Open Access
ARTICLE
Photosynthetic Gas Exchange and Nitrogen Assimilation in Green Bean Plants Supplied with Two Sources of Silicon
Department of Plant Physiology, Centro de Investigación en Alimentación y Desarrollo, Ciudad Delicias, 33089, México
* Corresponding Author: Esteban Sánchez. Email:
(This article belongs to the Special Issue: Biofertilizers and Nano Fertilizers as Tools to Increase Agriculture Resilience and Sustainability)
Phyton-International Journal of Experimental Botany 2024, 93(5), 963-980. https://doi.org/10.32604/phyton.2024.048742
Received 17 December 2023; Accepted 08 April 2024; Issue published 28 May 2024
Abstract
Beans contain a wide range of vitamins, proteins, calcium, and zinc which make them an important food source for many countries. To meet the demand for bean production worldwide, large amounts of fertilizers and pesticides are used. However, the cost of production and environmental impact increases. To produce food sustainably, the use of beneficial nutrients such as silicon as a biostimulant has been proposed. However, information about the effect of different sources of silicon on the metabolism of bean plants is scarce. Bean plants cv. Strike were grown in pots for 60 days and the effect of foliar application of silicon nanoparticles and the silicon-based biostimulant Codasil® at 4 concentrations (0, 1, 2, and 4 mM) on total biomass, yield, photosynthetic pigment concentration, photosynthetic activity, stomatal conductance, transpiration rate, chlorophyll fluorescence, and nitrogen assimilation were evaluated. The results obtained showed that the supply of silicon at a dose of 1 mM functioned as a biostimulant, favoring gas exchange and nitrogen assimilation within the plant, which stimulated growth and yield. The results of this research work allowed a better comprehension of the effects of silicon application through silicon nanoparticles and the biostimulant Codasil® on the physiology of green bean plants.Keywords
Beans are an important source of protein, vitamins calcium, magnesium, and zinc. Because of this, they are a significant food source, mainly for developing countries [1]. Additionally, green beans (Phaseolus vulgaris L.) contain several bioactive compounds: phenolic compounds, lectins, oligosaccharides, and flavonoids that help prevent cardiovascular diseases, obesity, diabetes, and cancer [2]. These properties make beans the most important legume for human consumption [3]. However, to meet production demands, large amounts of fertilizers and pesticides are used, the increasing cost of production and environmental impact [4]. Hence, one of the objectives of modern agriculture is to sustainably produce the adequate quantity and quality of food [5]. An alternative for sustainable production is the use of biostimulants and nanoparticles of different elements, which improve crop productivity, efficient use of nutrients, and tolerance against biotic (plant pathogens, pests) and abiotic stresses (drought, salinity, heavy metal, extreme temperatures) [6,7].
A plant biostimulant is any product that can stimulate vegetative growth, and yield and can ameliorate the harmful effects of stress [8]. They contain a wide array of molecules, compounds, and nutrients including Zn, Si, vitamins, amino acids, and plant hormones [9,10]. Their complex chemical nature promotes growth by regulating plant physiological processes, notably, biochemical pathways and regulatory systems within the plant. Examples of such processes include seed germination, photosynthesis, nutrient absorption, and antioxidant systems [11,12]. In addition, a key factor in the use of biostimulants is the significant effects on crops when applied in small quantities, which differentiates them from agrochemicals and fertilizers [13].
Similarly, nanotechnology is an emerging and promising tool to stimulate plant growth and development by influencing metabolic processes such as transpiration and photosynthesis [14]. The small size of nanoparticles (1–100 nm), porosity, high surface area-to-weight ratio, and high reactivity of their surface make them readily available for plant uptake [12,15]. Furthermore, they can serve as delivery systems for other particles (nutrients) in a controlled manner [7]. It also has been reported that nanoparticles improve resilience to biotic and abiotic stress [16]. Therefore, nanoparticles could be employed in precision agriculture, delivering in a precise and controlled manner the necessary inputs for the correct development of plants, offering greater productivity, efficiency, and economic benefits.
Specifically, the application of silicon (Si) in the form of nanoparticles (NanoSi) is of particular interest, owing to the considerable benefits of Si in plant metabolism, particularly in coping with biotic and abiotic stresses [17]. Nonetheless, Si accumulation capacity is directly linked to beneficial effects on the plant, in addition to the fact that the amount of Si hoarded is dependent on the stress level of the plant [18]. Nanoparticle nutrition is a relatively new area of plant mineral nutrition. For example, Si uptake is species and Si transporter-dependent, making Si fertilization less effective in certain species compared to others [19]. Certainly, information on how different Si sources can affect the basic physiological parameters of green beans such as photosynthetic gas exchange and nitrogen metabolism parameters is scarce. The purpose of this study was to assess the effects of NanoSi and the biostimulant Codasil at 4 doses each (0,1, 2, and 4 mM) on total biomass, yield, photosynthetic gas exchange parameters, and nitrogen assimilation parameters.
The experiment was established under shade net conditions in Cd. Delicias, Chihuahua, Mexico (28°11′ 36′′ N, 105°28′16′′ W) during the period August–October 2022. A shade net was necessary to protect the green bean plants from the high solar radiation (1100 W m−2) present in the region. Green bean seeds (Phaseolus vulgaris L.) cv. strike was sown in plastic pots (13.4 L capacity). These pots contained a mixture of vermiculite and perlite in a 2:1 weight/weight ratio as substrate (Two plants per pot). Two plants per pot were used because some analyses were carried out with dry material and others required freshly harvested material. A modified Hoagland solution by Sánchez et al. [20] was used for plant irrigation and composed as follows: 6 mM NH4NO3, 1.6 mM K2HPO4, 0.3 mM K2SO4, 4 mM CaCl2, 1 µM ZnSO4, 5 µM Fe-EDDHA, 2 µM MnSO4, 0.25 µM CuSO4, 0.3 µM Na2MoO4, 0.5 µM H3BO with pH of 6.0–6.1 and electrical conductivity of 2 dS m−1. This solution was applied at a volume of 0.5 L per pot per day until 30 days after sowing, whereafter one L of solution was applied every third day until harvest (60 days after sowing).
2.2 Experimental Desing and Treatments
Seven treatments with six replicates each were distributed in a completely randomized experimental design (Table 1): NanoSi and Codasil® at doses of 1, 2, and 4 mM, with 0 mM being the control for both; these doses were based on previous reports, although they were slightly higher in this experiment [19,21]. Randomization was achieved by Excel’s random number generator.
All treatments were randomly foliar sprayed at evening once the first true leaves appeared, and subsequently every week for a period of two months.
The chemical compositions of the treatments used in this research are presented below (Table 2).
Plants were sampled 60 days after sowing when plants were physiologically mature and with ripe fruit. After harvest, plants were washed to remove environmental residues.
Biomass accumulation was determined from the average weight per plant. Fresh plant material was oven dried (Shell) at 70°C for 24 h until constant weight. Subsequently, total biomass production was quantified with an analytical balance (AND HR-120, San Jose, California, USA), and expressed as grams of plant dry weight (g plant−1 d.w.).
Yield was obtained as the average fruit weight (AND HR-120, San Jose, California, USA) and reported as the average plant gram fresh weight (g plant−1 f.w.).
2.4.3 Photosynthetic Gas Exchange
Readings of gas exchange variables were performed with a LI-COR 6400 portable meter on each plant, choosing healthy and undamaged leaves. A concentration of 400 µmol/mol CO2 was used for the reference cell, while a concentration of 380 µmol/mol CO2 was used for the sample cell. The vapor pressure deficit of the air in the sampling chamber was less than 1.5 kPa and the temperature of the leaf storage block was 30°C. Photosynthetic activity was expressed as μmol CO2 m−2 s−1 and transpiration rate in mmol H2O m−2 s−1, stomatal conductance as mol CO2 m2 s−1, photosystem II efficiency in Fv/Fm and transpiration rate in mmol H2O m−2 s−1.
The concentration of photosynthetic pigments was determined by the method proposed by Wellburn et al. [22], where 0.12 g of fresh leaves were weighed in the form of 7 mm diameter discs. Subsequently, 10 mL of methanol was added to the samples and were incubated at room temperature in darkness for 24 h. The absorbance was then measured at 470 nm (carotenoids), 653 nm (chlorophyll b, Chl b), and 666 nm (chlorophyll a, Chl a). photosynthetic pigment concentrations were expressed in µg cm−2 and calculated using the following formulas:
2.4.5 “In vivo” Nitrate Reductase Activity
Nitrate reductase (NR) enzyme activity (EC 1.6.6.1) was determined by adapting the methodology proposed by Sánchez et al. [20], and Maurino et al. [23]. Discs of 7 mm diameter were obtained from the leaf blade of fresh material, weighing between 0.125–0.150 g, and placed in 10 mL of filtration medium consisting of potassium phosphate buffer (100 mM, pH 7.5% and 1% v/v propanol). Subsequently, the tissues were subjected to low pressure (0.8 bar) for 10 min in absence of light, and thereafter incubated at 30°C in darkness for 1 h. To stop NR activity, the samples were immersed in a hot water bath (100°C) for 15 min. After this, NR activity was performed with 1 mL aliquot, 2 mL of sulfanilamide at 1% dissolved in 1.5 M HCl (1 g sulfanilamide + 20 mL 35% HCl dissolved in 100 mL water), and 2 mL of 0.02% NNEDA (N-1-naphthyl ethylenediamine) dissolved in 0.2 M HCl (20 g NNEDA dissolved in 100 mL 0.2 M HCl). After 20 min, absorbance readings were taken at 540 nm, against a NO2− standard curve between 0.025–2 µg mL−1, as proposed by Hagelman and Hucklesby [24]. NR activity was reported as µmol of NO2− formed per g f.w.−1 h−1.
For the determination of amino acids, the method proposed by Yemm et al. [25], was used with the ninhydrin reagent with slight modifications. Approximately 0.5 g of plant material was weighed and homogenized with 5 mL of 50 mM phosphate buffer pH 7.0. After this, a mesh screen filtered the homogenate and was centrifuged at 12360 g for 15 min. The supernatant was used for the quantification of amino acids and soluble proteins. Subsequently, 100 µL of supernatant was placed in a test tube with 1.5 mL of ninhydrin reagent. The mixture was placed in a hot water bath at 100°C for 20 min. A glycine curve was prepared (in the same manner as the sample). After 20 min, the samples were transferred to a container with ice. Then, 8 mL of 50% propanol was added to each of the sample tubes and the curve tubes and left to stand for 30 min. Subsequently, they were read at a wavelength of 570 nm, against the glycine standard curve. The concentration of amino acids and soluble proteins was expressed as mg g−1 f.w.
Soluble proteins were determined after homogenization of 0.5 g of fresh sample in cold 50 mM KH2PO buffer at pH 7 and centrifugation of 12360 g, at 4°C for 15 min. The supernatant was also used for amino acid determination. The method used was that proposed by Bradford [26]. 1 mL of Bradford’s reagent was taken in a test tube and 20 µL of bovine albumin (BSA) was added from each of the tubes with known concentration, corresponding to each point of the curve. Subsequently, it was read at an absorbance of 595 nm against the standard curve. Soluble proteins were expressed as mg g−1 f.w.
The data obtained were subjected to an analysis of variance and a mean separation test by the least-significant difference (LSD) method (95%), using SAS statistical software (SAS Inst. Inc. Cary, NC, USA). The data presented are mean values ± standard error.
The application of 1- and 4-mM doses of both NanoSi and Codasil had beneficial effects on total biomass accumulation. The NanoSi4 treatment presented the highest total biomass accumulation concerning the control (no Si application), with an increase of 16%, although no significant differences were found with NanoSi1, Codasil1, and Codasil4 (Fig. 1).
Figure 1: Effects of two silicon sources on total biomass in green beans cv. Strike. Different letters present statistical differences between them (LSD p ≤ 0.05). The application of both sources of Si was beneficial for the accumulation of biomass
In the present study, application of NanoSi and Codasil improved yields, except for Codasil1 and 2. The NanoSi2 treatment showed the highest yield, with a 36% increase over the control (Fig. 2).
Figure 2: Effects of two silicon sources on yield in green beans cv. Strike. Different letters present statistical differences between them (LSD p ≤ 0.05). Yield was improved by the application of NanoSi and Codasil
In the present study, the foliar application of silicon was beneficial for photosynthetic activity, where the NanoSi1 treatment showed the highest activity compared to the control, with an increase of 50% (Fig. 3).
Figure 3: Effects of two silicon sources on photosynthetic activity in green beans cv. Strike. Different letters present statistical differences between them (LSD p ≤ 0.05). Photosynthetic activity was enhanced by NanoSi1 application
In the present study, the NanoSi treatment was superior to the other treatments except for the control, with which it did not present significant differences although it had a 10% increase (Fig. 4).
Figure 4: Effects of two silicon sources on Stomatal conductance in green beans cv. Strike. Different letters present statistical differences between them (LSD p ≤ 0.05). Stomatic conductance was improved by NanoSi1 application, although with no significant difference with the control plants
In the present study, Codasil1 exhibited the lowest transpiration rates compared to the rest of treatments (Fig. 5). This treatment reduced the transpiration rate by 32% compared to the control group.
Figure 5: Effects of two silicon sources on transpiration rates in green beans cv. Strike. Different letters present statistical differences between them (LSD p ≤ 0.05). Transpiration rates were reduced with the application of Codasil and higher doses of NanoSi
The Codasil1 treatment presented the best Fv/Fm ratio, with an increase of 21% compared to the control, although it was not significantly different from the NanoSi1 and NanoSi4 groups (Fig. 6). With higher doses of each treatment, a decline in the Fv/Fm values is observed.
Figure 6: Effects of two silicon sources on chlorophyll fluorescence in green beans cv. Strike. Different letters present statistical differences between them (LSD p ≤ 0.05). Chlorophyll fluorescence was enhanced by the lowest doses of NanoSi and Codasil
Total chlorophyll concentration was higher in the NanoSi1 treatment, with an increase of 48% over the control (Fig. 7). A progressive increase in doses of both treatments results in a downward trend of chlorophyll concentrations.
Figure 7: Effects of two silicon sources on total chlorophyll in green beans cv. Strike. Different letters present statistical differences between them (LSD p ≤ 0.05). Chlorophyll contents were increased with the lowest doses of each treatment and a downward trend was observed with the higher doses of each treatment
3.8 Nitrate Reductase Activity
In the present work, the application of Codasil2 increased nitrate reductase (NR) enzyme activity by 191% compared to the control (Fig. 8), although no significant differences were found with the rest of the treatments. NR activity declines with progressive increases in NanoSi doses.
Figure 8: Effects of two silicon sources on nitrate reductase activity in green beans cv. Strike. Different letters present statistical differences between them (LSD p ≤ 0.05). Codasil increased the NR activity of bean plants
In the present study, the Codasil2 treatment presented an increase in the soluble amino acid concentration of 28% concerning the control, although this difference was not significant compared to the rest of the treatments, except the Codasil4 treatment (Fig. 9). A downward trend is observed the highest dose of each treatment.
Figure 9: Effects of two silicon sources on soluble amino acids in green beans cv. Strike. Different letters present statistical differences between them (LSD p ≤ 0.05). Amino acid concentration was increased with NanoSi and Codasil
Soluble protein concentration was higher in the Codasil1 treatment, with an increase of 38%, concerning the control (Fig. 10). However, the Codasil1 treatment was not significantly different from the NanoSi4, NanoSi2, and Codasil2 treatments.
Figure 10: Effects of two silicon sources on soluble proteins in green beans cv. Strike. Different letters present statistical differences between them (LSD p ≤ 0.05)
In general, the application of both silicon sources had beneficial effects on plant metabolism. However, NanoSi enhanced yield, biomass accumulation, photosynthetic activity, stomatic conductance a photosynthetic pigment concentration. Whereas Codasil improved transpiration rates, chlorophyll fluorescence, nitrate reductase activity, and soluble amino acid and protein contents (Fig. 11).
Figure 11: Graphic abstract of the parameters affected by exogenous application of NanoSi and Codasil on green beans cv. Strike
Biomass accumulation is dependent on plant photosynthetic activity, and its distribution to reproductive organs plays a key role in obtaining higher yields [27,28]. Several authors have reported biomass increase in a variety of conditions, such as drought and salinity in crops such as strawberries and beans at Si doses of 0.5 1 and 1.5 mM [11,21]. Furthermore, the beneficial effects of Si enhanced biomass accumulation in sweet basil by improving photosynthesis, photosystem II efficiency, stomatic conductance, electron transport rate, and photochemical quenching [29]. Biomass increase was also observed in soybean grown under cold stress and without cold stress [30]. Cold stress raises reactive oxygen species levels and upregulates the antioxidant systems to prevent oxidative stress damage. In addition, stress-signaling genes sharply increase ABA levels in response to stress. In our study, it may be possible that Si influenced gene expression of amino acids or proteins involved in photosynthesis, nutrient uptake, transpiration, hormone production, and reinforcement of the antioxidant system [31–33]. In this experiment, the increased efficiency and/or regulation of these processes results could increase biomass accumulation for the plant.
Crop yield responds to the interaction between the genotype and the environment [34]. The small size of NanoSi makes them readily available for plant uptake and has a favorable impact on the nutritional balance and physiological activities of the plant which improves its growth and, therefore, its yield [35]. Indeed, studies in soybean, grapevine, bean, and strawberry have shown the influence of silicon in stimulating physiological processes such as photosynthesis, transpiration regulation, nutrient uptake, and resistance to both biotic and abiotic stresses which increases yield [36–38]. In a recent study done on wheat, Si supplementation increased yield not only in drought conditions but also in well-irrigated plants [39]. In this study, yield was improved by enhanced antioxidant systems, higher chlorophyll contents, and buildup of photoassimilates. Moreover, exogenous application of NanoSi in water-stressed potatoes increased yield by optimizing water use efficiency and leaf gas exchange [40].
Photosynthesis is the main way to obtain energy to drive plant growth and yield, let alone the generation of the oxygen necessary for the rest of living beings [41]. That is why the optimization of such a process is essential to obtain higher yields. These results are those reported by Mahmoud et al. [42], in potatoes, where the application of NanoSi resulted in improved stomatal conductance and increased chlorophyll content in the leaves. In our case, a boosted stomatal conductance allows for increased intracellular CO2, better light absorption, and improved photosystem II quantum yield. Mukarram et al. [32] also reported that photosynthesis in Cymbopogon flexuosus was enhanced by NanoSi due to improved photochemical quenching and electron transport rate. The antioxidant system of Cymbopogon flexuosus was also strengthened by accelerating enzymatic superoxide dismutase (SOD), peroxidase (POD), catalase (CAT), and non-enzymatic (ascorbic acid and GSH) antioxidant activity, limiting cell damage by lipid peroxidation. This result was also observed in wheat [43]. Another reported benefit is the increase in the size of chloroplasts, greater number of grana in leaves, and leaf erectness due to silicon deposition allowing for better light absorption, which increases the efficiency of photosynthetic activity [44–46].
Stomatic conductance modulates gas diffusion between the leaf and the atmosphere, regulating CO2 assimilation, water loss, and evaporative cooling [47]. Stomata are in a continuous cycle of opening and closing in response to external and internal signals, such as light or hormones to maintain a balance between CO2 assimilation and water loss by transpiration [48]. Although NanoSi1 showed no significant difference from the control treatment, the enhanced stomatal conductance could be due to a change in cell wall plasticity and elasticity which facilitates the passage of CO2 between the environment and the plant [32]. The increased passage of CO2 further boosts photosynthesis and increases yield as mentioned before. NanoSi also enhanced stomatal conductance in Cymbopogon flexuosus growing under salinity conditions, improving CO2 assimilation and reducing transpiration rates [49]. Previous studies have also indicated that Si supplementation can form a cuticle double layer in the leaf reducing transpiration and maintaining water status within the plant [50]. In addition, exogenous silicon application could improve stomatal conductance by increasing the sensitivity of stomata to K+ flux, an element that is also related to stomata opening and closing, reducing water loss and increasing CO2 uptake [51,52].
The movement of water throughout the plant is a physiological process known as transpiration. Transpiration rates are influenced by distinct biophysical drivers (temperature, water vapor pressure deficit, and net radiation) and stomatal conductance [53]. The Codasil treatments decreased transpiration through the stomata, thus increasing water use efficiency. One of the components of Codasil is K+ which participates in osmotic regulation, aquaporins functioning, and maintenance of cell turgor pressure as documented in water-stressed lettuce [54,55]. In addition, the free amino acids also present in Codasil could function as osmolytes regulating osmotic potential in the cells and stomatal opening as noted by Abdelkader et al. [56] when they applied exogenous amino acids to salt-stressed lettuce. Another study applied an amino acid-based biostimulant in drought-stressed soybean reported that the accumulation of soluble sugars also has a key role in maintaining osmotic balance under such conditions [57]. It is also possible that Si may upregulate the expression of aquaporins in green bean, as was reported in sorghum [58]. As such, more efficient water movement along with better nutrient uptake improves performance in all physiological activities of plant cells.
When light is absorbed by chlorophyll, the energy assimilated can be used to drive photosynthesis, dissipate as heat, or re-emit as light (fluorescence). Thus, fluorescence is an indicator of plant photosynthetic performance, where a decrease in the Fv/Fm index represents a reduction in photosystem II efficiency, especially caused by photoinhibition [59]. In both treatments and at the lowest doses, high chlorophyll content together with optimized fluorescence due to enhanced cell homeostasis could further support the photosynthetic process. In the case of Codasil, the treatment reduced transpiration rates, improving the water supply to photosynthesis and increased photosynthetic efficiency. These results agree with those documented in Pinus sylvestris and Quercus robur [60]. In another study with peppermint, NanoSi improved chlorophyll fluorescence values and also noted a gradual decline of this parameter at higher concentrations [61].
Chlorophyll is the main pigment for carrying out photosynthesis and is involved in the process of light absorption, transfer, distribution, and transformation, leading to improved leaf health and crop yield [62]. These results coincide with those reported in sorghum under iron deficiency [63], where Si application increased chlorophyll concentration by facilitating Fe uptake, an element that plays an important role in chlorophyll synthesis [64]. Similarly, Si application improved Mg uptake in cold-stressed alfalfa, this additional supply of Mg could also enhance chlorophyll content [65]. Si decreased the concentration of malonaldehyde in leaves and roots, which restricted chlorophyll degradation. Moreover, Si increased chlorophyll concentration in young barley leaves, maize seedlings, and green peas by upregulating the activity of antioxidant enzymes including SOD, CAT, and guaiacol peroxidase resulting in less accumulation of reactive oxygen species [66–68]. Nonetheless, chlorophyll concentration showed a downward trend when higher doses of Si were applied. An excess of chlorophyll generates reactive oxygen species and must be removed to avoid harmful effects on plant growth [69,70]. Therefore, catabolic regulation of chlorophyll biosynthesis is essential for normal growth, although its elucidation remains incomplete. Additional studies of reactive oxygen species are required to verify this information.
The enzyme nitrate reductase (EC 1.7.1.1) is essential for nitrogen assimilation for higher plants and directly regulates nitrate reduction [71,72], where nitrate reductase activity is proportional to the amount of nitrate present, i.e., the higher the nitrate concentration, the higher the enzymatic activity of nitrate reductase [73]. These results coincide with those reported in maize seedlings and cucumber under nitrate stress, where the application of Si improved the effects of N in plants by being absorbed in greater quantities, which in turn increased the activity of nitrate reductase and therefore, had a higher metabolic activity [74,75]. In addition, the application of biostimulants with bioactive molecules such as amino acids could have a positive effect on nitrate reduction by down-regulating nitrate transporter genes, as well as, increasing the transcription levels of related genes in the nitrogen metabolic pathway [76,77]. However, the addition of NanoSi at higher doses (2 and 4 mM) together with the addition of 1 mM KNO3, decreased nitrate reductase activity. Concerning this decrease, there may be a limit to the nitrate concentration that regulates nitrate reductase enzyme activity. Hao et al. [78] documented that high doses of NanoSi applied to maize hampered nitrogen metabolism by inhibiting nitrogen assimilation or by breaking down nitrogen metabolites due to catabolism. In their study, they found that activities of key enzymes including glutamine synthetase (GS), glutamate dehydrogenase (GDH), and glutamate synthase (GOGAT) were reduced by these high doses, thus affecting plant growth. In our experiment, only NR activity was measured and the study of GS, GDH, and GOGAT is necessary to further understand how high doses of silicon affect nitrogen metabolism.
Amino acids, in addition to being involved in protein synthesis, serve a variety of functions, which include precursors of hormones, signaling factors in physiological processes, root development, antioxidant metabolism, and regulators of nitrogen uptake [1,79,80]. Recent studies in water-stressed wheat documented that foliar application of Si raised soluble amino acid levels [81]. In addition, Trejo-Tellez et al. [82] also found that silicon increases soluble amino acid concentrations in pepper plants. However, foliar application of amino acids could influence nitrogen uptake and assimilation by regulating enzymes responsible for nitrogen assimilation [83]. For example, the application of amino acids (L-methionine, L-tryptophan, and L-glycine) in lettuce functions as signal transducer molecules of various beneficial physiological processes for the plant [84]. Within this group of amino acids, L-methionine induces better sulfur and nitrogen uptake when applied in low amounts [85]. It is important to note that a high concentration of L-methionine could cause stress to the plant [86], which would explain the downward trend in amino acid concentration with increasing doses of Codasil by altering processes such as photosynthesis and causing physiological disorders by interfering with normal amino acid metabolism [87]. Similarly, the application of NanoSi at higher doses could hurt amino acid accumulation.
Proteins are composed of long chains of amino acids that can perform multiple functions owing to the unique sequence of their constituent amino acids [88]. These results coincide with what has been reported in research conducted in wheat and rice, where the application of both Si in conventional form and NanoSi improved the content of soluble proteins [89]. Additionally, Sattar et al. [39] documented a surge in soluble protein content when applying exogenous Si to wheat plants under drought stress. Moreover, foliar application of Si in wheat under different water deficits (mild, moderate, and severe) increased the total soluble protein content [81]. In our study, this increase in the concentration of soluble proteins in NanoSi4 may be because amino acids are used for protein synthesis, or for better nitrogen translocation, which improves nitrogen metabolism and protein synthesis in plants. Similarly, Si could affect the synthesis of specific proteins and the efficacy of mRNA and DNA synthesis, increasing protein concentration [90].
The foliar application of Si through NanoSi and the biostimulant Codasil® was beneficial since it increased biomass accumulation and yield by influencing photosynthetic activity, stomatal conductance, transpiration rates, and chlorophyll fluorescence, as well as the accumulation of photosynthetic pigments. In addition, the application of Si stimulated nitrate reductase activity, which in turn allowed a greater accumulation of amino acids and proteins. This translates into improved nitrogen assimilation. Based on these results, the supply of silicon at low doses (1 mM) functioned as a biostimulant, favoring gas exchange and nitrogen assimilation within the plant, which stimulated growth and yields in green beans. However, further studies are necessary to understand how higher doses of NanoSi affect plant metabolism, especially in chlorophyll synthesis and catabolism, nitrate reductase, and soluble amino acids. Likewise, Codasil has beneficial effects on plant physiology due to its complex chemical nature, but the isolation of the amino acids present in Codasil will help pinpoint how these individual amino acids help plant growth. Finally, the results of this research work allowed a better understanding of the effects of silicon application through silicon dioxide nanoparticles and the biostimulant Codasil® on the physiology of the green bean plant.
Acknowledgement: None.
Funding Statement: The authors received no specific funding for this study.
Author Contributions: The authors confirm their contribution to the paper as follows: study conception and design: Sánchez E.; data collection Anchondo-Páez J.C., Ramírez-Estrada C.A.; analysis and interpretation of results: Salcido-Martínez A., Ochoa-Chaparro E.H.; draft manuscript preparation: Anchondo-Páez J.C. All authors reviewed the results and approved the final version of the manuscript.
Availability of Data and Materials: The datasets analysed during the current study are available from the corresponding author on reasonable request.
Ethics Approval: Not applicable.
Conflicts of Interest: The authors declare that they have no conflicts of interest to report regarding the present study.
References
1. Calvo P, Nelson L, Kloepper JW. Agricultural uses of plant biostimulants. Plant Soil. 2014;383:3–41. doi:10.1007/s11104-014-2131-8. [Google Scholar] [CrossRef]
2. Chávez-Mendoza C, Hernández-Figueroa KI, Sánchez E. Antioxidant capacity and phytonutrient content in the seed coat and cotyledon of common beans (Phaseolus vulgaris L.) from various regions in Mexico. Antioxid. 2019;8(1):5. doi:10.3390/antiox8010005 [Google Scholar] [PubMed] [CrossRef]
3. Uebersax MA, Cichy KA, Gomez FE, Porch TG, Heitholt J, Osorno JM, et al. Dry beans (Phaseolus vulgaris L.) as a vital component of sustainable agriculture and food security: a review. Legum Sci. 2023;5(1):e155. doi:10.1002/leg3.155. [Google Scholar] [CrossRef]
4. Elemike E, Uzoh I, Onwudiwe D, Babalola O. The role of nanotechnology in the fortification of plant nutrients and improvement of crop production. Appl Sci. 2019;9(3):499. doi:10.3390/app9030499. [Google Scholar] [CrossRef]
5. El Sheikha AF, Allam AY, Taha M, Varzakas T. How does the addition of biostimulants affect the growth, yield, and quality parameters of the snap bean (Phaseolus vulgaris L.)? How is this reflected in its nutritional value? Appl Sci. 2022;12(2):776. doi:10.3390/app12020776. [Google Scholar] [CrossRef]
6. Povero G, Mejia JF, di Tommaso D, Piaggesi A, Warrior P. A systematic approach to discover and characterize natural plant biostimulants. Front Plant Sci. 2016;7:435. doi:10.3389/fpls.2016.00435 [Google Scholar] [PubMed] [CrossRef]
7. Rastogi A, Tripathi DK, Yadav S, Chauhan DK, Živčák M, Ghorbanpour M, et al. Application of silicon nanoparticles in agriculture. 3 Biotech. 2019;9:1–11. doi:10.1007/s13205-019-1626-7 [Google Scholar] [PubMed] [CrossRef]
8. Du Jardin P. Plant biostimulants: definition, concept, main categories and regulation. Sci Hort. 2015;196:3–14. doi:10.1016/j.scienta.2015.09.021. [Google Scholar] [CrossRef]
9. Bulgari R, Franzoni G, Ferrante A. Biostimulants application in horticultural crops under abiotic stress conditions. Agronomy. 2019;9(6):306. doi:10.3390/agronomy9060306. [Google Scholar] [CrossRef]
10. Santi C, Zamboni A, Varanini Z, Pandolfini T. Growth stimulatory effects and genome-wide transcriptional changes produced by protein hydrolysates in maize seedlings. Front Plant Sci. 2017;8:433. doi:10.3389/fpls.2017.00433 [Google Scholar] [PubMed] [CrossRef]
11. Avestan S, Ghasemnezhad M, Esfahani M, Barker AV. Effects of nanosilicon dioxide on leaf anatomy, chlorophyll fluorescence, and mineral element composition of strawberry under salinity stress. J Plant Nutr. 2021;44(20):3005–19. doi:10.1080/01904167.2021.1936036. [Google Scholar] [CrossRef]
12. Shalaby TA, Abd-Alkarim E, El-Aidy F, Hamed ES, Sharaf-Eldin M, Taha N, et al. Nano-selenium, silicon and H2O2 boost growth and productivity of cucumber under combined salinity and heat stress. Ecotoxicol Environ Saf. 2021;212:111962. doi:10.1016/j.ecoenv.2021.111962 [Google Scholar] [PubMed] [CrossRef]
13. Puglia D, Pezzolla D, Gigliotti G, Torre L, Bartucca ML, Del Buono D. The opportunity of valorizing agricultural waste, through its conversion into biostimulants, biofertilizers, and biopolymers. Sustainability. 2021;13(5):2710. doi:10.3390/su13052710. [Google Scholar] [CrossRef]
14. Magda S, Hussein MM. Determinations of the effect of using silica gel and nano-silica gel against Tutaabsoluta (Lepidoptera: gelechiidae) in tomato fields. J Chem Pharm Res. 2016;8(4):506–12. [Google Scholar]
15. Solanki P, Bhargava A, Chhipa H, Jain N, Panwar J. Nano-fertilizers and their smart delivery system. Nanotech Food Agri. 2015;66:81–101. doi:10.1007/978-3-319-14024-7_4. [Google Scholar] [CrossRef]
16. Mathur P, Roy S. Nanosilica facilitates silica uptake, growth and stress tolerance in plants. Plant Physiol Biochem. 2020;157:114–27. doi:10.1016/j.plaphy.2020.10.011 [Google Scholar] [PubMed] [CrossRef]
17. Felisberto G, de Mello Prado R, de Oliveira RLL, de Carvalho Felisberto PA. Are nanosilica, potassium silicate and new soluble sources of silicon effective for silicon foliar application to soybean and rice plants? Silicon. 2021;13:3217–28. doi:10.1007/s12633-020-00668-y. [Google Scholar] [CrossRef]
18. Coskun D, Deshmukh R, Sonah H, Menzies JG, Reynolds O, Ma JF. The controversies of silicon’s role in plant biology. New Phytol. 2019;221:67–85. doi:10.1111/nph.15343 [Google Scholar] [PubMed] [CrossRef]
19. Sánchez E, Rivero RM, Ruiz JM, Romero L. Changes in biomass, enzymatic activity and protein concentration in roots and leaves of green bean plants (Phaseolus vulgaris L. cv. Strike) under high NH4NO3 application rates. Sci Hortic. 2004;99(3–4):237–48. [Google Scholar]
20. Parande S, Zamani GR, Zahan MHS, Ghaderi MG. Effects of silicon application on the yield and component of yield in the common bean (Phaseolus vulgaris) under salinity stress. Intl J Agron Plant Prod. 2013;4(7):1574–9. [Google Scholar]
21. Qados AA, Moftah AE. Influence of silicon and nano-silicon on germination, growth and yield of faba bean (Vicia faba L.) under salt stress conditions. Am J Exp Agric. 2015;5(6):509–24. [Google Scholar]
22. Wellburn AR. The spectral determination of chlorophylls a and b as well total carotenoids, using various solvents whit spectrophotometer of different resolution. J Plant Physiol. 1994;144:307–13. doi:10.1016/S0176-1617(11)81192-2. [Google Scholar] [CrossRef]
23. Maurino SG, Echevarria C, Mejias JA, Vargas MA, Maldonado JM. Properties of the in vivo nitrate reductase assay in maize, soybean, and spinach leaves. J Plant Physiol. 1986;124(1–2):123–30. doi:10.1016/S0176-1617(86)80184-5. [Google Scholar] [CrossRef]
24. Hagelman RH, Hucklesby DP. Nitrate reductase from higher plants. Method Enzymol. 1971;23:491–503. doi:10.1016/S0076-6879(71)23121-9. [Google Scholar] [CrossRef]
25. Yemm EW, Cocking EC, Ricketts RE. The determination of amino-acids with ninhydrin. Analyst. 1995;80(948):209–14. doi:10.1039/AN9558000209. [Google Scholar] [CrossRef]
26. Bradford MM. A rapid and sensitive method for the quantification of microgram quantities of protein utilizing the principle of protein-dye binding. Anal Biochem. 1976;72:248–54. doi:10.1016/0003-2697(76)90527-3. [Google Scholar] [CrossRef]
27. Gao XL, Sun JM, Gao JF, Feng BL, Wang PK, Chai Y. Accumulation and transportation characteristics of dry matter after anthesis in different mung bean cultivars. Acta Agron Sin. 2009;35(9):1715–21 (In Chinese). doi:10.3724/SP.J.1006.2009.01715. [Google Scholar] [CrossRef]
28. Plénet D, Mollier A, Pellerin S. Growth analysis of maize field crops under phosphorus deficiency. II. Radiation-use efficiency, biomass accumulation and yield components. Plant Soil. 2000;224:259–72. doi:10.1023/A:1004835621371. [Google Scholar] [CrossRef]
29. El-Mahrouk ESM, Atef EAM, Gabr MK, Aly MA, Głowacka A, Ahmed MA. Application of ZnO NPs, SiO2 NPs and date pollen extract as partial substitutes to nitrogen, phosphorus, and potassium fertilizers for sweet basil production. Plants. 2024;13(2):172. doi:10.3390/plants13020172 [Google Scholar] [PubMed] [CrossRef]
30. Ahmad W, Coffman L, Weerasooriya AD, Crawford K, Khan AL. The silicon regulates microbiome diversity and plant defenses during cold stress in Glycine max L. Front Plant Sci. 2024;14:1280251. doi:10.3389/fpls.2023.1280251 [Google Scholar] [PubMed] [CrossRef]
31. Khoshgoftarmanesh AH, Khodarahmi S, Haghighi M. Effect of silicon nutrition on lipid peroxidation and antioxidant response of cucumber plants exposed to salinity stress. Arch Agron Soil Sci. 2014;60:639–53. doi:10.1080/03650340.2013.822487. [Google Scholar] [CrossRef]
32. Mukarram M, Khan MMA, Corpas FJ. Silicon nanoparticles elicit an increase in lemongrass (Cymbopogon flexuosus (Steud.) Wats) agronomic parameters with a higher essential oil yield. J Hazard Mater. 2021;412:125254. doi:10.1016/j.jhazmat.2021.125254 [Google Scholar] [PubMed] [CrossRef]
33. Rady MM, Elrys AS, El-Maati MFA, Desoky ESM. Interplaying roles of silicon and proline effectively improve salt and cadmium stress tolerance in Phaseolus vulgaris plant. Plant Physiol Biochem. 2019;139:558–68. doi:10.1016/j.plaphy.2019.04.025 [Google Scholar] [PubMed] [CrossRef]
34. Khaki S, Wang L. Crop yield prediction using deep neural networks. Front Plant Sci. 2019;10:621. doi:10.3389/fpls.2019.00621 [Google Scholar] [PubMed] [CrossRef]
35. Laane HM. The effects of foliar sprays with different silicon compounds. Plants. 2018;7(2):45. doi:10.3390/plants7020045 [Google Scholar] [PubMed] [CrossRef]
36. Hussain S, Mumtaz M, Manzoor S, Li SX, Ahmed I, Skalicky M, et al. Foliar application of silicon improves growth of soybean by enhancing carbon metabolism under shading conditions. Plant Physiol Biochem. 2021;159:43–52. doi:10.1016/j.plaphy.2020.11.053 [Google Scholar] [PubMed] [CrossRef]
37. Rashad YM, El-Sharkawy HH, Belal BE, Abdel Razik ES, Galilah DA. Silica nanoparticles as a probable anti-oomycete compound against downy mildew, and yield and quality enhancer in grapevines: field evaluation, molecular, physiological, ultrastructural, and toxicity investigations. Front Plant Sci. 2021;12:763365. doi:10.3389/fpls.2021.763365 [Google Scholar] [PubMed] [CrossRef]
38. Younes NA, El-Sherbiny M, Alkharpotly AA, Sayed OA, Dawood AF, Hossain MA, et al. Rice-husks synthesized-silica nanoparticles modulate silicon content, ionic homeostasis, and antioxidants defense under limited irrigation regime in eggplants. Plant Stress. 2024;11:100330. doi:10.1016/j.stress.2023.100330. [Google Scholar] [CrossRef]
39. Sattar A, Sher A, Ijaz M, Ul-Allah S, Hussain S, Rasheed U, et al. Modulation of antioxidant defense mechanisms and morpho-physiological attributes of wheat through exogenous application of silicon and melatonin under water deficit conditions. Sustainability. 2023;15(9):7426. doi:10.3390/su15097426. [Google Scholar] [CrossRef]
40. Al-Selwey WA, Alsadon AA, Ibrahim AA, Labis JP, Seleiman MF. Effects of zinc oxide and silicon dioxide nanoparticles on physiological, yield, and water use efficiency traits of potato grown under water deficit. Plants. 2023;12(1):218. doi:10.3390/plants12010218 [Google Scholar] [PubMed] [CrossRef]
41. Liu B, Soundararajan P, Manivannan A. Mechanisms of silicon-mediated amelioration of salt stress in plants. Plants. 2019;8(9):307. doi:10.3390/plants8090307 [Google Scholar] [PubMed] [CrossRef]
42. Mahmoud AWM, Samy MM, Sany H, Eid RR, Rashad HM, Abdeldaym EA. Nanopotassium, nanosilicon, and biochar applications improve potato salt tolerance by modulating photosynthesis, water status, and biochemical constituents. Sustainability. 2022;14(2):723. doi:10.3390/su14020723. [Google Scholar] [CrossRef]
43. Raza MAS, Zulfiqar B, Iqbal R, Muzamil MN, Aslam MU, Muhammad F, et al. Morpho-physiological and biochemical response of wheat to various treatments of silicon nano-particles under drought stress conditions. Sci Rep. 2023;13:2700. doi:10.1038/s41598-023-29784-6 [Google Scholar] [PubMed] [CrossRef]
44. Detmann KC, Araújo WL, Martins SC, Sanglard LM, Reis JV, Detmann E, et al. Silicon nutrition increases grain yield, which, in turn, exerts a feed-forward stimulation of photosynthetic rates via enhanced mesophyll conductance and alters primary metabolism in rice. New Phytol. 2012;196(3):752–62. doi:10.1111/j.1469-8137.2012.04299.x [Google Scholar] [PubMed] [CrossRef]
45. Xie Z, Song F, Xu H, Shao H, Song R. Effects of silicon on photosynthetic characteristics of maize (Zea mays L.) on alluvial soil. Sci World J. 2014;2014:1–6. doi:10.1155/2014/718716 [Google Scholar] [PubMed] [CrossRef]
46. Zhang W, Xie Z, Lang D, Cui J, Zhang X. Beneficial effects of silicon on abiotic stress tolerance in legumes. J Plant Nutr. 2017;40(15):2224–36. doi:10.1080/01904167.2017.1346127. [Google Scholar] [CrossRef]
47. Faralli M, Matthews J, Lawson T. Exploiting natural variation and genetic manipulation of stomatal conductance for crop improvement. Curr Opin Plant Biol. 2019;49:1–7. doi:10.1016/j.pbi.2019.01.003 [Google Scholar] [PubMed] [CrossRef]
48. Yamori W, Kusumi K, Iba K, Terashima I. Increased stomatal conductance induces rapid changes to photosynthetic rate in response to naturally fluctuating light conditions in rice. Plant Cell Environ. 2020;43(5):1230–40 [Google Scholar] [PubMed]
49. Mukarram M, Khan MMA, Kurjak D, Lux A, Corpas FJ. Silicon nanoparticles (SiNPs) restore photosynthesis and essential oil content by upgrading enzymatic antioxidant metabolism in lemongrass (Cymbopogon flexuosus) under salt stress. Front Plant Sci. 2023;14:733. doi:10.3389/fpls.2023.1116769 [Google Scholar] [PubMed] [CrossRef]
50. Zhu Y, Gong H. Beneficial effects of silicon on salt and drought tolerance in plants. Agron Sustain Dev. 2014;34:455–72. [Google Scholar]
51. Vaculík M, Pavlovič A, Lux A. Silicon alleviates cadmium toxicity by enhanced photosynthetic rate and modified bundle sheath’s cell chloroplasts ultrastructure in maize. Ecotoxicol Environ Saf. 2015;120:66–73. doi:10.1016/j.ecoenv.2015.05.026 [Google Scholar] [PubMed] [CrossRef]
52. Hasanuzzaman M, Bhuyan M, Nahar K, Hossain M, Mahmud J, Hossen M, et al. Potassium: a vital regulator of plant responses and tolerance to abiotic stresses. Agronomy. 2018;8(3):31. doi:10.3390/agronomy8030031. [Google Scholar] [CrossRef]
53. Kirschbaum MUF. McMillan AMS. Warming and elevated CO2 have opposing influences on transpiration. Which is more important? Curr For Rep. 2018;4:51–71. doi:10.1007/s40725-018-0073-8. [Google Scholar] [CrossRef]
54. Hidalgo-Santiago L, Navarro-León E, López-Moreno FJ, Arjó G, González LM, Ruiz JM, et al. The application of the silicon-based biostimulant Codasil® offset water deficit of lettuce plants. Sci Hortic. 2021;285:110177. doi:10.1016/j.scienta.2021.110177. [Google Scholar] [CrossRef]
55. Calles T. Preface to special issue on leguminous pulses. Plant Cell Tissue Organ Cult. 2016;127:541–2. doi:10.1007/s11240-016-1146-7. [Google Scholar] [CrossRef]
56. Abdelkader M, Voronina L, Baratova L, Shelepova O, Zargar M, Puchkov M, et al. Biostimulants-based amino acids augment physio-biochemical responses and promote salinity tolerance of lettuce plants (Lactuca sativa L.). Horticulturae. 2023;9(7):807. [Google Scholar]
57. Repke RA, Silva DMR, dos Santos JCC, de Almeida Silva M. Alleviation of drought stress in soybean by applying a biostimulant based on amino acids and macro-and micronutrients. Agronomy. 2022;12(10):2244. doi:10.3390/agronomy12102244. [Google Scholar] [CrossRef]
58. Liu P, Yin L, Deng X, Wang S, Tanaka K, Zhang S. Aquaporin-mediated increase in root hydraulic conductance is involved in silicon-induced improved root water uptake under osmotic stress in Sorghum bicolor L. J Exp Bot. 2014;65(17):4747–56 [Google Scholar] [PubMed]
59. Guidi L, Lo Piccolo E, Landi M. Chlorophyll fluorescence, photoinhibition and abiotic stress: does it make any difference the fact to be a C3 or C4 species? Front Plant Sci. 2019;10:174. doi:10.3389/fpls.2019.00174 [Google Scholar] [PubMed] [CrossRef]
60. Oszako T, Kowalczyk K, Zalewska W, Kukina O, Nowakowska JA, Rutkiewicz A, et al. Feasibility of using a silicon preparation to promote growth of forest seedlings: application to pine (Pinus sylvestris) and Oak (Quercus robur). Forests. 2023;14(3):577. doi:10.3390/f14030577. [Google Scholar] [CrossRef]
61. Ahmad B, Khan MMA, Jaleel H, Shabbir A, Sadiq Y, Uddin M. Silicon nanoparticles mediated increase in glandular trichomes and regulation of photosynthetic and quality attributes in Mentha piperita L. J Plant Growth Regul. 2020;39:346–57. doi:10.1007/s00344-019-09986-x. [Google Scholar] [CrossRef]
62. Hafez EM, Osman HS, Gowayed SM, Okasha SA, Omara AED, Sami R, et al. Minimizing the adversely impacts of water deficit and soil salinity on maize growth and productivity in response to the application of plant growth-promoting rhizobacteria and silica nanoparticles. Agronomy. 2021;11:676. doi:10.3390/agronomy11040676. [Google Scholar] [CrossRef]
63. Teixeira GCM, de Mello Prado R, Oliveira KS, D’Amico-Damião V, da Silveira Sousa Junior G. Silicon increases leaf chlorophyll content and iron nutritional efficiency and reduces iron deficiency in sorghum plants. J Soil Sci Plant Nut. 2020;20:1311–20. doi:10.1007/s42729-020-00214-0. [Google Scholar] [CrossRef]
64. Marschener P. Marschner’s mineral nutrition of higher plants. San Diego, California, USA: Academic Press; 2012. doi:10.1017/S001447971100130X. [Google Scholar] [CrossRef]
65. Rahman MA, Song Y, Hasan MM, Jahan MS, Siddiqui MH, Park HS, et al. Mechanistic basis of silicon mediated cold stress tolerance in Alfalfa (Medicago sativa L.). Silicon. 2023;16:1–13. doi:10.1007/s12633-023-02697-9. [Google Scholar] [CrossRef]
66. Nikolic DB, Nesic S, Bosnic D, Kostic L, Nikolic M, Samardzic JT. Silicon alleviates iron deficiency in barley by enhancing expression of strategy II genes and metal redistribution. Front Plant Sci. 2019;10:416. doi:10.3389/fpls.2019.00416 [Google Scholar] [PubMed] [CrossRef]
67. Sharf-Eldin AA, Alwutayd KM, El-Yazied AA, El-Beltagi HS, Alharbi BM, Eisa MA, et al. Response of maize seedlings to silicon dioxide nanoparticles (SiO2NPs) under drought stress. Plants. 2023;12(14):2592. doi:10.3390/plants12142592 [Google Scholar] [PubMed] [CrossRef]
68. Sutulienė R, Ragelienė L, Samuolienė G, Brazaitytė A, Urbutis M, Miliauskienė J. The response of antioxidant system of drought-stressed green pea (Pisum sativum L.) affected by watering and foliar spray with silica nanoparticles. Horticulturae. 2021;8(1):35. doi:10.3390/horticulturae8010035. [Google Scholar] [CrossRef]
69. Elshayb OM, Nada AM, Sadek AH, Ismail SH, Shami A, Alharbi BM, et al. The integrative effects of biochar and ZnO nanoparticles for enhancing rice productivity and water use efficiency under irrigation deficit conditions. Plants. 2022;11(11):1416. doi:10.3390/plants11111416 [Google Scholar] [PubMed] [CrossRef]
70. Xiong B, Li L, Li Q, Mao H, Wang L, Bie Y, et al. Identification of photosynthesis characteristics and Chlorophyll metabolism in leaves of Citrus Cultivar (Harumi) with varying degrees of Chlorosis. Int J Mol Sci. 2023;24(9):8394. doi:10.3390/ijms24098394 [Google Scholar] [PubMed] [CrossRef]
71. Chamizo-Ampudia A, Sanz-Luque E, Llamas A, Galvan A, Fernandez E. Nitrate reductase regulates plant nitric oxide homeostasis. Trends Plant Sci. 2017;22:163–74. doi:10.1016/j.tplants.2016.12.001 [Google Scholar] [PubMed] [CrossRef]
72. Imran M, Sun X, Hussain S, Ali U, Rana MS, Rasul F, et al. Molybdenum-induced effects on nitrogen metabolism enzymes and elemental profile of winter wheat (Triticum aestivum L.) under different nitrogen sources. Int J Mol Sci. 2019;20(12):3009. doi:10.3390/ijms20123009 [Google Scholar] [PubMed] [CrossRef]
73. Signore A, Bell L, Santamaria P, Wagstaff C, van Labeke MC. Red light is effective in reducing nitrate concentration in rocket by increasing nitrate reductase activity and contributes to increased total glucosinolates content. Front Plant Sci. 2020;11:604. doi:10.3389/fpls.2020.00604 [Google Scholar] [PubMed] [CrossRef]
74. da Silva ES, de Mello Prado R, Soares ADAVL, de Almeida HJ, dos Santos DMM. Response of corn seedlings (Zea mays L.) to different concentrations of nitrogen in absence and presence of silicon. Silicon. 2021;13:813–8. doi:10.1007/s12633-020-00480-8. [Google Scholar] [CrossRef]
75. Gou T, Yang L, Hu W, Chen X, Zhu Y, Guo J, et al. Silicon improves the growth of cucumber under excess nitrate stress by enhancing nitrogen assimilation and chlorophyll synthesis. Plant Physiol Biochem. 2020;152:53–61. doi:10.1016/j.plaphy.2020.04.031 [Google Scholar] [PubMed] [CrossRef]
76. Liu XQ, Lee KS. Effect of mixed amino acids on crop growth. Agri Sci. 2012;1:119–58. [Google Scholar]
77. Chen BM, Wang ZH, Li SX, Wang GX, Song HX, Wang XN. Effects of nitrate supply on plant growth, nitrate accumulation, metabolic nitrate concentration and nitrate reductase activity in three leafy vegetables. Plant Sci. 2004;167:635–43. doi:10.1016/j.plantsci.2004.05.015. [Google Scholar] [CrossRef]
78. Hao Y, Yu Y, Sun G, Gong X, Jiang Y, Lv G, et al. Effects of multi-walled carbon nanotubes and nano-silica on root development, leaf photosynthesis, active oxygen and nitrogen metabolism in maize. Plants. 2023;12(8):1604. doi:10.3390/plants12081604 [Google Scholar] [PubMed] [CrossRef]
79. Rouphael Y, Colla G. Synergistic biostimulatory action: designing the next generation of plant biostimulants for sustainable agriculture. Front Plant Sci. 2018;9:1655 [Google Scholar] [PubMed]
80. Teixeira WF, Fagan EB, Soares LH, Umburanas RC, Reichardt K, Neto DD. Foliar and seed application of amino acids affects the antioxidant metabolism of the soybean crop. Front Plant Sci. 2017;8:327 [Google Scholar] [PubMed]
81. Ahmad Z, Waraich EA, Barutçular C, Hossain A, Erman M, Çiğ F, et al. Enhancing drought tolerance in wheat through improving morpho-physiological and antioxidants activities of plants by the supplementation of foliar silicon. Phyton-Int J Exp Bot. 2020;89(3):529–39. doi:10.32604/phyton.2020.09143. [Google Scholar] [CrossRef]
82. Trejo-Téllez LI, García-Jiménez A, Escobar-Sepúlveda HF, Ramírez-Olvera SM, Bello-Bello JJ. Silicon induces hormetic dose-response effects on growth and concentrations of chlorophylls, amino acids and sugars in pepper plants during the early developmental stage. PeerJ. 2020;8:e9224. doi:10.7717/peerj.9224 [Google Scholar] [PubMed] [CrossRef]
83. Halpern M, Bar-Tal A, Ofek M, Minz D, Muller T, Yermiyahu U. The use of biostimulants for enhancing nutrient uptake. Adv Agron. 2015;130:141–74. doi:10.1016/bs.agron.2014.10.001. [Google Scholar] [CrossRef]
84. Khan S, Yu H, Li Q, Gao Y, Sallam BN, Wang H, et al. Exogenous application of amino acids improves the growth and yield of lettuce by enhancing photosynthetic assimilation and nutrient availability. Agronomy. 2019;9(5):266. doi:10.3390/agronomy9050266. [Google Scholar] [CrossRef]
85. Yakhin OI, Lubyanov AA, Yakhin IA, Brown PH. Biostimulants in plant science: a global perspective. Front Plant Sci. 2017;7:2049. doi:10.3389/fpls.2016.02049 [Google Scholar] [PubMed] [CrossRef]
86. Woltz SS, Jackson CR. Production of yellow strapleaf of chrysanthemum & similar disorders by amino acid treatment. Plant Physiol. 1961;36(2):197. doi:10.1104/pp.36.2.197 [Google Scholar] [PubMed] [CrossRef]
87. Schillberg S, Raven N, Spiegel H, Rasche S, Buntru M. Critical analysis of the commercial potential of plants for the production of recombinant proteins. Front Plant Sci. 2019;10:720. doi:10.3389/fpls.2019.00720 [Google Scholar] [PubMed] [CrossRef]
88. Cuong TX, Ullah H, Datta A, Hanh TC. Effects of silicon-based fertilizer on growth, yield and nutrient uptake of rice in tropical zone of Vietnam. Rice Sci. 2017;24(5):283–90. doi:10.1016/j.rsci.2017.06.002. [Google Scholar] [CrossRef]
89. Helal NM, Khattab HI, Emam MM, Niedbała G, Wojciechowski T, Hammami I, et al. Improving yield components and desirable eating quality of two wheat genotypes using Si and NanoSi particles under heat stress. Plants. 2022;11(14):1819. doi:10.3390/plants11141819 [Google Scholar] [PubMed] [CrossRef]
90. Soundararajan P, Sivanesan I, Jana S, Jeong BR. Influence of silicon supplementation on the growth and tolerance to high temperature in Salvia splendens. Hortic Environ Biotech. 2014;55:271–9. doi:10.1007/s13580-014-0023-8. [Google Scholar] [CrossRef]
Cite This Article
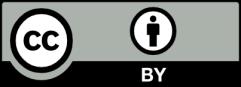
This work is licensed under a Creative Commons Attribution 4.0 International License , which permits unrestricted use, distribution, and reproduction in any medium, provided the original work is properly cited.