Open Access
REVIEW
Coffee Leaf Rust (Hemileia vastatrix) Disease in Coffee Plants and Perspectives by the Disease Control
1 Instituto de Ciencias Agrícolas de la Universidad Autónoma de Baja California (ICA-UABC), Nuevo León, Baja California, 21705, México
2 Laboratorio de Biología Molecular del Instituto Tecnológico Nacional de Tuxtla Gutierrez (TecNM), Tuxtla Gutierrez, Chiapas, 29050, México
3 Facultad de Ciencias Agronómicas Campus V. Universidad Autónoma de Chiapas (UNACH), Villaflores, Chiapas, 30470, México
* Corresponding Author: Daniel Gonzalez-Mendoza. Email:
(This article belongs to the Special Issue: Abiotic and Biotic Stress Tolerance in Crop)
Phyton-International Journal of Experimental Botany 2024, 93(5), 923-949. https://doi.org/10.32604/phyton.2024.049612
Received 12 January 2024; Accepted 02 April 2024; Issue published 28 May 2024
Abstract
Coffee Leaf Rust (CLR) is caused by Hemileia vastatrix in Coffea spp. It is one of the most dangerous phytopathogens for coffee plantations in terms of coffee productivity and coffee cup quality. In this review, we resume the problem of CLR in Mexico and the pathogenesis of H. vastatrix. The review abord plant-pathogen interactions which lead a compatible or incompatible interactions and result in CLR disease or resistance, respectively. The review abord Coffea spp. defense response pathways involved in H. vastatrix pathogenicity. Additionally, current measures to control H. vastatrix proliferation and germination were aborded focused on phytosanitary actions, and biological and chemical control. Finally, new trendlines to reduce the impact of CLR as nanoparticles and nanotechnology were analyzed.Keywords
Nomenclature
AgNPs | Silver nanoparticles |
CLR | Coffee Leaf Rust |
CBB | Coffee Berry Borer |
CeNPs | Cerium nanoparticles |
CeONPs | Cerium oxide nanoparticles |
CLM | Coffee Leaf Miner |
CMCS | Carboxymethyl chitosan |
CSB | Coffee Stem Borers |
CS | Chitosan |
CuNPs | Copper nanoparticles |
CuO-NPs | Copper oxide nanoparticles |
Cu/Zn-NPs | Bimetallic copper/zinc nanoparticles |
CWSB | Coffee White Stem Borer |
DAMPs | Damage Associated Molecular Patterns |
EO | Essential oil |
ET | Ethylene |
ETS | Effector-Triggered Susceptibility |
HDT | Timor Hybrid |
HR | Hypersensitive response |
HMCs | Haustorial Mother Cell |
ISR | Induced Systemic Responses |
JA | Jasmonic acid |
Ms | Mentha spicata |
MnNPs | Manganese nanoparticles |
NPs | Nanoparticles |
OEE | Orange essential oil |
PAL | Phenylalanine ammonia-lyase |
PAMPs | Pathogen Associated Molecular Patterns |
PbS NPs | Lead Sulfide nanoparticles |
PE | Penconazole |
PR | Protein-related Pathogen |
PRRs | Pattern Recognition Receptors |
PTI | PAMPs triggered immunity |
RLKs | Receptor-Like Kinases |
RLP | Receptor-Like Protein |
ROS | Reactive Oxygen Species |
SA | Salicylic acid |
SeNPs | Selenium nanoparticles |
SiNPs | Silicon nanoparticles |
SNPs | Silver nanoparticles |
TiNPs | Titanium nanoparticles |
TiO2 | Titanium oxide nanoparticles |
ZnNPs | Zinc nanoparticles |
ZnO-NPs | Zinc oxide nanoparticles |
Coffee trade is one of the four main commodities in agricultural products, and it could be the second commodity in countries in development, especially for coffee export. Around the world, coffee commerce represents an income for over 20 million of families in 56 countries [1,2]. In 2022, Mexico was in the 7th place in Coffea arabica worldwide production with 702,686.02 ha dedicated to coffee planting with productivity of 1,025,034.80 tons as well as a significant income of 6,534,603.54 thousand Mexican Pesos [3,4]. In 2023, Mexico was in the 6th place of C. arabica worldwide production and in the 10th place of coffee in general (C. arabica and Coffea canephora) worldwide production [4]. In Mexico, the coffee commerce could significantly affect the economic dependence of 300,000 coffee producers where 96% of the cultivated surface is destined for C. arabica and 80% of the national production is focused on the exportation of coffee [2,5].
Coffee Leaf Rust (CLR) is caused by Hemileia vastatrix which belongs to the Basidiomycota phylum, Pucciniomycetes class, and Pucciniales order [6]. H. vastatrix uredinospores deposits in the leaf underside and is an obligate parasite, which means H. vastatrix feeds through on coffee leaves to live and complete their reproductive cycle while their host is alive (feeding from host nutrients). CLR disease symptomatology enlists the apparition of chlorotic spots in leaves, reduction of the potential photochemical yield of photosystems, defoliation, and reduction of coffee plants’ productivity and quality of produced coffee. Also, the disease interferes with plant growth and development because of the limitation of coffee plants in nutrient uptake. Even in controlled plantations, the symptoms of diseased plants can continually evolve and affect the growth and development of coffee plants in subsequent years [7–10].
The losses in coffee productivity are divided into primary losses and second losses, the primary losses involve fruit loss by defoliation and death of damaged branches, and the second losses are caused by the cost of energy to plants to recover from the primary losses [11]. Coffee productivity losses by CLR can lie between 15%–20% and increase up to 70% [9,10]. In Colombia, losses of about 31% were reported from 2008 to 2011 in comparison with the production of 2007 [12]. In 2016, Mexico reported losses of 50% of the national production due CLR outbreak struggling the country since 2012 [13,14]. Guatemala reported losses between 59%–70% in 2012 [15]. After the crisis of 2013, Peru reported losses of up to 60% of its coffee production [16]. Centro America reported losses of up to 30% in 2013 [17]. Recent CLR outbreaks report an annual loss between one to two billion US dollars [18,19].
H. vastatrix affects domestic and wild Coffea spp. in the majority of C. arabica varieties and some C. canephora varieties, the CLR can be considered the most severe disease in coffee plantations due to its high propagation rate, in records CLR has reported monumental losses as was informed in Ceylon (now Sri Lanka) at the first CLR reported apparition in 1869 when the island was one of three major coffee producers worldwide; the disease spread to the main coffee producers states in Ceylon quickly with devastating consequences, and near of a decade later, it forced the coffee producers to abandon their production and dedicate to other plantations as tea plantations [20–22].
After the first report of CLF apparition, H. vastatrix spores spread to other coffee-producing countries. By 1990, the presence of CLR was reported in all coffee-producing countries except the island of Hawaii in the U.S. where the first apparition of CLR was reported in 2020 [7,9,20–24].
The germination of H. vastatrix spores is favored by dark humid environments between 21°C–27°C and available water on the host leaves for 8 h. Other factors can influence the grade of severity, e.g., the cultivated genotype determines the compatibility of the H. vastatrix spores and coffee plant interactions. Typica and Borbon Coffea arabica varietals are more susceptible to CLR disease. Also, the plantation density impacts the disease severity due to the dark-wet microenvironment generation in high-density coffee plantations. Fruit load in coffee plants also impacts the severity of the disease, in high-loaded plants the phenolic compounds with antimicrobial activity are mobilized to coffee berries [7,8,25,26].
H. vastatrix-Coffea spp. Interactions begin with the first contact of uredinospores and leaves underside. In optimal conditions and scenarios to develop a compatible interaction, uredinospores stick to epidermal cells and the germination tube grows until reaches the raised lips of the subsidiary cells of stomata [11]. H. vastatrix uredinospores germinates between 10°C–35°C with an optimum germination growth between 21°C–23°C, at temperatures below 15°C and above 35°C the fungal growth is slowed [19,27]. Altitude and rainfall patterns also affect the severity of the disease, in higher rainfall seasons the germination of uredinospores is favored [11]. Altitudes negatively impact the CLR severity, since CLR severity decreases with the altitude increase [28]. This is related to the decrement in the mean annual temperature in highlands in comparison with lowlands crops [28].
Pale et al. analyze the relationship between altitude, rainfall, and temperature level with CLR severity, their study analyses the data sets available by government organs in Mexico in different coffee producer municipalities. The results show a positive relationship between CLR severity and harvest period related to consistent and ripe fruit phenological stage in the coffee plants, the vegetative growth stage shows the lower CLR severity, and in the stage of fruit ripening the severity starts to increase. The results also were consistent with the positive relationship between rainfall seasons to CLR severity, rainfall seasons increase CLR severity due to the incensement in environmental moisture and leaf wetness, as well as increase the CLR incidence by water droplets splashed from infected leaves to healthy leaves [11].
CLR Disease’s first symptoms show as small chlorotic spots with 1–3 mm diameter and turn to orange when the infection sorus is formed. The principal infection structures are the uredinospores which were produced at the infection sorus. As the severity increases, the uredinospore production increases at the underside leaf. H. vastatrix uredinospores spreads by direct contact and can be mobilized by wind or water splashes [7,8,25,27]. H. vastatrix uredinospores can ascend to the atmosphere and propagate to other coffee plantations by wind turbulence and rain [29].
Since coffee plantations are a complex agroecosystem, understanding how the factors at the agroecosystem interact and impact the CLR dispersion and severity can help to adapt some agronomic practices to disease control [16,29,30]. Several techniques exist to prevent H. vastatrix germination and control CLR disease, e.g., proper crop management can be adapted to the current agricultural practices at the coffee plantations [31].
For the chemical part, the producers can apply some foliar fungicides and is recommended to apply them at the vegetative growth stage when the CLR incidence is still low [11]. The protective fungicides usually are made of copper compound and generate a protective coating in the leaves, these fungicides just prevent the first infection stages of H. vastatrix and slow down their propagation. On the other hand, systemic fungicides usually compounded by triazoles are more aggressive than protective fungicides, systemic fungicides inhibit ergosterol production [8,32].
Another chemical treatment alternative is the emerging field of nanotechnology. Nanotechnology is based on the synthesis or modification of nanoscale materials [33]. Nanoscale materials as nanoparticles can be synthesized from two main approaches, bottom-up and top-down [33]. Among the main advantages of the use of nanotechnology is the easy surface modification of nanoparticles to specific functions or applications [34]. Nanotechnology has gained attention in several agriculture fields due the its potential application in food safety packages, crop productivity, phytopathogens control, and soil health [33,35,36].
The present review provides an overview of our current knowledge of the coffee-H. testatrix interaction, mainly regarding pathogen infection and variability, and multilayered host defense responses. This review also contains information about the main phytosanitary measures to control coffee H. vastatrix and the potential use of nanoparticles as a possible control of this pathogen.
The Pucciniales (before named Uredinales) is a fungal order phytopathogen commonly known as Rusts. The Pucciniales represent one of the major severity and complex phytopathogens. The Pucciniales order is composed of 120 genera and 6,000 species. The main affected agriculture products are wheat, soja, and coffee crops which represent a high economic impact derived from crop losses [37]. Pucciniales are known for having a constant evolution with their host, which resulted in their adaptability and resistance. The Pucciniales infect their host as obligate biotrophic pathogens feed from the host cells’ nutrients, which means the pathogen feeds and grows only if the host is alive. In other words, rust depends on a living host to grow and reproduce [38]. Focused on H. vastatrix, their co-evolution with their host makes it more difficult to generate coffee resistance speciesn [39,40].
The rusts are categorized as specific host pathogens and their reproductive cycles are differentiated by their sporulation stages and how complete their reproductive cycle [39,41]. The rusts are caused by biotrophic fungi, and depend entirely on their living hosts, some rusts need two taxonomically different hosts (aecial and telial hosts) to complete their reproductive cycle (heteroecious), other rust (autoecious) as Hemileia vastatrix only have one kwon specific host and can complete their reproductive cycle above the same host or in different plant from the same species [23,37,42].
Fungi-caused rusts share some properties as they have highly developed infection structures, one of them is the haustorium a specialized structure to makes possible the nutrient uptake from the living cell host. Haustorium derives from haurire (in Latin), which means to drink or to draw [38]. Besides the haustorium role in nutrient uptake, they have limited secretory activity related to their lytic enzyme’s secretions and ensure the plant-pathogen compatible interaction due to the effectors delivery which suppresses the host defense signals for long periods and have a developed defense structure that separates the fungal and plant plasma membranes [23,43,44].
H. vastatrix is classified as primitive rust, since CLR discovery has a diverse catastrophe registered, the first registered apparition of H. vastatrix was in the Ceylon (now Sri Lanka) epidemic in 1869 [20–22,40]. It is a biotrophic pathogen and, hence needs a live host to live, feed, and complete its reproductive cycle. Besides C. arabica being susceptible to two rusts caused by H. vastatrix and H. coffeicola, only H. vastatrix has an economic worldwide impact on coffee cultivars countries [40].
H. vastatrix has ovoid/reniform uredinospores (single cell) with protuberances/spines at the dorsal and convex face and smooth surfaces in the ventral and concave face, uredinospores have thick walls and hydrophobic surfaces (Fig. 1). Mature uredinospores can develop up to 300 spines on average at their surfaces, their spines are grouped and functioned to grip and hold on into foliar tissues [42]. H. vastatrix teliospore has a smooth surface and its uredinospores and teliospores have hyaline walls with 1 um thickness. H. vastatrix forms supra stomatal sori with bouquet shapes [40].
Figure 1: Hemileia vastatrix uredinospores isolated from a coffee plantation in Angel Albino Corzo, Chiapas, Mexico with coordinates 15°45′33.8′′N 92°38′03.3′′W
The life cycle of H. vastatrix is hemicycle with autoecious reproduction. When the uredinospores achieve the invasion of coffee leaves, start to produce reproductive structures such as uredinospores, teliospores, and basidiospores. Uredinospores are the principal inoculum source to spread H. vastatrix. Basidiospores cannot penetrate and infect coffee leaves and teliospores rarely reinfect their host [40,42].
H. vastatrix uredinospores resist harsh environmental conditions and can survive in long drought periods or nutrient shortages and low temperatures. Uredinospores can stay dormant until the environmental conditions change. Uredinospore germination requires free-water, dark-wet environments for periods of 6–8 h. Environments between 21°C–27°C with high relative humidity (up to 80%) improve uredinospores gemination. Uredinospores were renewing at the infection sorus and represent the most important infection structure for the asexual cycle of H. vastatrix [6,19,39,40,42,45].
At the end of colonization, mature uredinospores develop infection sori and generate new uredinospores, basidiospores, and teliospores [24]. Teliospores produced promycelium which produced basidiospores [40].
The infection by H. vastatrix begins at the penetration phase by the uredinospore’s adhesion to the underside leaf surface, a crucial step in the H. vastatrix pathogenesis because it prevents the pathogen displacement from their host and improves the thigmotropism. Uredinospores-host first contact is fundamental to the establishment of the signal chain via transmembrane proteins [42,46]. By signal chain activation H. vastatrix can receive and process extracellular information into transcription factors, activating protein synthesis pathways to maintain the cellular wall thickness and morphogenesis [42,46].
After the first contact, the germination tube is formed during the uredinospores germination in the dikaryotic phase, the germination tube end has a hypha hook shape tip which senses and transduces the leaf topography information via signal chain, and the appressorium is formed after the stomata identification [23].
The appressorium is the first structure formed above the stomata by the uredinospores germination; this makes the stomata penetration begin. During the appressorium formation, lipids and glycogen are transferred from the cell to the appressorium. Once it is over the stomata, a penetration hypha grows to penetrate the stomata and reach the substomatal chamber with the differentiation of the substomatal vesicle [23,40]. In the parasitic phase, the penetration hypha differentiates to produce lateral branches that subsequently differentiate into haustorial mother cells (HMC) to produce a haustorium after its first contact with the mesophyll cell wall. Each lateral hypha resembles an anchor [23,40,42]. After 36 h of inoculation, the first haustorium is formed, infecting the near subsidiary cells [23,40].
As pathogenesis progresses (Fig. 2), H. vastatrix continues to form intercellular hyphae, HMCs, and haustorium, to invade substomatal cavities such as lower epidermal cells, mesophyll, spongy and palisade cells. At this stage, a dense mycelium is formed under the penetration stomata; at this point, chlorotic spots may be visible [23,40,47]. 21 days left, the sporulation phase begins with uredinospores bouquet shape sori are formed through the stomata as suprastomatal sori (Fig. 3A), at this stage, the chlorotic spot turns orange (Fig. 3B) and the uredinospores sori produces new infection structures which can be propagate easily through wind, rain splashes, and insects to restart the cycle in other leaves or plants. In advance of the disease severity, new uredinospores sorus are produced in the same leaf and the near leaves (Fig. 3C) [23,38,40,42,47].
Figure 2: Hemileia vastatrix reproductive cycle [23,40,47]
Figure 3: Hemileia vastatrix uredinospores and Sorus viewed in stereoscope (A) the uredinospores were isolated from coffee leaves with CLR (B) in a coffee plantation in Angel Albino Corzo, Chiapas, Mexico with coordinates 15°45′33.8′′N 92°38′03.3′′W (C)
3 Hemileia vastatrix-Coffea spp. Interactions
Rust fungi’s reproductive cycle usually involves two parasitic stages, dikaryotic and monokaryotic. The monokaryotic phase is related to basidiospore germination, basidiospores penetrates directly the epidermal cells. The dikaryotic phase starts with uredinospores germ tube formation and elongation for surface recognition. When the germination recognizes the subsidiary cells of the stomata, forms the appressorium over the stomata. The rust fungi produce sophisticated infection structures well differentiated and divided by septum, these structures help the fungi to avoid host recognition and improve the pathogenesis [38,39,43,48].
The CLR disease development starts in the dikaryotic phase when uredinospores begin in contact with the leaf’s underside. For this phase the adhesion of the fungi to the leaf surface is crucial. The adhesion of uredinospores is improved by the hydrophobic nature of uredinospores and the cuticle wax layer in the host leaves. The spines at the uredinospores surfaces also improve the adhesion by mechanical grip. After the first contact, an extracellular matrix is formed with low molecular weight carbohydrates and glycosylated polypeptides. The formation of the adhesion pad seems to be by the activity of cutinases and esterases [42]. The germ tube recognizes the topography of the ridge stomatal lips and the leaf alcohols and sends the signals to start the appressorium differentiation from the germ tube, the surface recognition involves chemical and physical signals as topography, hydrophobicity, and the presence of cutin monomers [39,42,43,48,49].
The success of infection may be determined by the susceptibility of the host to the rust race. The differentiation of the infection hypha begins with one of the fungi’s defense mechanisms as the production of suppressors of defense to invade their host easily [39,42,48]. Independent of the plant-pathogen interaction results the H. vastatrix uredinospores and their complex; pre and post-penetration structures have an arrangement to evade the host chitinases, where the chitin is inside the structures and the outside in contact with the host environment are labels of β-1,3-glucans [49].
The morphological differentiation due to the formation of infection hypha is crucial in the pathogenesis successful. The penetration hypha enables nutrient uptake, otherwise, the growth of the germination tube depletes the fungi’s nutrients. After the first haustorium formation, the nutrient uptake is limited, hence, the fungi are forced to change their fungal wall composition. This event marks a change in protein synthesis, ribosome synthesis, and secretion of hydrolases [39,42,48].
Once the appressorium is formed above the stomata, an infected peg starts to grow from Haustorial Mother Cells (HMC). The infection peg grows between guard cells through substomatal space and forms the substomatal vesicle. After then the intercellular hypha is formed, the hosts start to detect the phytopathogen presence by the delivered elicitors. At the first contact of the HMC with mesophyll cells, the HMC starts to differentiate into haustorium to begin the host cell infection. The mechanisms of haustorium infection are similar to those of the appressorium, the HMC attaches to the host cell wall and forms a penetration hypha, the HMCs are protected with a thick multilayer wall, the mechanisms of appressorium penetration are related to the application of pressure and secretion of lytic enzymes over the penetration zone previously, cell wall degradation is restricted to the penetration zone [39,42,43,48,49].
The haustorium is involved in intimate communication with the host cells, as well are implicated in the suppression of host defense response and nutrient uptake [42]. This intimate communication between the haustorium and the cell hosts is possible due to the formation of an extra haustorial matrix and membrane which divides the haustorial plasma membrane and the haustorium body from the plant plasma membrane [42]. The extra haustorial matrix is composed primarily of biomolecules derived from the cell hosts and provided by the fungi during the infection, this matrix makes an intimate contact zone where the haustorium body is protected from the plant plasma [39,42,48].
The haustorium (Fig. 4) is formed from the neckband that maintains and connects the HMC with the haustorium. The neckband protects the haustorium by sealing the haustorium interfaces from the apoplast flux. The neckband is the first infection structure formed after HMC hypha penetration. The penetration hypha broke the cell host membrane due to the applied pressure and the secretion of lytic enzymes. After the haustorium formation, the extra haustorial matrix and membrane were formed. The absence of ATPase activity at the haustorial membrane implies an influx of solutes from the host cell without control [42]. The formation of the haustorial involves a re-organization of the cell host components and cytoskeleton, and a continued exchange and secretion of proteins, effectors, and elicitors from the haustorium [42,43].
Figure 4: Schematic representation of Hemileia vastatrix infection structures in Coffea spp. leaves [42,43]
During the penetration, parasitic, and sporulation phases the fungi produce sophisticated highly specialized structures that produce proteins, enzymes, and secondary metabolites to adapt and evade the host defense mechanism and assure their sporulation phase and therefore, complete the reproductive cycle [38,42,43,50,51]. The secondary metabolite productions can alter the hormone pathways as auxins, gibberellic acids, jasmonic acid, and salicylic acid [43].
Plants’ susceptibility and resistance to pathogens is determined by the interactions between host plants and pathogens. Plants have their own recognition and defense mechanisms to identify and protect them from potential pathogens and dangerous conditions. Plant defense mechanisms are complex and involve passive and active defense lines. The passive (natural) or nonhost resistance mechanisms involved some barriers to prevent adhesion and penetration of infection structures. Whitin’s passive mechanism can be found in the deposition of wax cuticles, lignification of cell walls, the production of secondary metabolites, and silica accumulation [24,52–54].
The active defense lines involve the pathogen and damage recognition. The Pattern Recognition Receptors (PRRs) detect molecules related to pathogen invasion as Pathogen Associated Molecular Patterns (MAMPs or PAMPs) or related to host stress/damage as Damage Associated Molecular Patterns (DAMPs). PRRs are transmembrane proteins, in the presence of PAMPs or DAMPs that bind or release to a protein system to activate the downstream of immune signaling. PRRs detect microbial elicitors produced during the pathogen invasion (MAMPs or PAMPs), this recognition process is called PAMP-triggered immunity (PTI). PRRs are mainly receptor-like proteins (RLP), and receptor-like kinases (RLKs) [40,49,53,55].
Elicitors can be sourced from pathogen secretions (exogenous) or delivered molecules from wound plant tissues (endogenous) [49,55]. The elicitors can be specific or non-race specific. Usually, the elicitors are composed of peptides, proteins (esterases), glycoproteins, fatty acids derivatives, sterols, oligosaccharides, or polysaccharides [46,49,55]. Endogenous elicitors are linked to DAMPs and are produced in wound tissue by enzymatic lytic activity, blocking or detriment of mechanical barriers due to the advance of the disease [46,49,55].
DAMPs act as a long-distance immune mechanism and activate the local and systemic defense signaling. DAMPs are molecules released by damaged cells due to pathogen invasion or by cells being under attack. DAMP molecules are primarily fragments of polysaccharides cut off cell membranes, cytosolic proteins, and other macromolecules as peptides, amino acids, and nucleotides [53]. PAMPs and DAMPs can activate immune responses to PTI in synchrony to increase or amplify the immune signals, as well PTI and ETI can overlap to work in parallel or in synchrony to suppress the pathogen propagation [24,53].
Effector Immunity (ETI) is another active defense mechanism based on the pathogen invasion detection by pathogen effectors. Effectors are small proteins produced and mainly secreted by pathogens to achieve colonization [55–57]. Effectors can modify the structure and function of host cells and also can modify the host metabolism and defense pathways. Some effectors are capable of interfering with PTI and ETI which results in a compatible interaction due to the induced Effector-Triggered Susceptibility (ETS) to the host [24,55–58].
When the effector is detected by R protein receptors active ETI or PTI the invaded plant starts to alert the defense system. Hence the host cells activate a signal cascade related to increased ion fluxes. The increment of cytoplasmatic calcium concentrations is related to PTI. Calcium ions function as a secondary messenger for the production of stress-related hormones. This activates the signaling pathways of salicylic acid (SA), jasmonic acid (JA), and ethylene (ET). The SA pathway is the more related pathway to suppress the compatibility interaction due to the activation of defense enzyme mechanisms. SA activates the expression of phenylalanine ammonia-lyase (PAL), oxidative burst, ROS accumulation, and protein kinase cascade activation [24,32,55,56]. In coffee-resistant cultivars, the SA pathway is activated by the expression of resistance genes (CaNPR1, CaPR1, and CaPR5) [59]. ETI and PTI can induce similar defense mechanisms, e.g., both can activate the salicylic acid pathway considered as the main defense mechanism against H. vastatrix, but ETI responses seem to be more prolonged than PTI [24,55,56].
Other defense mechanisms involved in Coffea spp. response against H. vastatrix is the activity of the enzyme Polyphenol Oxidase (PPO), an enzyme containing copper as a cofactor and 5-caffeolyquinic acid (chlorogenic acid) as a substrate. PPO catalyzes the oxidation of phenolic compounds (monophenols → o-diphenols → quinones) near to wound site when the tissue is damaged stopping the germination by Hypersensitive Response (HR) acceleration, reducing the bioavailability of alkylate proteins and by the improvement of polymerization of proteins to form physical barriers [60–63]. Other post-haustorial defense mechanisms are related to the enzymatic activity of Plant Peroxidases (PODs) as PPO, PAL, β-1,3-glucanase, chitinase by the degradation of chitin at the H. vastatrix cell wall, and lipoxygenase [32,63–65].
Pathogens can respond to plant defense systems to avoid it and lead to a successful pathogenesis. In the same way, plants can prevent or respond on time to the pathogen invasion and break the pathogenesis. Therefore, leading a successful resistance to that pathogen. E.g., the HR activation by the expression of the gene CaNDR1b in coffee cultivars is involved in pathogen recognition [59]. The HR is activated with the first haustorium formation as an early defense mechanism by the detection of effectors by PRRs. This provokes the production of Reactive Oxygen Species (ROS) to “eliminate” the infected cell and cut the pathogen nutrient uptake. The activation of HR also is related to the deposition of callose, cell wall lignification, and synthesis of chlorogenic acid [49,51,53,58,66,67].
These responses are the result of the co-evolution of some plant pathogens and could provide some physical or chemical barriers and the production of elicitors or effectors to activate or deactivate the barriers of the counterpart [49]. In dependence of the organism’s genetic material, these barriers or defense mechanisms could be activated as preventive and protective mechanisms and others could be induced by the detection of pathogen attack. Some defense mechanisms are hypersensitive reaction, phytoalexins production, structural barriers, production of elicitors, and activation of systemic mechanisms [42,49–51].
H. vastatrix interactions with Coffea spp. respond to the gene-for-gene model proposed by Flor as Coffea spp. race-specific defense mechanism [68,69]. The gene-for-gene model explains how plant-pathogen interactions could lead to susceptibility or resistance because of specific parasite responses, the model explains the resistance when each elicitor produced by the virulence gene expression and interacts with a virulence gene receptor in the host organisms, this binding active a signal cascade and therefore plant defense system and produced an incompatible plant-pathogen interaction [55]. Each effector expressed by a specific Avr gene is recognized by a specific R gene, leading to an incompatible interaction. When the host and pathogen have a straight co-evolution relationship, the Avr genes tend to mutate to evade the recognition system by the host R genes leading to a compatible plant-pathogen interaction or a susceptibility case of the host to the pathogen [55,58,67,70].
In the gene-for-gene model, each dominant resistance gene (SH1-SH9) conferring coffee leaf rust resistance for coffee spp. is one virulence gene (v1-v9) in the H. vastatrix genome, and the genes are present individually or in groups for each Coffea species or H. vastatrix race [13,49,68–70]. The Severity of the pathogenesis is determined by the interaction of the genes in the Coffea species and H. vastatrix race. The recognition of H. vastatrix virulence genes by resistance genes in Coffea species tends to an incompatible plant-pathogen interaction. The v1-v9 genes’ presence in H. vastatrix races defines their severity, at now at least 50 H. vastatrix races are known worldwide, and the races with less genetic material are more dangerous as their sporulation times and capacity are shorter than the races with more virulence genes [13,49,56,68,70,71].
In Mexico and Brazil as in other coffee producers in Central and South America race II (v5) is the most common in coffee crops. In America races I (v2, v5), III (v1, v5), XV (v4, v5) and X (v1, v4, v5) are also common [13,72]. In pure arabicas are found genes SH1, SH2, SH4, and SH5, in C. liberica is found the gene SH3, in C. canephora, are found the genes SH6, SH7, SH8, and SH9, and also found in Timor Hybrid HDT (SH6-SH9) due its hybridization origin from C. arabica × C. canephora [13,69]. Coffea genotypes can be classified according to their resistance or susceptibility to H. vastatrix races, Group A is related to coffea genotypes with resistance to all known H. vastatrix races, while Group E is for the Coffea genotypes with susceptibility to all known H. vastatrix races [49,69].
Following the concepts in the gene-for-gene model, Coffea plants can perform a complete or partial resistance to CLR; complete resistance is achieved when the defense SH1-9 genes bind with virulence v1-9 genes in H. vastatrix. Incompatible plant-pathogen interaction is expressed in the resistance coffea genotype when homozygous dominant genes AA and RR are present in each organism (H. vastatrix and Coffea species respectively), dominant heterozygous Aa and Rr also result in an incompatible plant-pathogen interaction, while recessive genes aa and rr results in a compatible plant-pathogen interaction or a successful disease [46].
Plant-pathogen interaction between Coffea spp. and H. vastatrix leads to a compatible, incompatible, and partially compatible, thus as a result the balance of Coffea spp. defense mechanisms expressed and H. vastatrix adaptability and pathogenicity (Fig. 5). As mentioned above, Coffea spp. have different defense mechanisms involving their natural or passive and active mechanisms related to a specific host or no, and these mechanisms can be classified as those expressed before the first haustorial formation and those expressed after the first haustorial formation [24].
Figure 5: Schematic representation of possible plant-pathogen interaction scenarios where (A) represents unsuccessful interaction due specificity of appressorium hypha to penetrate stomas as represented in (B). (C) represents an incompatible interaction due hypersensitive response of Coffea sp. due pathogen recognition system, which is avoided in (D) by the effectors liberation by haustorium leading in a full compatible interaction as is represented in (E) [42,43]
HR in Coffea species is one of the main defense responses and is activated in both cases, compatible and incompatible interaction, HR can be activated and amplified by PTI and ETI at the first contact with the uredinospores with host leaves, but the ETI mechanism is more related with a complete incompatibility interaction or disease resistant [24]. HR usually begins in stomata and subsidiary cells as guard cells and mesophyll cells [24]. Other effective defense mechanisms in incompatible interactions are associated with pathogenesis-related proteins (PRs) as PR2, PR3, PR10, PR15, and PR16 were related to incompatible responses, especially PR2 (β-1,3-glucanase) and PR3 (chitinase) [24,64].
Luján-Hidalgo et al. [73] reports in 2020 the biochemical responses of C. arabica plants to H. vastratrix infection where they found a significant increase in the amount of caffeine and gallic acid presence in infected leaves in comparison with uninfected ones which results can be related to Induced Systemic Responses (ISR), as well an increase of enzymatic activity (chitinases and β-1,3-glucanases) associated to protein-related pathogen (PR) [64,73]. An emerging alternative in the control of CLR is the technological development from the genomics perspective, e.g., the foliar application of RNA interference (RNAi) using spray-induced gene silencing (SIGS). SIGS can be applied to silence pre and post-exhaustorial effectors genes in H. vastatrix uredinospores germination [74].
5 Current Control of Coffee Leaf Rust (Hemileia vastatrix) in Coffee Plantations
The main phytosanitary measures to control CLR propagation are preventive and corrective actions, in the preventive action is the control of plant population density to prevent shadow excess by the overlapping of coffee plants. In some cases, the farmers tend to breed or cultivate CLR-resistant cultivars such as HDT, a resistant cultivar derived from the natural hybridization of Coffea arabica × Coffea canephora with the resistance genes SH6-SH9 [6,75]. In general, the main actions to control CLR can be divided into phytosanitary measures where the farmers take actions to prevent favorable germination and sporulation conditions of H. vastatrix, where the flowering cycles can be used as an indicator to start the phytosanitary actions [76]. Genetic control with resistance cultivars and breeding with new resistance cultivars by resistance genes present in Timor Hybrid HDT [6,75].
The management of shadow and pruning helps in the control of the disease, excess of shadow reduces the photosynthetic reactions in the lower coffee plants by the canopy, also, the overlap of branches in the canopy generates microclimates and promotes the germination of H. vastatrix spores [16,31]. Pruning as a renewal technique removes the old unproductivity branches, which could be a source of inoculum for several microorganisms, and promotes aeration and photosynthesis [16,31].
Gonzales et al. demonstrate the relationship between shadow system, pruning techniques, and cultivated genotype in a study conducted in 2022. The study analyzes the effect of pruning techniques (non-pruning, descope, medium pruning, and recipe) in susceptible C. arabica varieties such as Typica and Pache. Their results reduce de percentage of incidences of CLR from non-pruning by 37.81% in contrast with coffee plants pruning by recipe method by 16.15%. The reduction in the percentage of incidence was more representative in C. arabica Typica than in Pache varietal in the difference shadow system and pruning methods analyzed [16].
Reducing the plantation density also can reduce the CLR severity, in high-density plantations the environmental moisture between plants and the plant-to-plant interactions can be increased [29]. A studio simulated by Mora et al. analyses the interplay between harvesting, plant density, and ripening time in the dispersion of CLR. The results show an increment in CLR dispersion in high-density plantations analyzed from 500 plants/ha to 5000 plants/ha, where the rust incidence increases from 500 to 3000 plants/ha with the highest incidence at 2000 plants/ha. The study also shows the importance of caring about synchrony in the ripening, asynchrony ripening can lead the agricultures to work long distances per day, which increases the probability of spreading the uredinospores by contact [29].
Coffee plantations shadowed by trees also play a crucial role in CLR dispersion, planted trees in the agroforestry systems can improve the coffee plants metabolism and the physiological resistance [30,77]. The shape of trees at the canopy can increase the CLR severity by the microclimate generation and reduce the CLR dispersion. Planted trees at the periphery can reduce the CLR dispersion airborne [16,29,30]. In a study conducted by Daba et al. in 2015/16 and 2020/21 showed lower CLR incidence in a forest coffee system (FC) than in a semi-forest coffee system (SFC), in FC the human activity is limited so the dispersion of urediniospores is limited, also the dense canopy in the FC reduces the wind speed and penetration of water droplets [77].
Chemical control involves the use of organic or inorganic pesticides. Biological control involves the use of natural pesticides elaborated with plant extracts or by the application of antagonist microorganisms [41]. Chemical and biological control have special attention due to their function of elicitors with properties to active natural defense pathways in coffee plants. For, Lam et al. reported in 2019 the effectivity of methanolic extract of Baccharis glutinosa roots in C. arabica Var. Borbon and Caturra with significative inhibition of H. vastatrix uredinospores germination [41]. The results show a significant decrement in the germination capacity of uredinospores from 38.54 to 7.01, 1.67, and 2.2 in leaves treated with copper oxychloride and MEBs 100, 270, and 750 mg mL−1, respectively [41].
Ramirez et al. reported in 2020 a study conducted in 2018 in Oaxaca, Mexico. The study evaluates the CLR severity in C. arabica var. Geisha seedlings inoculated with H. vastatrix uredinospores. They compared the effect of 24 treatments by foliar application to Geisha seedlings. The treatments with the higher inhibition were Bacit-Sur+Nat-R composed of colonies of Bacillus subtilis and Trichoderma spp. with hepar sulfur at 15%, Bovista plumbea at 5% and homeopathic plant extract at 10% in water. The treatment Bacit-Sur+Nat-R shows a significative lower CLR incidence in contras of copper oxychloride (Oc) [10].
Besides the agronomic phytosanitary measures, the application of chemical products can lead to protecting coffee plants in a preventive or systemic way. Cupric pesticides usually are used in combination with systemic or systemic pesticides to avoid pathogen resistance to pesticides [78,79]. Cupric fungicides are the most used as protective or contact fungicides due to their capacity to make a coating over the treated leaves. Furthermore, copper improves the vigor and retention of coffee leaves [80]. The availability of copper ions in the foliar surfaces inhibits the first stages of infection of H. vastatrix.
If the used pesticide contains copper in its reactive chemical formula (Cu2+) the copper ions can reduce the germination of urediniospores and penetration of hyphae because copper inhibits the respiratory process, and protein synthesis and weakens the cell membrane, also the copper tends to interact with pathogen cell wall due their affinity to carboxyl groups available on the surface [6,8,31,79–81]. Cu ions can cause cell death by their capability to interrupt metabolic pathways, development of oxidative stress, and loss of membrane integrity due to Cu ions absorption which leads to the generation and accumulation of ROS [80,81].
Inside the main disadvantage of copper fungicides is the need to reapply constantly to protect new leaves and due their washability by rain or irrigation. Also, the copper fungicides start to lose efficiency at 10% of infection of H. vastatrix [6,8]. The main used copper fungicides are copper oxychloride, copper hydroxide, copper oxide, and copper sulfate [6,8,19].
The corrective or systemic fungicides can stop the infection in the early stages (before sporulation), in comparison with protective pesticides, the systemic pesticides are absorbed by the plant and transported to act on the infection site, in other words, the systemic pesticides work from inside the leaves instead the leaves surfaces. E.g., the triazole compounds inhibit ergosterol production but the effectiveness is reduced at the sporulation stage of the disease. Mesostemic fungicides as strobilurins work from the wax cuticle in the leaves and inhibit the sporulation of H. vastatrix. Hence is recommended the combined application of strobilurins and triazole compounds [6,8,78,79,82]. The mixture of triazoles and strobilurins can be applied in the soil or the foliar surface [78,79,82]. Some common triazoles used in Mexico and Latin America are epoxiconazole, cyproconazole, azoxystrobin, and tebuconazole which can be applied alone or in combination [83].
6 Potential Use of Nanoparticles in the Control of Pests and Diseases in Agriculture
The nanoparticles (NPs) can be defined as a particle with a length of 1 to 100 nm in any axis (x, y, or z), at this dimension the particles behave as “big molecules” instead of bulk materials. This improves the reactivity and the surface area making it more effective in the nanoparticulate form than in the bulk size [84,85]. The nanoparticles can be classified mainly by the precursor material or by the synthesis mechanisms. When the nanoparticles are obtained from a macroscopic source the synthesis route is called “top-down” and the mostly methods used are electrochemistry, nanolithography, laser ablation, and thermal decomposition [84,86,87]. The route is called “bottom-up” when the nanoparticle synthesis starts from chemical precursors as in sol-gel, photosynthesis, chemical reduction, and nano-emulsions among others. Otherwise, can be classified in order of their chemical source as organic or inorganic nanoparticles [84,86–88].
The application of nanoparticles has been popularized due to the surface-area ratio improving the efficacy over their bulk-size counterparts. The nanoparticles have been studied and applied in the agricultural industry in the development of technologies for food packaging, pesticides, and fertilizers. In these formulations, the nanoparticles are considered as an active ingredient or as a vehicle for active ingredients. The main studied inorganic nanoparticles in the control of phytopathogens are the nanoparticles from silver (AgNPs), copper (CuNPs), zinc (ZnNPs), Cerium (CeNPs), silicon (SiNPs), and titanium (TiNPs) [88–90].
Organic nanoparticles also have been applied in the control of phytopathogens, e.g., the application of salicylic acid and chitosan nanoparticles in the control of Punccinia triticina, the nanosystem report to causes the lysis of the uredinospores [91]. The functional groups in the surfaces of the nanoparticles can be modified to functionalize the nanoparticles or to synthesize a nanocomposite or a nanosystem [88–90]. SiNPs and TiNPs are commonly functionalized to use as vehicles for active compounds. E.g., the surface modification of SiNPs with chitosan oligosaccharides tested in-vitro in the inhibition growth of Fusarium spp. [90].
Recently has been reported the application of AgNPs and CuNPs in the suppression of germination of H. vastatrix uredinospores [92]. Little information has been reported on the application of nanotechnology in the control CLR or in the inhibition of germination of H. vastatrix uredinospores. Table 1 resumes recent publication information on the control of phytopathogens by nanotechnology.
The main action mechanisms of nanoparticles involve the generation of ROS by the photocatalytic activity of nanoparticles, thus damaging the cell organelles, and biomolecules as oxidation of proteins and DNA degradation, inducing oxidative stress and interfering with the electron chain transport [107,108]. The concentration of produced ROS depends on the nanoparticle’s chemical nature and nanoparticle dose [107]. Metallic nanoparticles can release metal ions, the released ions can modify the structure of biomolecules by their interaction with thiol, amino, and carboxyl functional groups [108]. In photosynthesized nanoparticles, the released ions can carry antimicrobial compounds derived from the plant extract [108].
Nanoparticles interact with the whole environment after they apply to a plant, interacting with the plant of interest, the phytopathogens, the soil, and the habitats of the soil [109,110]. The nanoparticles can enter the plant depending on the application via the nanoparticle physicochemical properties [109]. Nanoparticles can enter to plant by leaves and root uptake [109]. Can be administrated in seed imbibition or seedlings/plants by foliar application or soil supply [111]. The uptake and assimilation of nanoparticles are carried out by roots and leaf tissues such as leaf epidermis and stomata, as well as by wounded tissues [109,111]. The applied nanoparticles can mobilize or translocate inside plants by the apoplastic and symplastic pathways [109,111].
Besides the positive effects of nanoparticles in the control of phytopathogens and promoting tolerance to biotic and abiotic stress, nanoparticles can be harmful to studied plants [111,112]. The main factors in the toxicity of nanoparticles are the size, morphology, chemical nature, and dose [110,111,113]. Penetration and translocation of nanoparticles inside the plants concern the uses of nanoparticles. Thus, can generate the bioaccumulation of nanoparticles in plant organelles such as vacuoles and vesicles [114,115].
The excessive generation of ROS conduces to an imbalance with the plant antioxidant system and leads to phytotoxic effects. The excess of ROS can lead to oxidative burst, cell death, and stomata closure, leading to a reduction in CO2 fixation [111,113,116]. The foliar application of nanoparticles in high concentrations can induce physiological damage as a reduction of the photosynthesis rate. This is related to stomatal closure and blockage of chloroplast and mitochondria electron transport chain [111,113]. Table 2 shows some phytotoxic effects of nanoparticles.
The possible toxicological effects of nanoparticles concern the application of nanoparticles in organic and sustainable crops. Organic agriculture takes care of the soil, ecosystem, and people’s health [120]. Some requirements in organic and sustainable crop systems involve the integration of biological processes into the production chain, avoiding the use of toxic pesticides, reducing the use of non-renewable resources as much as possible, and implementing solutions derived from the farmer’s experiences and skills [120,121]. Since organic agriculture involves the use of innovative and scientific measurements combined with traditional practices, several studies must be done before applying nanoparticles to coffee farms with organic and sustainable systems [120–123].
To comply with organic and sustainable crop practices, it is important to assess the security of the crop environment. Before starting with applications of nanoparticles a comprehensive literature revision must be done. Understanding the action mechanism and the physicochemical properties of nanoparticles improves the comprehension of nanoparticle interactions. These interactions are related to how the nanoparticles impact the interest in phytopathogen, host plant, soil, and soil microbiome. The application of nanoparticles in phytopathogen control can be accompanied by biological control and sustainable crop practices with a complete overview of the nanoparticle’s impact on the farm environment.
The application of nanoparticles in the control of H. vastatrix germination is not been explored at now but has extreme potential in research, e.g., the phytotoxicity and safety amounts and types of nanoparticles in different phenological states of coffee plants or coffee seeds germination and how the nanoparticles applications can induce different responses in photosystems, secondary metabolites synthesis, and enzymatic activity or how nanoparticles can induce defense response pathways in coffee plants.
The disease caused by H. vastatrix represents a great problem phytosanitary in coffee plants that affects sustainable coffee production all over the world. Although there are various strategies for its control, it is a fact that integrated management is required to control this disease. In this sense, the use of new tools such as nanotechnology could be a possible alternative in the control of the disease. Therefore, future studies need to address an integrated approach to managing the disease with an international exchange of knowledge and biological materials and the implementation of new technologies.
Acknowledgement: The author acknowledges the Consejo Nacional de Humanidades, Ciencias y Tecnologías CONHACyT for the support offered to his studies as a postgraduate student from Doctorado en Ciencias Agrícolas at the Instituto de Ciencias Agrícolas in Universidad Autónoma de Baja California.
Funding Statement: The authors received no specific funding for this study.
Author Contributions: The study was conceived and designed by A. Salazar-Navarro, V. Ruiz-Valdiviezo, J. Joya-Davila, and D. Gonzalez-Mendoza. The manuscript was drafted by A. Salazar-Navarro. Funding was obtained by V. Ruiz-Valdiviezo, J. Joya-Davila, and D. Gonzalez-Mendoza. The study was supervised by D. Gonzalez-Mendoza, V. Ruiz-Valdiviezo, and J. Joya-Davila. All authors have reviewed and approved the published version of the manuscript.
Availability of Data and Materials: The data utilized in this study have been incorporated within the tables and pictures.
Ethics Approval: Not applicable.
Conflicts of Interest: The authors declare that they have no conflicts of interest to report regarding the present study.
References
1. Patay É.B, Bencsik T, Papp N. Phytochemical overview and medicinal importance of Coffea species from the past until now. Asian Pac J Trop Med. 2016;9(12):1127–35. doi:10.1016/j.apjtm.2016.11.008 [Google Scholar] [PubMed] [CrossRef]
2. Pérez Constantino A, Ramírez Dávila JF, Figueroa Figueroa DK. Infestations of the coffee berry borer, Hypotenemus hampei (Coleoptera: scolitydae) in coffee-growing areas of the State of Mexico, Mexico. Rev Colomb Entomol. 2023;49(1):1–5. doi:10.25100/socolen.v49i1.12097. [Google Scholar] [CrossRef]
3. Servicio de Información Agroalimentaria y Pesquera. Anuario Estadístico de la Producción Agrícola. Anuario Estadístico de la Producción Agrícola (In Spanish). 2022. Available from: https://nube.siap.gob.mx/cierreagricola/. [Accessed 2023]. [Google Scholar]
4. USDA. Coffee: world markets and trade. 2023. Available from: https://public.govdelivery.com/accounts/USDAFAS/subscriber/new. [Accessed 2023]. [Google Scholar]
5. Gasperín-García EM, Platas-Rosado DE, Zetina-Córdoba P, Vilaboa-Arroniz J, Dávila FM. Quality of life of coffee growers in the high mountains of Veracruz, Mexico. Rev Agron Mesoam. 2023 Jan 1;34(1):50163. doi:10.15517/am.v34i150163. [Google Scholar] [CrossRef]
6. Talhinhas P, Batista D, Diniz I, Vieira A, Silva DN, Loureiro A, et al. The coffee leaf rust pathogen Hemileia vastatrix: one and a half centuries around the tropics. Mol Plant Pathol. 2017;18(8):1039–51. doi:10.1111/mpp.12512 [Google Scholar] [PubMed] [CrossRef]
7. Romero JM, Camili J. Manual de Producción sostenible de café en la República Dominicana, vol. 1. 1st ed. Santo Domingo: IICA; 2019. p. 1–105 (In Spanish). Available from: http://www.iica.int. [Accessed 2022]. [Google Scholar]
8. Barquero M. Recomendaciones para el combate de la roya del cafeto, vol. 1. 1st ed. Barva: CICAFE; 2013. p. 1–76 (In Spanish). [Google Scholar]
9. Abigail Funlayo A, Olalekan Adenuga O, Mapayi E, Olufemi Olaniyi O. Coffee: botany, distribution, diversity, chemical composition and its management. IOSR-JAVS. 2017;10(7):57–62. doi:10.9790/2380-1007035762. [Google Scholar] [CrossRef]
10. Ramírez-Rodríguez RF, Castañeda-Hidalgo E, Robles C, Santiago-Martínez GM, Isabel Pérez-León M, Lozano-Trejo S. Efectividad de biofungicidas para el control de la roya en plántulas de café. Rev Mexicana Cienc Agric. 2020;11(6):1403–12 (In Spanish). doi:10.29312/remexca.v11i6.2614. [Google Scholar] [CrossRef]
11. Pale-Ezquivel I, Musule R, Pineda-López MDR, Alarcón-Gutiérrez E, Sánchez-Velásquez LR. Temporal progress of coffee leaf rust and environmental conditions affecting severity in Veracruz State, Mexico. Coffee Sci. 2023;18(1):e182047. doi:10.25186/.v18i.2047. [Google Scholar] [CrossRef]
12. Villarreyna R, Barrios M, Vílchez S, Cerda R, Vignola R, Avelino J. Economic constraints as drivers of coffee rust epidemics in Nicaragua. Crop Prot. 2020 Jan 1;127:104980. doi:10.1016/j.cropro.2019.104980. [Google Scholar] [CrossRef]
13. Torres Castillo NE, Melchor-Martínez EM, Ochoa Sierra JS, Ramirez-Mendoza RA, Parra-Saldívar R, Iqbal HMN. Impact of climate change and early development of coffee rust–An overview of control strategies to preserve organic cultivars in Mexico. Sci Total Environ. 2020;738(140225):1–14. doi:10.1016/j.scitotenv.2020.140225 [Google Scholar] [PubMed] [CrossRef]
14. Henderson TP. La roya y el futuro del café en Chiapas. Rev Mex Sociol. 2019;81(2):389–416 (In Spanish). doi:10.22201/iis.01882503p.2019.2.57874. [Google Scholar] [CrossRef]
15. Dupre SI, Harvey CA, Holland MB. The impact of coffee leaf rust on migration by smallholder coffee farmers in Guatemala. World Dev. 2022 Aug 1;156(105918):1–12. doi:10.1016/j.worlddev.2022.105918. [Google Scholar] [CrossRef]
16. Gonzales R, Arévalo L, Solis R. Shade management and pruning in two coffee varieties vs. plant growth and leaf rust in the Peruvian Amazon. Bioagro. 2023;35(1):49–58. doi:10.51372/bioagro351.6. [Google Scholar] [CrossRef]
17. Cardeña-Basilio I, Ramírez-Valverde B, Juárez-Sánchez JP, de la Peña AH, Cruz-León A. Coffee rust in Hueytamalco, Puebla. Rev Mexicana Cienc Agric. 2023;14(29):e3540 (In Spanish). doi:10.29312/remexca.v14i29.3540. [Google Scholar] [CrossRef]
18. Koutouleas A. Coffee leaf rust: wreaking havoc in coffee production areas across the tropics. Plant Health Cases. 2023;1–12. doi:10.1079/planthealthcases.2023.0005. [Google Scholar] [CrossRef]
19. Gichuru E, Alwora G, Gimase J, Kathurima C. Coffee leaf rust (Hemileia vastatrix) in Kenya—A review. Agronomy. 2021 Dec 1;11(12):1–9. [Google Scholar]
20. Gullino ML. Coffee rust in Ceylon: why English people drink tea. In: Spores. 1st ed. Switzerland: Springer Nature; 2021. p. 29–32. [Google Scholar]
21. Mccook S. The devourer of dreams. In: Coffee Is Not Forever: A Global History of the Coffee Leaf Rust. 1st edition. Ohio, Athens: Ohio University Press; 2019. p. 1–16. [Google Scholar]
22. McCook S. Global rust belt: Hemileia vastatrix and the ecological integration of world coffee production since 1850. J Glob Hist. 2006;1(2):177–95. doi:10.1017/S174002280600012X. [Google Scholar] [CrossRef]
23. RoyChowdhury M, Sternhagen J, Xin Y, Lou B, Li X, Li C. Evolution of pathogenicity in obligate fungal pathogens and allied genera. PeerJ. 2022 Aug 25;10:e13794. doi:10.7717/peerj.13794 [Google Scholar] [PubMed] [CrossRef]
24. Silva MDC, Guerra Guimarães L, Diniz I, Loureiro A, Azinheira H, Pereira AP, et al. An overview of the mechanisms involved in coffee-Hemileia vastatrix interactions: plant and pathogen perspectives. Agronomy. 2022 Feb 1;12(2):1–30. [Google Scholar]
25. Kolmer JA, Ordonez ME. The rust fungi. In: Encyclopedia of Life Sciences (ELS). Chichester: John Wiley & Sons; 2009. p. 1–8. [Google Scholar]
26. Alvarado-Huamán L, Borjas-Ventura R, Castro-Cepero V, García-Nieves L, Jiménez-Dávalos J, Julca-Otiniano A, et al. Dynamics of severity of coffee leaf rust (Hemileia vastatrix) on coffee, in Chanchamayo (Junin-Peru). Rev Agron Mesoam. 2020;31(3):517–29. doi:10.15517/am.v31i3.39726. [Google Scholar] [CrossRef]
27. Haddad F, Saraiva RM, Mizubuti ESG, Romeiro RS, Maffia LA. Isolation and selection of Hemileia vastatrix antagonists. Eur J Plant Pathol. 2014;139(4):763–72. doi:10.1007/s10658-014-0430-9. [Google Scholar] [CrossRef]
28. Belachew K, Senbeta GA, Garedew W, Barreto RW, Del Ponte EM. Altitude is the main driver of coffee leaf rust epidemics: a large-scale survey in Ethiopia. Trop Plant Pathol. 2020 Oct 1;45(5):511–21. doi:10.1007/s40858-020-00383-4. [Google Scholar] [CrossRef]
29. Mora E, Cauwelaert V, Li K, Hajian-Forooshani Z, Vandermeer J, Benítez M. Interplay between harvesting, planting density and ripening time affects coffee leaf rust dispersal and infection. bioRxiv; 2023. doi: 10.1101/2023.10.31.564961. [Google Scholar] [CrossRef]
30. Avelino J, Gagliardi S, Perfecto I, Isaac ME, Liebig T, Vandermeer J, et al. Tree effects on coffee leaf rust at field and landscape scales. Plant Dis. 2023 Feb 1;107(2):247–61. doi:10.1094/PDIS-08-21-1804-FE [Google Scholar] [PubMed] [CrossRef]
31. Resende MLV, Pozza EA, Reichel T, Botelho DMS. Strategies for coffee leaf rust management in organic crop systems. Agronomy. 2021;11(9):1–14. doi:10.3390/agronomy11091865. [Google Scholar] [CrossRef]
32. Honorato Júnior J, Zambolim L, Aucique-Pérez CE, Resende RS, Rodrigues FA. Photosynthetic and antioxidative alterations in coffee leaves caused by epoxiconazole and pyraclostrobin sprays and Hemileia vastatrix infection. Pestic Biochem Physiol. 2015;123:31–9. doi:10.1016/j.pestbp.2015.01.016 [Google Scholar] [PubMed] [CrossRef]
33. Vijayakumar MD, Surendhar GJ, Natrayan L, Patil PP, Ram PMB, Paramasivam P. Evolution and recent scenario of nanotechnology in agriculture and food industries. J Nanomater. 2022;2022:1–17. doi:10.1155/2022/1280411. [Google Scholar] [CrossRef]
34. Zhou Y, Wu J, Zhou J, Lin S, Cheng D. pH-responsive release and washout resistance of chitosan-based nano-pesticides for sustainable control of plumeria rust. Int J Biol Macromol. 2022;222:188–97. doi:10.1016/j.ijbiomac.2022.09.144 [Google Scholar] [PubMed] [CrossRef]
35. Usman M, Farooq M, Wakeel A, Nawaz A, Cheema SA, Rehman H, et al. Nanotechnology in agriculture: current status, challenges and future opportunities. Sci Total Environ. 2020;721(137778):1–16. doi:10.1016/j.scitotenv.2020.137778 [Google Scholar] [PubMed] [CrossRef]
36. Majumdar S, Keller AA. Omics to address the opportunities and challenges of nanotechnology in agriculture. Crit Rev Environ Sci Technol. 2021;51(22):2595–636. doi:10.1080/10643389.2020.1785264. [Google Scholar] [CrossRef]
37. Aime MC, Bell CD, Wilson AW. Deconstructing the evolutionary complexity between rust fungi (Pucciniales) and their plant hosts. Stud Mycol. 2018;89:143–52. doi:10.1016/j.simyco.2018.02.002 [Google Scholar] [PubMed] [CrossRef]
38. Fernandez D, Talhinhas P, Duplessis SB. 12 rust fungi: achievements and future challenges on genomics and host-parasite interactions. In: The mycota: Agricultural applications. Berlin, Heidelberg: Springer; 2013. p. 315–41. [Google Scholar]
39. Ecile Lorrain C, Gonc KC, Santos D, Germain H, Hecker A, Ebastien Duplessis S. Advances in understanding obligate biotrophy in rust fungi. New Phytol. 2019;222:1190–206. doi:10.1111/nph.15641 [Google Scholar] [PubMed] [CrossRef]
40. Talhinhas P, Azinheira HG, Loureiro A, Batista D, Vieira B, Tisserant E, et al. Overview of the functional virulent genome of the coffee leaf rust pathogen Hemileia vastatrix with an emphasis on early stages of infection. Front Plant Sci. 2014;5(88):1–17. doi:10.3389/fpls.2014.00088 [Google Scholar] [PubMed] [CrossRef]
41. Lam-Gutiérrez A, Winkler R, Garrido-Ramírez ER, Rincón-Rosales R, Gutiérrez-Miceli FA, Peña-Ocaña BA, et al. Antifungal activity of root extracts from baccharis salicina on germination of uredospores of Hemileia vastatrix. Int J Agric Biol. 2021;25(5):1075–84. doi:10.17957/IJAB/15.1766. [Google Scholar] [CrossRef]
42. Voegele RT, Hahn M, Mendgen K. The uredinales: cytology, biochemistry, and molecular biology. In: The mycota: Plant relationships. Berlin, Heidelberg: Springer; 2009. p. 69–98. [Google Scholar]
43. Mapuranga J, Zhang N, Zhang L, Chang J, Yang W. Infection strategies and pathogenicity of biotrophic plant fungal pathogens. Front Microbiol. 2022;13(799396):1–15. doi:10.3389/fmicb.2022.799396 [Google Scholar] [PubMed] [CrossRef]
44. Mapuranga J, Zhang L, Zhang N, Yang W. The haustorium: the root of biotrophic fungal pathogens. Front Plant Sci. 2022 Aug 29;13(963705):1–15. doi:10.3389/fpls.2022.963705 [Google Scholar] [PubMed] [CrossRef]
45. Avelino J, Rivas G, Rivas LG. La roya anaranjada del café. HAL; 2014. Available from: https://hal.archives-ouvertes.fr/hal-01071036. [Accessed 2022]. [Google Scholar]
46. Castillo NET, Acosta YA, Parra-Arroyo L, Martínez-Prado MA, Rivas-Galindo VM, Iqbal HMN, et al. Towards an eco-friendly coffee rust control: compilation of natural alternatives from a nutritional and antifungal perspective. Plants. 2022;11(20):1–23. doi:10.3390/plants11202745 [Google Scholar] [PubMed] [CrossRef]
47. Do Céu Silva M, Várzea V, Guerra-Guimarães L, Azinheira HG, Fernandez D, Petitot AS, et al. Coffee resistance to the main diseases: leaf rust and coffee berry disease. Braz J Plant Physiol. 2006;18(1):119–47. doi:10.1590/S1677-04202006000100010. [Google Scholar] [CrossRef]
48. Heath MC. Signalling between pathogenic rust fungi and resistant or susceptible host plants. Ann Bot. 1997;80(6):713–20. doi:10.1006/anbo.1997.0507. [Google Scholar] [CrossRef]
49. Cui L, Hanika K, Visser RGF, Bai Y. Improving pathogen resistance by exploiting plant susceptibility genes in coffee (Coffea spp.). Agronomy. 2020;10(12):1928. doi:10.3390/agronomy10121928. [Google Scholar] [CrossRef]
50. Mendgen K, Deising H. Infection structures of fungal plant pathogens—A cytological and physiological evaluation. New Phytol. 1993;124(2):193–213. doi:10.1111/j.1469-8137.1993.tb03809.x [Google Scholar] [PubMed] [CrossRef]
51. Duplessis S, Joly DL, Dodds PN. Rust effectors. In: Effectors in plant-microbe Interactions. 1st edition, Hoboken, New Jersey: John Wiley & Sons, Inc.; 2012. p. 155–93. [Google Scholar]
52. Wang M, Gao L, Dong S, Sun Y, Shen Q, Guo S. Role of silicon on plant-pathogen interactions. Front Plant Sci. 2017;8(701):1–14. doi:10.3389/fpls.2017.00701 [Google Scholar] [PubMed] [CrossRef]
53. Hou S, Liu Z, Shen H, Wu D. Damage-associated molecular pattern-triggered immunity in plants. Front Plant Sci. 2019;10(646):1–16. doi:10.3389/fpls.2019.00646 [Google Scholar] [PubMed] [CrossRef]
54. Burbano-Figueroa Ó. Plant resistance to pathogens: a review describing the vertical and horizontal resistance concepts. Rev Argent Microbiol. 2020;52(3):245–55. doi:10.1016/j.ram.2020.04.006 [Google Scholar] [PubMed] [CrossRef]
55. Patel ZM, Mahapatra R, Jampala SSM. Role of fungal elicitors in plant defense mechanism. In: Molecular aspects of plant beneficial microbes in agriculture. 1st edition. Amsterdam, Netherlands: Elsevier; 2020. p. 143–58. [Google Scholar]
56. Couttolenc-Brenis E, Carrión GL, Villain L, Ortega-Escalona F, Ramírez-Martínez D, Mata-Rosas M, et al. Prehaustorial local resistance to coffee leaf rust in a Mexican cultivar involves expression of salicylic acid-responsive genes. PeerJ. 2020;2020(1):1–21. doi:10.7717/peerj.8345 [Google Scholar] [PubMed] [CrossRef]
57. Lima Castro IS, Rossi Marques Barreiros PR, de Oliveira Mendes TA, Florez JC, de Andrade Silva EM, Neves Porto B, et al. Gene expression and interactome analysis of candidate effectors associated with pre-and post-haustorial Hemileia vastatrix-coffee interaction. J Biotechnol Biomed. 2022;5(4):198–213. doi:10.26502/jbb.2642-91280061. [Google Scholar] [CrossRef]
58. Florez JC, Mofatto LS, do Livramento Freitas-Lopes R, Ferreira SS, Zambolim EM, Carazzolle MF, et al. High throughput transcriptome analysis of coffee reveals prehaustorial resistance in response to Hemileia vastatrix infection. Plant Mol Biol. 2017;95(6):607–23. doi:10.1007/s11103-017-0676-7 [Google Scholar] [PubMed] [CrossRef]
59. Couttolenc-Brenis E, Carrión G, Villain L, Ortega-Escalona F, Mata-Rosas M, Méndez-Bravo A. Defense response to Hemileia vastatrix in susceptible grafts onto resistant rootstock of Coffea arabica L. Agronomy. 2021;11(8):1–17. doi:10.3390/agronomy11081621. [Google Scholar] [CrossRef]
60. Silva FLF, Nascimento GO, Lopes GS, Matos WO, Cunha RL, Malta MR, et al. The concentration of polyphenolic compounds and trace elements in the Coffea arabica leaves: potential chemometric pattern recognition of coffee leaf rust resistance. Food Res Int. 2020;134(109221):1–9. doi:10.1016/j.foodres.2020.109221 [Google Scholar] [PubMed] [CrossRef]
61. Melo GA, Shimizu MM, Mazzafera P. Polyphenoloxidase activity in coffee leaves and its role in resistance against the coffee leaf miner and coffee leaf rust. Phytochemistry. 2006;67(3):277–85. doi:10.1016/j.phytochem.2005.11.003 [Google Scholar] [PubMed] [CrossRef]
62. Araji S, Grammer TA, Gertzen R, Anderson SD, Mikulic-Petkovsek M, Veberic R, et al. Novel roles for the polyphenol oxidase enzyme in secondary metabolism and the regulation of cell death in walnut. Plant Physiol. 2014;164(3):1191–203. doi:10.1104/pp.113.228593 [Google Scholar] [PubMed] [CrossRef]
63. Silva MC, Guerra-Guimarães L, Loureiro A, Nicole MR. Involvement of peroxidases in the coffee resistance to orange rust (Hemileia vastatrix). Physiol Mol Plant Pathol. 2008;72(1–3):29–38. doi:10.1016/j.pmpp.2008.04.004. [Google Scholar] [CrossRef]
64. Guerra-Guimarães L, Silva MC, Struck C, Loureiro A, Nicole M, Rodrigues CJ, et al. Chitinases of Coffea arabica genotypes resistant to orange rust Hemileia vastatrix. Bio Plant. 2009;53(4):702–6. doi:10.1007/s10535-009-0126-8. [Google Scholar] [CrossRef]
65. Silva MC, Nicole M, Guerra-Guimarães L, Rodrigues CJ. Hypersensitive cell death and post-haustorial defence responses arrest the orange rust (Hemileia vastatrix) growth in resistant coffee leaves. Physiol Mol Plant Pathol. 2002;60(4):169–83. doi:10.1006/pmpp.2002.0389. [Google Scholar] [CrossRef]
66. Bent AF, Mackey D. Elicitors, effectors, and R genes: the new paradigm and a lifetime supply of questions. Annu Rev Phytopathol. 2008 Jun;45:399–436. doi:10.1146/annurev.phyto.45.062806.094427 [Google Scholar] [PubMed] [CrossRef]
67. de Wit PJGM, Mehrabi R, van den Burg HA, Stergiopoulos I. Fungal effector proteins: past, present and future: review. Mol Plant Pathol. 2009;10(6):735–47. doi:10.1111/j.1364-3703.2009.00591.x [Google Scholar] [PubMed] [CrossRef]
68. Flor HH. Current status of the gene-for-gene concept. Annu Rev Phytopathol. 1971;9(1):275–96. doi:10.1146/annurev.py.09.090171.001423. [Google Scholar] [CrossRef]
69. Herrera PJC, Alvarado AG, Cortina GHA, Combes MC, Romero GG, Lashermes P. Genetic analysis of partial resistance to coffee leaf rust (Hemileia vastatrix Berk & Br.) introgressed into the cultivated Coffea arabica L. from the diploid C. canephora species. Euphytica. 2009;167(1):57–67. doi:10.1007/s10681-008-9860-9. [Google Scholar] [CrossRef]
70. Agrios GN. Genetics of plant disease. In: Plant pathology. 5th edition. London, UK: Academic Press; 2005. p. 124–74. [Google Scholar]
71. Li L, ITOR Manuel Pinto arzea VV, Xia Q, Xiang W, Tang T, Zhu M, et al. Diseases caused by fungi and fungus-like organisms. Plant Dis. 2018;105(12):4162. doi:10.1094/PDIS-04-21-0796-PDN. [Google Scholar] [CrossRef]
72. Cabral PGC, Zambolim EM, Zambolim L, Lelis TP, Capucho AS, Caixeta ET. Identification of a new race of Hemileia vastatrix in Brazil. Australas Plant Dis Notes. 2009;4(1):129–30. doi:10.1071/DN09052. [Google Scholar] [CrossRef]
73. Luján-Hidalgo MC, Jiménez-Aguilar LA, Ruiz-Lau N, Reyes-Zambrano SJ, Gutiérrez-Miceli FA. Cambios bioquímicos en respuesta al ataque de roya en plantaciones de café. Polibotanica. 2020;25(49):1–11 (In Spanish). doi:10.18387/polibotanica.49.10. [Google Scholar] [CrossRef]
74. Koutouleas A, Collinge DB, Ræbild A. Alternative plant protection strategies for tomorrow’s coffee. Plant Pathol. 2023;72(3):409–29. doi:10.1111/ppa.13676. [Google Scholar] [CrossRef]
75. Jibat M. Review on resistance breeding methods of coffee leaf rust in Ethiopia. Int J Res Agric For. 2020;7(6):32–41. [Google Scholar]
76. Joya Dávila JG, Ruíz Sesma B, Lecona Guzmán CA, Ruiz Lau N, Ruíz Valdiviezo VM, Rojas Martínez RI, et al. Field phytoprotection of Coffea arabica mother plants, disinfection and callogenesis induction. Agrociencia. 2023;57(2):1–13. [Google Scholar]
77. Daba G, Berecha G, Lievens B, Hundera K, Helsen K, Honnay O. Contrasting coffee leaf rust epidemics between forest coffee and semi-forest coffee agroforestry systems in SW-Ethiopia. Heliyon. 2022;8(e11892):1–12. doi:10.1016/j.heliyon.2022.e11892 [Google Scholar] [PubMed] [CrossRef]
78. Alwora GO, Gichuru EK. Advances in the management of coffee berry disease and coffee leaf rust in Kenya. J Renew Agric. 2014;2(1):5. doi:10.12966/jra.03.02.2014. [Google Scholar] [CrossRef]
79. Sera GH, de Carvalho CHS, de Abrahão JCR, Pozza EA, Matiello JB, de Almeida SR, et al. Coffee leaf rust in Brazil: historical events, current situation, and control measures. Agronomy. 2022;12(2):1–19. doi:10.3390/agronomy12020496. [Google Scholar] [CrossRef]
80. Vinícius S, Brinate B, Deleon Martins L, Nogueira G, Pereira Rosa G, Vieira Cunha V, et al. Copper can influences growth, disease control and production in arabica coffee trees. Aust J Crop Sci. 2015;9(7):678–83. [Google Scholar]
81. Rai M, Ingle AP, Pandit R, Paralikar P, Shende S, Gupta I, et al. Copper and copper nanoparticles: role in management of insect-pests and pathogenic microbes. Nanotechnol Rev. 2018;7(4):303–15. doi:10.1515/ntrev-2018-0031. [Google Scholar] [CrossRef]
82. Zambolim L. Current status and management of coffee leaf rust in Brazil. Trop Plant Pathol. 2016;41(1):1–8. doi:10.1007/s40858-016-0065-9. [Google Scholar] [CrossRef]
83. Honorato J, Zambolim L, do Nascimento Lopes U, Lopes UP, da Silva Silveira Duarte H. DMI and QoI fungicides for the control of coffee leaf rust. Australas Plant Pathol. 2015;44(5):575–81. doi:10.1007/s13313-015-0373-4. [Google Scholar] [CrossRef]
84. Benelmekki M, Benelmekki M. An introduction to nanoparticles and nanotechnology. In: Designing hybrid nanoparticles. Morgan & Claypool Publishers; 2015. p. 1–14. [Google Scholar]
85. Acharya A, Pal PK. Agriculture nanotechnology: translating research outcome to field applications by influencing environmental sustainability. NanoImpact. 2020;19:100232. doi:10.1016/j.impact.2020.100232. [Google Scholar] [CrossRef]
86. Ijaz I, Gilani E, Nazir A, Bukhari A. Detail review on chemical, physical and green synthesis, classification, characterizations and applications of nanoparticles. Green Chem Lett Rev. 2020;13(3):59–81. doi:10.1080/17518253.2020.1802517. [Google Scholar] [CrossRef]
87. Ealias AM, Saravanakumar MP. A review on the classification, characterisation, synthesis of nanoparticles and their application. IOP Conf Ser Mater Sci Eng. 2017;263(032019):1–15. doi:10.1088/1757-899X/263/3/032019. [Google Scholar] [CrossRef]
88. Alejandro A, Navarro S, Salas BV, Salas V. Synthesis of silica nanoparticles from sodium metasilicate. Int J Nanoparticles. 2022;14(1):1–12. doi:10.1504/IJNP.2022.10044641. [Google Scholar] [CrossRef]
89. Carvalho CA, Pozza EA, de Souza PE, Pozza AAA, de Resende MLV, Porto ACM, et al. Nanoparticles in the management of brown eye spot in coffee. Eur J Plant Pathol. 2022;163(3):767–74. doi:10.1007/s10658-022-02511-z. [Google Scholar] [CrossRef]
90. Salazar-Navarro AA, Rivera-Reyna NE, González-Mendoza D. Synthesis of silica chitosan oligosaccharides nanoparticles. Agro Productividad. 2023;16(11):107–14. doi:10.32854/agrop.v16i11.2728. [Google Scholar] [CrossRef]
91. Elsharkawy M, Omara R, Mostafa Y, Alamri S, Hashem M, Alrumman S, et al. Mechanism of wheat leaf rust control using chitosan nanoparticles and salicylic acid. J Fungi. 2022;8(3):304. doi:10.3390/jof8030304 [Google Scholar] [PubMed] [CrossRef]
92. Leal FDS, Santos Neto H, Pinheiro ICL, Oliveira JM, Pozza AAA, Pozza EA. Copper and silver nanoparticles control coffee rust: decrease the quantity of sprayed active ingredients and is an alternative for sustainable coffee production. Eur J Plant Pathol. 2024;168:39–51. doi:10.1007/s10658-023-02726-8. [Google Scholar] [CrossRef]
93. El-Saadony MT, Abd El-Hack ME, Taha AE, Fouda MMG, Ajarem JS, Maodaa SN, et al. Ecofriendly synthesis and insecticidal application of copper nanoparticles against the storage pest Tribolium castaneum. Nanomater. 2020;10(587):1–16. doi:10.3390/nano10030587 [Google Scholar] [PubMed] [CrossRef]
94. Salazar-Navarro AA, Valdez-Salas B, Rivera-Reyna NE, Ail-Catzim CE, González-Mendoza D. Síntesis de nanopartículas de cobre con extracto de aloe vera para control de tribolium castaneum. In: Investigación en Ciencias Agrícolas. 1st edition. Zapopan, Mexico: Astra; 2022. p. 299–305 (In Spanish). [Google Scholar]
95. Mosquera-Sánchez LP, Arciniegas-Grijalba PA, Patiño-Portela MC, Guerra-Sierra BE, Muñoz-Florez JE, Rodríguez-Páez JE. Antifungal effect of zinc oxide nanoparticles (ZnO-NPs) on Colletotrichum sp., causal agent of anthracnose in coffee crops. Biocatal Agric Biotechnol. 2020;25(101579):1–9. doi:10.1016/j.bcab.2020.101579. [Google Scholar] [CrossRef]
96. Pavitra G, Sushila N, Sreenivas AG, Ashok J, Sharanagouda H. Biosynthesis of green silica nanoparticles and its effect on cotton aphid, aphis gossypii glover and mealybug, phenacoccus solenopsis tinsley. Int J Curr Microbiol Appl Sci. 2018;7(10):1450–60. doi:10.20546/ijcmas.2018.710.162. [Google Scholar] [CrossRef]
97. Mendez-Trujillo V, Valdez-Salas B, Carrillo-Beltran M, Curiel-Alvarez MA, Tzintzun-Camacho O, Ceceña-Duran C, et al. Green synthesis of bimetallic nanoparticles from Prosopis juliflora (Sw) DC., and its effect against cotton mealybug, Phenacoccus solenopsis (Hemiptera: Pseudococcidae). Phyton-Int J Exp Bot. 2019;88(3):269–75. doi:10.32604/phyton.2019.07316. [Google Scholar] [CrossRef]
98. Taha NA, Hamden S, Bayoumi YA, Elsakhawy T, El-Ramady H, Solberg S.Ø. Nanofungicides with selenium and silicon can boost the growth and yield of common bean (Phaseolus vulgaris L.) and control alternaria leaf spot disease. Microorganisms. 2023;11:728. doi:10.3390/microorganisms11030728 [Google Scholar] [PubMed] [CrossRef]
99. Badar R, Ahmed A, Munazir M, Asghar M, Bashir F. Wheat leaf rust control through biofabricated zinc oxide nanoparticles. Australas Plant Pathol. 2023;52(6):609–12. doi:10.1007/s13313-023-00949-1. [Google Scholar] [CrossRef]
100. Sabir S, Arshad M, Ilyas N, Naz F, Amjad MS, Malik NZ, et al. Protective role of foliar application of green-synthesized silver nanoparticles against wheat stripe rust disease caused by Puccinia striiformis. Green Process Synthesis. 2022;11(1):29–43. doi:10.1515/gps-2022-0004. [Google Scholar] [CrossRef]
101. Shahbaz M, Fatima N, Mashwani ZUR, Akram A, Haq E, Mehak A, et al. Effect of phytosynthesized selenium and cerium oxide nanoparticles on wheat (Triticum aestivum L.) against stripe rust disease. Molecules. 2022;27(23):1–20. doi:10.3390/molecules27238149 [Google Scholar] [PubMed] [CrossRef]
102. Irshad MA, Nawaz R, Zia ur Rehman M, Imran M, Ahmad J, Ahmad S, et al. Synthesis and characterization of titanium dioxide nanoparticles by chemical and green methods and their antifungal activities against wheat rust. Chemosphere. 2020;258:127352. doi:10.1016/j.chemosphere.2020.127352 [Google Scholar] [PubMed] [CrossRef]
103. Nunes MR, Agostinetto L, da Rosa CG, Sganzerla WG, Pires MF, Munaretto GA, et al. Application of nanoparticles entrapped orange essential oil to inhibit the incidence of phytopathogenic fungi during storage of agroecological maize seeds. Food Res Intern. 2024;175:113738. doi:10.1016/j.foodres.2023.113738 [Google Scholar] [PubMed] [CrossRef]
104. Choudhary A, Salar RK, Thakur R. Synthesis, characterization and insecticidal activity of Mentha spicata essential oil loaded polymeric nanoparticles. Biocatal Agric Biotechnol. 2024;55:102989. doi:10.1016/j.bcab.2023.102989. [Google Scholar] [CrossRef]
105. Caetano ARS, Cardoso MG, Resende MLV, Chalfuon SM, Martins MA, Gomes HG, et al. Antifungal activity of poly(ε-caprolactone) nanoparticles incorporated with Eucalyptus essential oils against Hemileia vastatrix. Lett Appl Microbiol. 2022;75(4):1028–41. doi:10.1111/lam.13782 [Google Scholar] [PubMed] [CrossRef]
106. Gomes DG, Sanada K, Pieretti JC, Shigueoka LH, Sera GH, Seabra AB, et al. Nanoencapsulation boosts the copper-induced defense responses of a susceptible Coffea arabica cultivar against Hemileia vastatrix. Antibiot. 2023;12(2):1–22. doi:10.3390/antibiotics12020249 [Google Scholar] [PubMed] [CrossRef]
107. Ray MK, Mishra AK, Mohanta YK, Mahanta S, Chakrabartty I, Kungwani NA, et al. Nanotechnology as a promising tool against phytopathogens: a futuristic approach to agriculture. Agriculture. 2023;13(9):1–41. doi:10.3390/agriculture13091856. [Google Scholar] [CrossRef]
108. Correa MG, Martínez FB, Vidal CP, Streitt C, Escrig J, de Dicastillo CL. Antimicrobial metal-based nanoparticles: a review on their synthesis, types and antimicrobial action. Beilstein J Nanotechnol. 2020;11:1450–69. doi:10.3762/bjnano.11.129 [Google Scholar] [PubMed] [CrossRef]
109. Kanakari E, Dendrinou-Samara C. Fighting phytopathogens with engineered inorganic-based nanoparticles. Mat. 2023 Mar 1;16(6):1–29. doi:10.3390/ma16062388 [Google Scholar] [PubMed] [CrossRef]
110. Valdez-Salas B, Beltrãn-Partida E, Zlatev R, Stoytcheva M, Gonzalez-Mendoza D, Salvador-Carlos J et al. Structure-activity relationship of diameter controlled Ag@Cu nanoparticles in broad-spectrum antibacterial mechanism. Mater Sci Eng C Mater Biol Appl. 2021;119:111501. doi:10.1016/j.msec.2020.111501 [Google Scholar] [PubMed] [CrossRef]
111. Naidu S, Pandey J, Mishra LC, Chakraborty A, Roy A, Singh IK, et al. Silicon nanoparticles: synthesis, uptake and their role in mitigation of biotic stress. Ecotoxicol Environ Saf. 2023;255(114783):1–13. doi:10.1016/j.ecoenv.2023.114783 [Google Scholar] [PubMed] [CrossRef]
112. Bakhtiari M, Raeisi Sadati F, Raeisi Sadati SY. Foliar application of silicon, selenium, and zinc nanoparticles can modulate lead and cadmium toxicity in sage (Salvia officinalis L.) plants by optimizing growth and biochemical status. Environ Sci Pollut Res. 2023;30(18):54223–33. doi:10.1007/s11356-023-25959-w [Google Scholar] [PubMed] [CrossRef]
113. Wang X, Xie H, Wang P, Yin H. Nanoparticles in plants: uptake, transport and physiological activity in leaf and root. Mat. 2023;16(8):1–21. doi:10.3390/ma16083097 [Google Scholar] [PubMed] [CrossRef]
114. Ullah H, Li X, Peng L, Cai Y, Mielke HW. In vivo phytotoxicity, uptake, and translocation of PbS nanoparticles in maize (Zea mays L.) plants. Sci Total Environ. 2020;737(139558):1–11. doi:10.1016/j.scitotenv.2020.139558 [Google Scholar] [PubMed] [CrossRef]
115. Lv Z, Sun H, Du W, Li R, Mao H, Kopittke PM. Interaction of different-sized ZnO nanoparticles with maize (Zea maysaccumulation, biotransformation and phytotoxicity. Sci Total Environ. 2021;796(148927):1–11. doi:10.1016/j.scitotenv.2021.148927 [Google Scholar] [PubMed] [CrossRef]
116. Zia-ur-Rehman M, Anayatullah S, Irfan E, Hussain SM, Rizwan M, Sohail MI, et al. Nanoparticles assisted regulation of oxidative stress and antioxidant enzyme system in plants under salt stress: a review. Chemosphere. 2023;314(137649):1–15. doi:10.1016/j.chemosphere.2022.137649 [Google Scholar] [PubMed] [CrossRef]
117. Ahmed MH, Omran ZS. Effect of CuO nanoparticles on seed germination and seedling growth in Echinacea purpurea in vitro. Iraqi J Agric Sci. 2024;55:34–42. doi:10.36103/ijas.v55iSpecial.1883. [Google Scholar] [CrossRef]
118. Malandrakis AA, Kavroulakis N, Avramidou M, Papadopoulou KK, Tsaniklidis G, Chrysikopoulos CV. Metal nanoparticles: phytotoxicity on tomato and effect on symbiosis with the Fusarium solani FsK strain. Sci Total Environ. 2021;787:1–10. doi:10.1016/j.scitotenv.2021.147606 [Google Scholar] [PubMed] [CrossRef]
119. Anwar N, Mehmood A, Ahmad KS, Hussain K. Biosynthesized silver nanoparticles induce phytotoxicity in Vigna radiata L. Physiol Mol Biol Plants. 2021;27(9):2115–26. doi:10.1007/s12298-021-01073-4 [Google Scholar] [PubMed] [CrossRef]
120. Ozuna C, Mulík S, Valdez-Rodríguez B, del Abraham-Juárez MR, Fernández-López CL. The effect of organic farming on total phenols, total flavonoids, brown compounds and antioxidant activity of spent coffee grounds from Mexico. Biol Agric Hortic. 2020;36(2):107–18. doi:10.1080/01448765.2019.1704876. [Google Scholar] [CrossRef]
121. Jiménez-Ortega AD, Aguilar Ibarra A, Galeana-Pizaña JM, Núñez JM. Changes over time matter: a cycle of participatory sustainability assessment of organic coffee in Chiapas, Mexico. Sustainability. 2022;14(4):1–20. doi:10.3390/su14042012. [Google Scholar] [CrossRef]
122. Naik BJ, Kim SC, Seenaiah R, Basha PA, Song EY. Coffee cultivation techniques, impact of climate change on coffee production, role of nanoparticles and molecular markers in coffee crop improvement, and challenges. J Plant Biotechnol. 2021;48(4):207–22. doi:10.5010/JPB.2021.48.4.207. [Google Scholar] [CrossRef]
123. Singh RP, Handa R, Manchanda G. Nanoparticles in sustainable agriculture: an emerging opportunity. J Control Release. 2021;329:1234–48 [Google Scholar] [PubMed]
Cite This Article
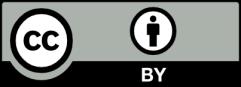
This work is licensed under a Creative Commons Attribution 4.0 International License , which permits unrestricted use, distribution, and reproduction in any medium, provided the original work is properly cited.