 2021;232(1):68–79. doi:10.1111/nph.v232.1. [Google Scholar] [CrossRef]
72. Younas MU, Ahmad I, Qasim M, Ijaz Z, Rajput N, Parveen Memon S, et al. Progress in the management of rice blast disease: the role of avirulence and resistance genes through gene-for-gene interactions. Agronomy. 2024;14(1):163. doi:10.3390/agronomy14010163. [Google Scholar] [CrossRef]
73. Abou Seeda M, Abou El-Nour EZ, Abdallah M, El-Bassiouny H. Impacts of metal, metalloid and their effects in plant physiology: a review. Middle East J Agric Res. 2022;11(3):838–931. [Google Scholar]
74. Filipović A. Water plant and soil relation under stress situations. In: Meena RS, Datta R, editors. Soil moisture importance. London: Intechopen; 2021. [Google Scholar]
75. Pang Y, Li L, Ren F, Lu P, Wei P, Cai J, et al. Overexpression of the tonoplast aquaporin AtTIP5;1 conferred tolerance to boron toxicity in Arabidopsis. J Genet Genomics. 2010;37(6):389–97. doi:10.1016/S1673-8527(09)60057-6. [Google Scholar] [PubMed] [CrossRef]
76. Nadeem F, Hanif MA, Majeed MI, Mushtaq Z. Role of macronutrients and micronutrients in the growth and development of plants and prevention of deleterious plant diseases-a comprehensive review. Int J Chem Biochem. 2018;14:1–22. [Google Scholar]
77. Riaz M, Kamran M, El-Esawi MA, Hussain S, Wang X. Boron-toxicity induced changes in cell wall components, boron forms, and antioxidant defense system in rice seedlings. Ecotoxicol Environ Saf. 2021;216:112192. doi:10.1016/j.ecoenv.2021.112192. [Google Scholar] [PubMed] [CrossRef]
78. Martins V, Garcia A, Alhinho AT, Costa P, Lanceros-Méndez S, Costa MMR, et al. Vineyard calcium sprays induce changes in grape berry skin, firmness, cell wall composition and expression of cell wall-related genes. Plant Physiol Biochem. 2020;150:49–55. doi:10.1016/j.plaphy.2020.02.033. [Google Scholar] [PubMed] [CrossRef]
79. Guo R, Ebenezer V, Wang H, Ki JS. Chlorine affects photosystem II and modulates the transcriptional levels of photosynthesis-related genes in the dinoflagellate Prorocentrum minimum. J Appl Phycol. 2017;29(1):153–63. doi:10.1007/s10811-016-0955-8. [Google Scholar] [CrossRef]
80. Yruela I. Copper in plants: acquisition, transport and interactions. Funct Plant Biol. 2009;36(5):409–30. doi:10.1071/FP08288. [Google Scholar] [PubMed] [CrossRef]
81. Li J, Cao X, Jia X, Liu L, Cao H, Qin W, et al. Iron deficiency leads to chlorosis through impacting chlorophyll synthesis and nitrogen metabolism in Areca catechu L. Front Plant Sci. 2021;12:710093. doi:10.3389/fpls.2021.710093. [Google Scholar] [PubMed] [CrossRef]
82. Wang Z, Hong X, Hu K, Wang Y, Wang X, Du S, et al. Impaired magnesium protoporphyrin IX methyltransferase (ChlM) impedes chlorophyll synthesis and plant growth in rice. Front Plant Sci. 2017;8:289556. doi:10.3389/fpls.2017.01694. [Google Scholar] [PubMed] [CrossRef]
83. Schmidt SB, Husted S. The biochemical properties of manganese in plants. Plants. 2019;8(10):381. doi:10.3390/plants8100381. [Google Scholar] [PubMed] [CrossRef]
84. Li M, Zhang P, Guo Z, Cao W, Gao L, Li Y, et al. Molybdenum nanofertilizer boosts biological nitrogen fixation and yield of soybean through delaying nodule senescence and nutrition enhancement. ACS Nano. 2023;17(15):14761–74. doi:10.1021/acsnano.3c02783. [Google Scholar] [PubMed] [CrossRef]
85. Biancarosa I, Espe M, Bruckner C, Heesch S, Liland N, Waagbø R, et al. Amino acid composition, protein content, and nitrogen-to-protein conversion factors of 21 seaweed species from Norwegian waters. J Appl Phycol. 2017;29(2):1001–9. doi:10.1007/s10811-016-0984-3. [Google Scholar] [CrossRef]
86. Khan F, Siddique AB, Shabala S, Zhou M, Zhao C. Phosphorus plays key roles in regulating plants’ physiological responses to abiotic stresses. Plants. 2023;12(15):2861. doi:10.3390/plants12152861. [Google Scholar] [PubMed] [CrossRef]
87. Wang Y, Wu WH. Regulation of potassium transport and signaling in plants. Curr Opin Plant Biol. 2017;39:123–8. doi:10.1016/j.pbi.2017.06.006. [Google Scholar] [PubMed] [CrossRef]
88. Narayan OP, Kumar P, Yadav B, Dua M, Johri AK. Sulfur nutrition and its role in plant growth and development. Plant Signal Behav. 2023;18(1):2030082. doi:10.1080/15592324.2022.2030082. [Google Scholar] [PubMed] [CrossRef]
89. Hara T, Takeda TA, Takagishi T, Fukue K, Kambe T, Fukada T. Physiological roles of zinc transporters: molecular and genetic importance in zinc homeostasis. J Physiol Sci. 2017;67(2):283–301. doi:10.1007/s12576-017-0521-4. [Google Scholar] [PubMed] [CrossRef]
90. Camaille M, Fabre N, Clément C, Ait Barka E. Advances in wheat physiology in response to drought and the role of plant growth promoting rhizobacteria to trigger drought tolerance. Microorganisms. 2021;9(4):687. doi:10.3390/microorganisms9040687. [Google Scholar] [PubMed] [CrossRef]
91. Quintana J, Bernal M, Scholle M, Holländer-Czytko H, Nguyen NT, Piotrowski M, et al. Root-to-shoot iron partitioning in Arabidopsis requires IRON-REGULATED TRANSPORTER1 (IRT1) protein but not its iron (II) transport function. Plant J. 2022;109(4):992–1013. doi:10.1111/tpj.15611. [Google Scholar] [PubMed] [CrossRef]
92. Ninkuu V, Liu Z, Sun X. Genetic regulation of nitrogen use efficiency in Gossypium spp. Plant Cell Environ. 2023;46(6):1749–73. doi:10.1111/pce.14586. [Google Scholar] [PubMed] [CrossRef]
93. Siddique MH, Babar NI, Zameer R, Muzammil S, Nahid N, Ijaz U, et al. Genome-wide identification, genomic organization, and characterization of potassium transport-related genes in Cajanus cajan and their role in abiotic stress. Plants. 2021;10(11):2238. doi:10.3390/plants10112238. [Google Scholar] [PubMed] [CrossRef]
94. Aslam M, Fakher B, Ashraf MA, Cheng Y, Wang B, Qin Y. Plant low-temperature stress: signaling and response. Agronomy. 2022;12(3):702. doi:10.3390/agronomy12030702. [Google Scholar] [CrossRef]
95. Bárzana G, Carvajal M. Genetic regulation of water and nutrient transport in water stress tolerance in roots. J Biotechnol. 2020;324:134–42. doi:10.1016/j.jbiotec.2020.10.003. [Google Scholar] [PubMed] [CrossRef]
96. Zupin M, Sedlar A, Kidrič M, Meglič V. Drought-induced expression of aquaporin genes in leaves of two common bean cultivars differing in tolerance to drought stress. J Plant Res. 2017;130(4):735–45. doi:10.1007/s10265-017-0920-x. [Google Scholar] [PubMed] [CrossRef]
97. Wei Q, Ma Q, Ma Z, Zhou G, Feng F, Le S, et al. Genome-wide identification and characterization of sweet orange (Citrus sinensis) aquaporin genes and their expression in two citrus cultivars differing in drought tolerance. Tree Genet Genomes. 2019;15:1–13. [Google Scholar]
98. Israel D. The ecophysiology of plasma membrane aquaporins in Arabidopsis thaliana and Fagus sylvatica (Ph.D. Thesis). University of Helsinki: Finland; 2023. [Google Scholar]
99. Hopmans JW, Qureshi A, Kisekka I, Munns R, Grattan S, Rengasamy P, et al. Critical knowledge gaps and research priorities in global soil salinity. In: Sparks DL, editor. Advances in agronomy. Newark: Academic Press, Elsevier; 2021. p. 1–191. [Google Scholar]
100. Qin X, Zhang K, Fan Y, Fang H, Nie Y, Wu XL. The bacterial MtrAB two-component system regulates the cell wall homeostasis responding to environmental alkaline stress. Microbiol. 2022;10(5):e02311–22. [Google Scholar]
101. Abedin MA, Hosenuzzaman M. Change in cropping pattern and soil health in relation to climate change and salinity in coastal Bangladesh. In: Pal I, Shaw R, editors. Multi-hazard vulnerability and resilience building. Newark: Elsevier; 2023. p. 315–32. [Google Scholar]
102. Calone R, Mircea DM, González-Orenga S, Boscaiu M, Lambertini C, Barbanti L, et al. Recovery from salinity and drought stress in the perennial Sarcocornia fruticosa vs. the annual Salicornia europaea and S. veneta. Plants. 2022;11(8):1058. doi:10.3390/plants11081058. [Google Scholar] [PubMed] [CrossRef]
103. Kohli SK, Khanna K, Bhardwaj R, Abd_Allah EF, Ahmad P, Corpas FJ. Assessment of subcellular ROS and NO metabolism in higher plants: multifunctional signaling molecules. Antioxidants. 2019;8(12):641. doi:10.3390/antiox8120641. [Google Scholar] [PubMed] [CrossRef]
104. Costa MG, Prado RdM, Santos SMM, Souza JJP, de Souza AES. Silicon, by promoting a homeostatic balance of C:N:P and nutrient use efficiency, attenuates K deficiency, favoring sustainable bean cultivation. BMC Plant Biol. 2023;23(1):1–15. [Google Scholar]
105. Gambhir P, Singh V, Raghuvanshi U, Parida AP, Pareek A, Roychowdhury A, et al. A glutathione-independent DJ-1/PfpI domain-containing tomato glyoxalaseIII2, SlGLYIII2, confers enhanced tolerance under salt and osmotic stresses. Plant Cell Environ. 2023;46(2):518–48. doi:10.1111/pce.v46.2. [Google Scholar] [CrossRef]
106. Dindas J. Cytosolic Ca2+, a master regulator of vacuolar ion conductance and fast auxin signaling in Arabidopsis thaliana (Dissertation). Universität Würzburg: Germany; 2019. [Google Scholar]
107. Wang J, Li Y, Zhu F, Ming R, Chen LQ. Genome-wide analysis of nitrate transporter (NRT/NPF) family in sugarcane Saccharum spontaneum L. Trop Plant Biol. 2019;12:133–49. doi:10.1007/s12042-019-09220-8. [Google Scholar] [CrossRef]
108. Basu S, Roychoudhury A. Transcript profiling of stress-responsive genes and metabolic changes during salinity in indica and japonica rice exhibit distinct varietal difference. Physiol Plant. 2021;173(4):1434–47. doi:10.1111/ppl.13440. [Google Scholar] [PubMed] [CrossRef]
109. Ikuyinminu E, Goñi O, Łangowski Ł, O’Connell S. Biochemical and phenotypic analysis of the effects of a precision engineered biostimulant for inducing salinity stress tolerance in tomato. Int J Mol Sci. 2023;24(8):6988. doi:10.3390/ijms24086988. [Google Scholar] [PubMed] [CrossRef]
110. Patankar HV, Al-Harrasi I, Al-Yahyai R, Yaish MW. Functional characterization of date palm aquaporin gene PdPIP1;2 confers drought and salinity tolerance to yeast and Arabidopsis. Genes. 2019;10(5):390. doi:10.3390/genes10050390. [Google Scholar] [PubMed] [CrossRef]
111. Frallicciardi J, Melcr J, Siginou P, Marrink SJ, Poolman B. Membrane thickness, lipid phase and sterol type are determining factors in the permeability of membranes to small solutes. Nat Commun. 2022;13(1):1605. doi:10.1038/s41467-022-29272-x. [Google Scholar] [PubMed] [CrossRef]
112. Joshi S, Nath J, Singh AK, Pareek A, Joshi R. Ion transporters and their regulatory signal transduction mechanisms for salinity tolerance in plants. Physiol Plant. 2022;174(3):e13702. doi:10.1111/ppl.13702. [Google Scholar] [PubMed] [CrossRef]
113. Guan M, Chen M, Cao Z. NRT2.1, a major contributor to cadmium uptake controlled by high-affinity nitrate transporters. Ecotoxicol Environ Saf. 2021;218:112269. doi:10.1016/j.ecoenv.2021.112269. [Google Scholar] [PubMed] [CrossRef]
114. Plett DC, Ranathunge K, Melino VJ, Kuya N, Uga Y, Kronzucker HJ. The intersection of nitrogen nutrition and water use in plants: new paths toward improved crop productivity. J Exp Bot. 2020;71(15):4452–68. doi:10.1093/jxb/eraa049. [Google Scholar] [PubMed] [CrossRef]
115. Dromantienė R, Pranckietienė I, Jodaugienė D, Paulauskienė A. The influence of various forms of nitrogen fertilization and meteorological factors on nitrogen compounds in soil under laboratory conditions. Agronomy. 2020;10(12):2011. doi:10.3390/agronomy10122011. [Google Scholar] [CrossRef]
116. Swetha B, Singiri JR, Novoplansky N, Grandhi R, Srinivasan J, Khadka J, et al. Salinity and short-term exposure to high temperature altered dead pericarp and diminished yield of the crop plant Brassica juncea. Authorea Preprints. 2024. doi:10.22541/au.170664580.09337292/v1. [Google Scholar] [CrossRef]
117. Franczuk J, Tartanus M, Rosa R, Zaniewicz-Bajkowska A, Dębski H, Andrejiová A, et al. The effect of mycorrhiza fungi and various mineral fertilizer levels on the growth, yield, and nutritional value of sweet pepper (Capsicum annuum L.). Agriculture. 2023;13(4):857. doi:10.3390/agriculture13040857. [Google Scholar] [CrossRef]
118. Zhang Y, Fu L, Jeon SJ, Yan J, Giraldo JP, Matyjaszewski K, et al. Star polymers with designed reactive oxygen species scavenging and agent delivery functionality promote plant stress tolerance. ACS Nano. 2022;16(3):4467–78. doi:10.1021/acsnano.1c10828. [Google Scholar] [PubMed] [CrossRef]
119. Mokshina N, Panina A, Galinousky D, Sautkina O, Mikshina P. Transcriptome profiling of celery petiole tissues reveals peculiarities of the collenchyma cell wall formation. Planta. 2023;257(1):18. doi:10.1007/s00425-022-04042-7. [Google Scholar] [PubMed] [CrossRef]
120. Singhal RK, Fahad S, Kumar P, Choyal P, Javed T, Jinger D, et al. Beneficial elements: new players in improving nutrient use efficiency and abiotic stress tolerance. Plant Growth Regul. 2023;100:237–65. doi:10.1007/s10725-022-00843-8. [Google Scholar] [CrossRef]
Cite This Article
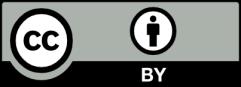
This work is licensed under a Creative Commons Attribution 4.0 International License , which permits unrestricted use, distribution, and reproduction in any medium, provided the original work is properly cited.