Open Access
REVIEW
Plant Chemical Defenses against Insect Herbivores—Using the Wild Tobacco as a Model
1 Hubei Provincial Tobacco Research Institute, Wuhan, 430030, China
2 Shiyan Branch of Hubei Tobacco Company, Shiyan, 442000, China
3 State Key Laboratory of Biocatalysis and Enzyme Engineering, School of Life Sciences, Hubei University, Wuhan, 430062, China
* Corresponding Authors: Zhenguo Chen. Email: ; Shiyou Lü. Email:
(This article belongs to the Special Issue: Plant Secondary Metabolism and Functional Biology)
Phyton-International Journal of Experimental Botany 2024, 93(4), 641-659. https://doi.org/10.32604/phyton.2024.049285
Received 02 January 2024; Accepted 15 March 2024; Issue published 29 April 2024
Abstract
The Nicotiana genus, commonly known as tobacco, holds significant importance as a crucial economic crop. Confronted with an abundance of herbivorous insects that pose a substantial threat to yield, tobacco has developed a diverse and sophisticated array of mechanisms, establishing itself as a model of plant ecological defense. This review provides a concise overview of the current understanding of tobacco’s defense strategies against herbivores. Direct defenses, exemplified by its well-known tactic of secreting the alkaloid nicotine, serve as a potent toxin against a broad spectrum of herbivorous pests. Moreover, in response to herbivore attacks, tobacco enhances the discharge of volatile compounds, harnessing an indirect strategy that attracts the predators of the herbivores. The delicate balance between defense and growth leads to the initiation of most defense strategies only after a herbivore attack. Among plant hormones, notably jasmonic acid (JA), play central roles in coordinating these defense processes. JA signaling interacts with other plant hormone signaling pathways to facilitate the extensive transcriptional and metabolic adjustments in plants following herbivore assault. By shedding light on these ecological defense strategies, this review emphasizes not only tobacco’s remarkable adaptability in its natural habitat but also offers insights beneficial for enhancing the resilience of current crops.Keywords
Plants have inhabited terrestrial landscapes for approximately 400 million years and in this extensive timeline have intricately co-evolved with insects [1]. Nearly half of all insect species are herbivorous, which can be further categorized based on their feeding modalities as chewers or suckers, posing threats to the survival of plants (Fig. 1). Tobacco, as a significant economic crop, has emerged as a crucial model organism in biological research due to its simple genome, extensive genetic variation, short growth cycle, and ease of cultivation. In natural environments, tobacco frequently faces attacks from chewing specialist herbivores like tobacco hornworm (Manduca sexta) larvae [2,3] as well as assucking pests such as aphids and whiteflies (Bemisia tabaci) [4]. These factors contribute to its suitability as an excellent model for studying plant-herbivore interactions.
Figure 1: A schematic overview of tobacco plant responses to herbivory. Tobacco faces attacks from both chewer herbivores like tobacco hornworms and sucker herbivores such as tobacco whiteflies, aphids, and tobacco leafhoppers. In the process of tobacco responding to herbivory, the hormone crosstalk network centered around JA plays a crucial role. Hormonal responses ultimately lead to the production of four major classes of defensive secondary metabolites, including alkaloids, phenylpropanoid compounds, green leaf volatiles, and terpenoids
In response to the diverse threats posed by herbivores, tobacco plants have evolved a finely-tuned defense mechanism throughout their evolutionary history. Secondary metabolites such as alkaloids, phenylpropanoid, and herbivory-induced plant volatiles (HIPVs), play a central role in this defense strategy (Fig. 1). Alkaloids, especially nicotine, deter a broad spectrum of generalist herbivores and also adversely affects the growth and behavior of specialist herbivores like tobacco hornworms [5,6]. Phenylpropanoid compounds in tobacco have been identified as vital components of the natural defense system against herbivores [7–9]. HIPVs with their multifaceted role in defense, act as both direct defense compounds to deter herbivores’ attacks [10], and indirect defense compounds, attracting herbivores’ predators [11,12] or eliciting non-specific defensive responses in neighboring plants [13]. The optimal defense theory states that plants allocate their defense resources based on the costs of defense, the importance of different plant tissues, and the probability of those tissues being attacked by herbivores. According to this theory, plants prioritize the protection of critical and vulnerable organs essential for their survival and reproduction [14]. However, the continuous synthesis of these chemical defenses comes with a significant metabolic cost. Therefore, when faced with most herbivorous insect threats, tobacco plants only activate these chemical defenses post-attack [15]. This process is modulated by a series of hormonal signaling pathways, with the jasmonic acid (JA) signaling pathway playing a pivotal role [16] (Fig. 1). The JA signaling pathway collaborates closely with other hormonal signaling pathways, such as those of salicylic acid (SA), abscisic acid (ABA), brassinolide (BR) and cytokinin (CK), to collectively regulate induced defenses following herbivory [17–20] (Fig. 1). This multi-tiered and multi-strategical integrated defense exemplifies how tobacco navigates the intricate hormonal regulation, trade-offs, and adaptations in its prolonged interactions with the environment and herbivores, a topic that has garnered significant attention in contemporary evolutionary biology and ecology.
Given the paramount importance of this topic, in-depth research into tobacco defense strategies is crucial for enhancing agricultural yields. Over the past few decades, substantial advancement has been made in deciphering the defense tactics employed by tobacco against insects. In this context, we review the range of defense strategies deployed by tobacco upon perceiving herbivore threats and how plant hormonal signaling dynamics shape these responses. We also summarized the key genes guiding the defense of tobacco against herbivores and offered insights into leveraging these genetic resources for the improvement of tobacco and other crop species.
2 Defensive Secondary Metabolites
In nature, the co-evolutionary interactions between plants and their antagonists are believed to drive the remarkable diversity of plant secondary metabolites (PSMs) [21]. PSM ensures the resilience and persistence of plants when exposed to challenging environmental conditions. Decades of research have highlighted the pivotal role of a substantial number of PSMs in plant defense response to herbivores [22]. In Nicotiana plants, alkaloids, phenylpropanoid compounds, and green leaf volatiles, terpenoids. play a major role in defense against herbivores. Depending on their volatility, these metabolites are generally classified into two main categories: non-volatile and volatile defensive PSMs. Based on the defense type, these metabolites are also typically categorized into two major classes: direct defense and indirect defensive PSMs. Direct defense metabolites can impact the growth and preference of herbivores, and the fitness-limiting resources investment required for their production might be greater than that needed for the generation of volatile compounds [23]. Indirect defenses have the potential to eliminate or incapacitate herbivores in the early stages of an attack, offering protection that might surpass that provided by the deployment of direct defenses. Typically, direct defenses do not result in the departure of adapted herbivores from the environment [12,24].
Species of the genus Nicotiana have been employed as model systems to explore the role of alkaloids in plant-herbivore interactions [25]. Alkaloids constitute a structurally diverse set of nitrogenous natural compounds, with their nitrogen atoms derived from amino acids [26]. Principal alkaloids identified in the genus Nicotiana include nicotine, nornicotine, anabasine, and anatabine [27]. Nicotine, consisting of over 90% of the alkaloid content in most tobacco species stands as the predominant alkaloid content in most tobacco species and has been extensively studied for its defensive role against herbivores [28]. Recent findings indicate that tobacco plants can even sense isolated vibrations produced by herbivores, thereby activating nicotine induction [29]. The structural resemblance between nicotine and acetylcholine facilitates the activation of cholinergic receptors by nicotine under natural stimulation. Nicotine, as a potent cholinergic agonist, binds with the nicotinic acetylcholine receptors (nAChRs), the most abundant excitatory postsynaptic receptors in insects [30]. This binding leads to an overstimulation of the insect’s nervous system, often culminating in paralysis and eventual death. Field experiments with tobacco plants silenced for putrescine N-methyltransferase (PMT) convincingly demonstrated the protective role of nicotine in nature. The silenced plants exhibited a threefold increase in leaf damage inflicted by herbivores [24]. However, in the coevolutionary arms race between tobacco plants and herbivores, specialist herbivores are not to be outdone. Herbivores can modify chemical defenses and even prevent their induction after harm. The tobacco specialist tobacco hornworm exemplifies this. They can disrupt the biosynthesis of nicotine in the roots through their oral regurgitant [23]. Although they grow more slowly on high-nicotine diets, they can withstand doses that are fatal to other herbivores [31]. Further studies indicate that M. sexta might use dietary nicotine as a defensive measure against its parasitoid, Cotesia congregata, which experiences higher mortality rates when parasitizing larvae that consume a high-nicotine diet [32]. This ongoing dynamic suggests a potential erosion in the defensive potency of nicotine over time.
The phenylpropanoid pathway is a crucial biochemical mechanism in plants, synthesizing a myriad of bioactive metabolites essential for plant function and resilience, including many compounds with anti-herbivore properties [33]. The enzyme phenylalanine ammonia-lyase (PAL) at the core of this intricate network catalyzes the conversion of L-phenylalanine to cinnamic acid, paving the way for the foundation for the production of various compounds [34]. The substrate, upon hydroxylation, forms p-hydroxycinnamic acid [33]. Through a conjugation process, it further branches out to produce compounds such as chlorogenic acid (CGA). The aggregation of its derived lignin monomers leads to the formation of lignin. Furthermore, through a series of biochemical reactions, the synthesis of phenolic amides is facilitated, including compounds such as caffeoyl putrescine (CP) and dicaffeoylspermidine (DCS) [33]. Many excellent reviews have detailed the biosynthesis of these phenylpropanoid compounds [35,36]. In this overview, we primarily elucidate the physiological roles of these phenylpropanoid metabolites in tobacco plants in defense against herbivores.
CGA is ubiquitously distributed throughout the plant kingdom and acts as a predominant phenylpropanoid, playing a pivotal role in plant defense mechanisms [37]. In tobacco plants, the hydroxycinnamoyl CoA quinate hydroxycinnamoyl transferase enzyme (HQT) represents the critical catalyst in the final step of the biosynthetic pathway, converting caffeoyl-CoA into CGA [37]. After being attacked by the stem borer T. mucorea, tobacco pith CGA levels increased sharply [7]. Moreover, experiments conducted in both greenhouse and field conditions with plants silenced in CGA biosynthesis (irHQT plants) demonstrated that CGA, along with other caffeic acid conjugates, functions as a direct defense mechanism, protecting the pith against T. mucorea attack [7]. However, a recent intriguing study revealed an antagonistic interaction between CGA and pyrrolizidine alkaloid (PA) in defending against thrips. In contrast, a synergistic interaction was observed between PA N-oxides and CGA. Intriguingly, the PA resulted in a higher mortality rate in thrips compared to PA N-oxides [38]. This paradoxical phenomenon suggests that CGA may interact with diverse plant metabolites, contributing to the intricate chemical milieu underlying plant defense against herbivores.
Lignin, a pivotal structural heteropolymer in plants, is synthesized through pathways involving enzymes such as cinnamoyl-CoA reductases (CCRs) and cinnamyl alcohol dehydrogenases (CADs) [39]. While its role in bolstering mechanical rigidity and offering protection against pathogens and herbivores is recognized, molecular evidence substantiating its defensive role against herbivory, especially based on lignin pathway silencing, remains scant. In field studies, these NaCAD-silenced specimens exhibited pronounced susceptibility to stem-boring weevils, specifically T. mucorea [40]. Follow-up studies have shown that unlignified pith is highly lignified against herbivore attack and that it inhibits the growth of pith-feeding herbivores [8]. Furthermore, interestingly, a large amount of feruloyltyramine was found in the attacked pulp as a newly emerging lignin component, which may be the result of alternative lignin metabolism caused by herbivorous attacks [8]. In summation, while lignin is postulated to play a critical role in plant chemical defenses, its precise function and interplay with other metabolic constituents present a multifaceted paradigm warranting further exploration.
Phenolamides, derived from hydroxycinnamic acid derivatives combined with aliphatic or aromatic amines, play a pivotal role in plant defense against herbivores [41]. The primary phenolamides in tobacco comprise CP and DCS [9]. The optimal defense theory posits that plants strategically allocate more defense-associated metabolites towards their valuable parts, such as young leaves, to protect from herbivore predation [42]. Concordantly, N. attenuata plants exhibit a shift in CP accumulation during their growth and in response to herbivore-associated cues [9]. Studies have indicated that a lack of CP and DCS accumulation renders plants more susceptible to herbivore predation [9]. Although the application of CP to N. attenuata leaves hampers the growth of M. sexta larvae [9], the exact mode of phenolamide toxicity in herbivores remains elusive, particularly in natural contexts. While research primarily focused on CP and DCS, a study has shown that a broader spectrum of PAs can be upregulated in N. attenuata leaves through simulated herbivory [43]. This array of phenolamides appears to accumulate in a NaMYB8-dependent manner [43], suggesting a concerted regulatory mechanism. The diverse range of phenolamides regulated during herbivory implies that a blend of these compounds might be essential for maximizing defense efficiency against herbivores.
2.3 Green Leaf Volatiles (GLVs)
GLVs primarily comprise C6 aldehydes, alcohols, and esters, derived from fatty acids via the Lipoxygenase (LOX)/Hydroperoxide Lyase (HPL) pathway [44]. Upon mechanical damage or caterpillar oral secretions (OS), GLVs are swiftly released, providing rapid and reliable information on the exact location of the attacking herbivore. Y-tube choice assays and field experiments in N. attenuate plants demonstrated that reduced expression of HPL (as-hpl) in N. attenuata plants demonstrated diminished attraction to the generalist predator Geocoris ssp, which feeds on eggs and early larvae of the specialist lepidopteran herbivore M. sexta [45]. Another study indicated that the (Z)/(E)-ratio of GLVs released by N. attenuata plants upon caterpillar attack by M. sexta changes, and this herbivore-induced shift in the (Z)/(E) ratio triples the foraging efficiency of the generalist predator Geocoris spp. in nature settings [46]. Beyond attracting predators or parasitoids of herbivores, GLVs have also been reported to directly deter herbivores by inhibiting oviposition [47]. While indirect defense mediated by the release of plant volatiles is well-described, evidence linking increased attraction of predators and parasitoids to enhance plant fitness remains limited. However, Schuman et al. demonstrated that wild-type N. attenuata plants, producing higher GLV levels, exhibit twice as many buds and flowers as plants with reduced GLV emission (hemi-irLOX2) [11]. Recent research has also unveiled an unknown metabolite named caffeoylputrescine-green leaf volatile compound (CPH), generated by the conjunction of coumaroylagmatine and GLVs, specifically C-6 aldehydes [10]. Abundantly produced when tobacco plants are infested by E. decipiens leafhoppers, CPH effectively defends against leafhopper attacks [10] This discovery underscores the potential modulation of plant internal metabolites to enhance resistance against herbivores, offering a novel perspective in plant defense strategies.
Terpenoids, a diverse group of organic compounds with over 80,000 identified variants, play a crucial role in plant defense [48]. In tobacco plants, terpenoids exhibit remarkable efficacy in deterring herbivores, operating through both volatile and non-volatile mechanisms. Volatile terpenoids, particularly monoterpenes and sesquiterpenes, act as an indirect line of defense [49]. These compounds, often released alongside GLVs, are characterized by their evaporation properties. Notably, monoterpene thymol exhibits pronounced toxic, growth-inhibitory, and repellant effects against the tobacco cutworm (Spodoptera litura Fab.) upon direct application [50]. Additionally, when N. attenuata foliage is consumed by specific herbivores like M. sexta larvae, there is a notable increase in the emission of volatile monoterpenes and sesquiterpenoids, including trans-α-bergamotene [51]. This response is further amplified when plant leaves are mechanically damaged and interact with larval secretions, leading to an enhanced release of these volatile compounds [52]. For instance, the herbivory of N. attenuata by various herbivores such as M. quinquemaculata, T. notatus, and E. hirtipennis, results in the induction of sesquiterpenes like trans-α-bergamotene and trans-β-farnesene [12]. These elevated VOC levels, particularly of trans-α-bergamotene, attract predators like big-eyed bugs (Geocoris pallens), which effectively control herbivore populations, particularly those of Manduca spp. eggs and small larvae on plants [12]. Additionally, the function of sesquiterpenes also exhibits tissue specificity. For instance, the induction of (E)-α-bergamotene in flowers enhances M. sexta moth-mediated pollination success, while the same compound in leaves mediates indirect defense against M. sexta larvae [12,46,49].
In contrast, non-volatile terpenoids offer a different mode of protection. Analysis of leaf surface exudates from N. tabacum cultivars resistant to the tobacco budworm, H. virescens, revealed the presence of unique compounds such as α- and β-duvatriendiol, α- and β-duvatriene-ols, cis-abienol, and 15-hydroxy abienol [53]. These cultivars demonstrated higher mortality rates among budworm larvae, correlating with higher levels of cembranoid diteropenoids or sucrose esters (SCEs) [53]. Furthermore, in N. attenuata plants, Hydroxygeranyllinalool diterpene glycosides (HGL-DTGs) have emerged as a potent defense against the tobacco hornworm, M. sexta. Their role was first highlighted through artificial diet-bioassay purification and further confirmed by creating HGL-DTG-deficient lines using virus-induced gene silencing (VIGS) [54–56]. Hornworm larvae, when fed on these HGL-DTG-deficient plants, gained thrice the mass compared to those on control plants or plants silenced in the TPI-associated gene [54]. This significant difference underscores the paramount defensive role of HGL-DTGs in tobacco plants, even outperforming known defenses like TPIs against certain hornworm larvae. Overall, the multifaceted role of terpenes in tobacco plant defense underscores their importance. Both volatile and non-volatile terpenoids contribute significantly to the ability of tobacco plants to deter herbivores, offering a nuanced and effective defense strategy.
3 Herbivory-Induced Jasmonic Acid Signaling Pathway in Tobacco
Plants, as sessile organisms, must continually adjust their defensive responses to cope with attacks by various herbivores, achieving higher adaptability and survival rates in natural environments where trade-offs between defense and growth often determine plant performance [57]. Tobacco plants have been shown to differentiate between generalist and specialist herbivores by perceiving specific elicitors associated with particular herbivore species [19,58]. As shown in Fig. 2, fatty acid-amino acid conjugates (FACs), found in the OS of most lepidopteran larvae, are introduced into wounds during feeding and rapidly activate SIPK and WIPK, leading to the biosynthesis of JA [52]. The pathway involves the conversion of α-linolenic acid, produced by GLA1 from the chloroplast membrane, to OPDA under the catalysis of LOX3, AOS, and AOC. OPDA is then transported to peroxisomes where catalyzed by OPR3 and through β-oxidation in three steps, it is converted into JA [59]. The expression of these key genes involved in JA synthesis is significantly up-regulated following herbivore attack [60], and the silencing of these genes results in a significant decrease in tobacco plants’ resistance to herbivores. For instance, ir-lox3 tobacco plants have lower nicotine content in the pith than wild-type plants and are more susceptible to stem borer attacks [40]. JA then conjugates with isoleucine to form JA-Ile, which is known to be the major endogenous bioactive jasmonate regulating downstream defense responses in plants [61,62]. Simultaneously silencing of NaJAR4 and NaJAR6, two genes encoding enzymes conjugating JA to amino acids, inhibited JA-ile but not JA accumulation, and the tobacco plants are also vulnerable to M. sexta attack, indicating that JA-ile plays a role in plant defense against herbivores [63]. Consistent with the proposed role of FACs upstream of JA biosynthesis, the FAC-elicited herbivore resistance in N. attenuata was dramatically attenuated when JA or JA-Ile biosynthesis-associated genes such as LOX3, JAR4, JAR6, and COI1 were silenced by RNA interference or an antisense approach [56,59,64].
Figure 2: A working model summarizing herbivory-induced JA signaling and defenses regulated by JA in tobacco plants. In tobacco plants under attack, the FACs in the OS of M. sexta are introduced into the leaf tissue, rapidly activating SIPK and WIPK. The increase in SIPK and WIPK activity triggers the biosynthesis of JA. JA is further converted into JA-Ile by JAR, and the binding of JA-Ile to SCFCOI1 initiates the degradation of the negative regulatory factors of the JA response, JAZ proteins, thereby activating MYC2, inducing the production of defense compounds, including PIs and HGL-DTGs. Herbivory-induced JA signaling is also conveyed to the roots, where nicotine and related alkaloids are synthesized. The xylem transport of nicotine to the aerial parts enhances the plant’s defense against herbivores. In the leaves, NtJAT1 and NtJAT2 transport nicotine into vacuoles. GLA1, glycerolipase type A1; LOX, lipoxygenase; AOC, allene-oxide cyclase; AOS, allene oxide synthase; OPDA, 12-oxo-phytodienoic acid; OPR3, OPDA reductase 3; JAR, jasmonate resistant; COI1, coronatine insensitive 1; SCF complex, SKP, Cullin and F-box; JAZ, jasmonate ZIM-domain
In the presence of JA-Ile, JASMONATE ZIM DOMAIN (JAZ) repressors physically interact with positive transcription factors of JA signaling, such as MYC2 proteins, and are recruited to the SCFCOI1 E3 ubiquitin ligase complex, ubiquitinated, and consequently degraded by the 26S proteasome. The removal of JAZ repressors releases MYC2 and other related transcription factors, thereby triggering downstream JA-dependent defense responses (Fig. 2), including the accumulation of direct defense PSMs such as nicotine, CP, DCS, and HGL-DTGs, as well as indirect defense metabolites such as GLVs, biosynthesized and released from the leaves [11,12,24,59,65].
Compared to the JA-dependent induction of other defense metabolites in the aerial parts of tobacco plants, the mechanism underlying herbivory-induced JA-dependent accumulation of nicotine is more complex (Fig. 2). Nicotine is synthesized in the roots of the tobacco plant through two pathways: the pyridine ring pathway starting from L-aspartic acid and the pyrrolidine ring pathway starting from either arginine or ornithine [66]. The pyridine ring pathway results in the formation of nicotinic acid, while the pyrrolidine ring pathway leads to the formation of N-methyl-Δ1-pyrrolinium. These two cyclic compounds then condense to form nicotine [66]. When the tobacco plant detects herbivorous attacks on its aerial tissues, the biosynthesis and accumulation of nicotine increases not only at the site of injury but throughout the entire plant [67]. Consequently, a damage signal needs to be conveyed from the site of injury to guide the nicotine biosynthesis in the roots. However, there is a spatial separation between the nicotine biosynthesis site in the roots and the herbivore attack sites in the aerial parts. This necessitates long-distance signal transduction pathways and transport mechanisms [68]. Research indicates that the signal activating nicotine biosynthesis is transmitted to the roots via the phloem, subsequently prompting the distribution of nicotine to leaf tissues through the xylem [69,70]. Leaf injury markedly boosts the release of synthesized nicotine from the roots via the xylem [70]. In response to long-distance signaling cascades, injury augments the jasmonate pools in the stem, which, either directly via transport mechanisms or indirectly through an alternate signaling pathway, enhances the jasmonate pools in the roots. Subsequently, these enriched jasmonate pools stimulate the synthesis of nicotine in the roots and augment the nicotine reserves across the tobacco plant [71]. Studies have shown that the chemical signals resulting from injury are directly proportional to the extent of damage the plant has sustained [68–70].
4 The Hormonal Crosstalk Network Centered around Jasmonic Acid
The intricate network of hormonal signaling transduction and potentially mutually reinforcing or mutually inhibitory relationships between various hormonal pathways in the context of plant defense against herbivores play a crucial role in orchestrating a plant’s response to herbivorous attacks [72]. This sophisticated interplay enables the plant to establish an adaptive trade-off between defense and growth mechanisms, highlighting the pivotal role of hormonal communication in shaping the plant’s dynamic interaction with its environment. For instance, M. sexta larval feeding suppresses the JA-induced nicotine response in leaves, even though it also significantly amplifies JA production caused by injury. Specific suppression of the nicotine response has been attributed to the ET signal induced by M. sexta feeding in leaves [18]. This suppression may represent a shift in plant defense strategies, such as accumulating more CP and DCS, where CP has been rigorously shown to negatively affect the performance of M. sexta [18]. JA-ET crosstalk inhibits local cell expansion and growth following herbivory, thereby redirecting resources toward enhancing defenses against herbivore assault [73]. This suggests that hormonal interactions also play a significant role in the trade-off between plant growth and defense.
In addition, some key factors have been identified to modulate the crosstalk between JA signaling pathways and other hormonal signaling pathways such as BR, ABA, SA, and CK, thereby adjusting tobacco plant’s responses to herbivores (Fig. 3). In the interaction between N. attenuata and herbivores like the tobacco hornworm M. sexta, BR receptor NaBAK1 and its co-receptor NaBRI1 are critical regulators that may mediate complex interactions between BR and JA signaling pathway [74,75] (Fig. 3). Studies have shown that BAK1 is crucial for the increase in wound and herbivory-induced JA and JA-Ile levels, while BRI1 modulates JA-Ile accumulation by affecting JAR activity and Ile availability, with a lesser impact on JA accumulation [74,75]. However, NaBAK1-silenced plants show similar levels of defensive PSMs, such as TPI, and similar resistance to M. sexta larvae after simulated herbivory, indicating that BR signaling might also negatively regulate certain JA responses in N. attenuata [74]. A novel FAC-regulated protein, NaHER1 (Herbivory-Induced Regulator 1), is associated with changes in hormonal levels during plant responses to herbivory, particularly in the interplay between ABA and JA [76] (Fig. 3). Silencing NaHER1 affects JA and ABA levels and the accumulation of associated defense metabolites, leading to increased susceptibility to tobacco hornworm M. sexta [76]. Perception and signal transduction of FACs from herbivore OS induces the expression of NaHER1, which in turn increases ABA levels by inhibiting its catabolism. Elevated ABA levels enhance the accumulation of defensive metabolites and JA, bolstering N. attenuata defenses against herbivores. The antagonistic interactions between the JA and SA pathways are well documented [77], NaLecRK1 (a lectin receptor kinase) and NaNPR1 (Nonexpressor of PR-1) play a key role in regulating tobacco defenses against herbivory by mediating the crosstalk between SA and JA [78,79] (Fig. 3). In wild-type tobacco, suppression of NaLecRK1 expression leads to increased accumulation of SA, which in turn suppresses JA-mediated defenses. NaNPR1 also plays a role in regulating defenses against herbivores in tobacco plants. Studies indicate that silencing NaNPR1 leads to increased accumulation of SA and weakened JA signaling, resulting in a reduction of both direct (such as the accumulation of nicotine) and indirect (such as the release of GLVs) defense responses. Moreover, NaLecRK1 expression is activated by FAC, but JAs accumulation suppresses NaLecRK1 expression [79], suggesting a complex regulatory network to maintain defense mechanism balance. CK has been demonstrated to be a positive regulator of plant defense against pathogens [80]. Likewise, an increase in CK concentration in plants can also negatively affect the performance of herbivores [81]. Previous research reveals that after herbivore attack, the JA pathway induces the expression of NaMYB8, which is responsible for the accumulation of herbivore-induced phenolamides by regulating the expression of acyltransferases NaAT1, NaDH29, and NaCV86 [9,43]. This is consistent with results in irchk2/3 plants where the abundance of NaMYB8 transcripts and phenolamide concentrations are both reduced [82] (Fig. 3). Since the accumulation of NaMYB8 transcripts is considered to be JA-Ile-dependent [9,43], this implies that the CK signaling pathway may promote JA-Ile signaling.
Figure 3: Simplified control chart of defense activation pathway with JA signaling as the core and crosstalk with other plant hormones. BAK1, Bri1-associated receptor kinase 1; BRI1, BR insensitive 1; HER1, Herbivore elicitor-regulated 1; PYL4, Pyrabactin resistance-like 4; NPR1, nonexpressor of pathogenesis-related genes 1; LecRK1, Lectin receptor kinase 1; CHK2/3, Chase-domain containig HIS kinase 2
5 Regulatory Factors in Tobacco Plant Responses to Herbivory
In the defense arsenal of N. attenuata, the JA signaling cascade, in conjunction with its crosstalk with other hormonal pathways, forms an intricate regulatory network crucial for the plant’s resistance against herbivores [16]. This network is modulated by an array of positive regulators that amplify defense gene expression, enhancing the synthesis of protective chemical compounds. Concurrently, negative regulators fine-tune this response to prevent an excessive defensive stance that could lead to resource depletion. Such a dynamic interplay of positive and negative feedback mechanisms ensures a balance between growth and defense. In the ensuing discourse, the roles of these regulatory elements within the hormone regulatory network will be elucidated, particularly focusing on the implications of both positive and negative regulators in modulating the defensive responses of tobacco plants against herbivorous threats.
5.1 Positive Regulatory Factors
Over recent years, reverse genetic techniques including RNA interference (RNAi) and VIGS have identified numerous positive regulators of herbivory-induced JA biosynthesis, thereby enhancing the resistance of tobacco plants to herbivores. Some of these positive regulators, such as MAPKKs (NaMEK2), MAPKs (NaWIPK and NaSIPK), and WRKY transcription factors (NaWRKY3 and NaWRKY6), are upstream in JA biosynthesis (Table 1). NaSIPK and NaWIPK can be activated by upstream MAPKKs, such as NaMEK2 [83]. These MAPKs regulate the accumulation of JA and its derivatives, as well as plant defense responses, by phosphorylating specific transcription factors and other downstream effector molecules [84]. The initial evidence of MAPKs involvement in plant-herbivore interactions came from a study on N. tabacum in which Seo et al. demonstrated that NtWIPK transcripts and overall MAPK activity rapidly increased after wounding; additionally, antisense expression of NtWIPK resulted in reduced levels of JA and defense-associated genes [85]. Silencing NaWRKY3 and NaWRKY6 affects direct and indirect defenses, increasing plant sensitivity to natural herbivores. Exogenous JA application can recover the phenotype of WRKY-silenced plants, suggesting that lower JA levels in WRKY-silenced plants lead to reduced OS-induced defenses [65]. However, whether NaWRKY3 and NaWRKY6 can serve as targets for MAPKs remains unknown. SIPK and WIPK are downstream in the MAPK signaling cascade, while WRKY3 and WRKY6 are positioned even further downstream, directly affecting the accumulation of JA and the transcriptional regulation of defense genes. Beyond the upstream positive regulators of JA biosynthesis, various classes of positive regulators are also confirmed to participate in tobacco plant responses to herbivory. Three important components of the smRNAs biogenesis and action pathways in plants are the RNA-dependent RNA polymerases (RdRs), the Dicer-like proteins (DCLs), and the Argonautes (AGOs). Together they form the RNA-induced silencing complex (RISC), thereby determining the specificity of target selection based on smRNA-mRNA complementarities [86] (Table 1). When tobacco plants face herbivore attacks, specific pathways are engaged to induce herbivory-responsive mRNAs. These mRNAs regulate defense responses either by directly modulating the expression of genes related to signaling and defense or by regulating the expression of primary transcription factors [87–89]. Additionally, certain long non-coding RNAs (lncRNAs) including NaJAL1 and NaJAL3, NO level-related genes S-nitroso glutathione reductase (GSNOR), and suppressor of G-two allele of SKP1 (SGT1) have been proven to participate in part of JA-induced defensive responses [90–92] (Table 1). Interestingly, RuBPCase activase (RCA), a prevalent photosynthetic protein, when silenced, redirects JA flux from JA-Ile to methyl jasmonate (MeJA), thereby attenuating herbivory-induced defenses in N. attenuata [93] (Table 1). However, there is evidence that RCA is strongly downregulated after herbivore attack or simulated herbivory [94], implying that RCA mediates the trade-off between growth and defense.
5.2 Negative Regulatory Factors
In addition to the aforementioned positive regulators, key factors that negatively regulate the JA signaling pathway induced by herbivory have been identified in N. attenuata. These include two functionally redundant calcium-dependent protein kinases (CDPKs), namely NaCDPK4 and NaCDPK5, the NO-related proteins (NaNOA1), the JASMONATE ZIM DOMAIN proteins (NaJAZh) and the jasmonyl-L-isoleucine hydrolase 1 (NaJIH1) [95–98] (Table 1). Silencing both NaCDPK4 and NaCDPK5 (IRcdpk4/5 plants) leads to elevated levels of JA in uninduced stems and excessive JA accumulation in leaves after wounding or simulated M. sexta feeding [95] (Table 1). Additionally, IRcdpk4/5 plants display over-activated SIPK, and genetic analysis suggests that the increased SIPK activity in IRcdpk4/5 plants is a consequence of abnormally high JA levels and is dependent on COI1 [95]. However, the molecular mechanism by which NaCDPK4 and NaCDPK5 affect JA biosynthesis remains unclear. Consistent with the role of NO in plant-herbivore interactions, NaNOA1-silenced plants exhibit elevated herbivory-induced JA but reduced JA-Ile levels [96] (Table 1). The high JA level in NaNOA1-silenced plants is unrelated to MAPK activity or the transcription levels of JA biosynthesis genes NaGLA1, NaLOX3, NaAOS, NaAOC, and NaOPR3, while the reduced JA-Ile levels are not due to impaired JAR activity, JA-Ile conversion rates, or limited Ile supply [96]. NaJIH1 encodes a novel homeostatic step in JA metabolism, which along with the cytochrome p450 enzyme-mediated hydroxylation and carboxylation of JA-Ile, rapidly attenuates the JA-Ile burst. This enables the plant to adjust direct and indirect defenses to resist herbivore attacks under natural conditions [97] (Table 1). Recently, another MAPK identified in N. attenuata, NaMPK4, has emerged as a negative regulator of M. sexta-induced JA accumulation [99] (Table 1). Intriguingly, while silencing NaMPK4 substantially enhances the resistance to the specialist M. sexta, irmpk4 ircoi1 plants still largely maintain a higher level of resistance despite being severely affected in the levels of all known JA-regulated anti-herbivory compounds [99]. This suggests that NaMPK4 suppresses the accumulation of certain unknown defense compounds in a JA signaling-independent manner. In summary, these findings not only deepen our understanding of how plants combat herbivores through multiple levels of signal integration but also offer potential targets for future agricultural biotechnologies to enhance crop resistance to herbivores. Through these integrated regulatory networks, plants demonstrate complex and flexible survival strategies when faced with herbivorous pressures.
Recent advancements in the study of plant-herbivore interactions using tobacco as a model plant have significantly expanded our understanding of defense mechanisms and signaling networks. Discoveries such as new defense metabolites and the exploration of their functions, along with the elucidation of hormone signaling regulatory networks, provide important references for future crop genetic improvement. For instance, gene editing technologies can be employed to precisely modify key genes related to defense in the crop genome, thereby enhancing the crop’s resistance. Additionally, synthetic biology techniques can be utilized to introduce the synthesis pathways of critical defense metabolites into other crops, thereby enhancing their resistance and adaptability. However, there are still many important unanswered questions throughout the entire process, starting from the onset of herbivore attacks to the deployment of chemical defenses in tobacco plants. For instance, one of the abundant FACs in M. sexta OS, known as 18:3-Glu, undergoes rapid metabolism into various derivatives upon contact with injured leaf tissue [104]. Some of these derivatives are active elicitors, triggering defense reactions [104]. The reason behind plants modifying FACs into different forms rather than recognizing them directly remains unclear. Can these different forms of derivatives act as signaling molecules for different receptors and mediate distinct signal transduction mechanisms? How are the genes encoding herbivore elicitor receptors activated? Furthermore, how is the specificity of MAPK-substrate achieved after herbivore elicitors initiate signaling cascades? The interaction between different hormone signaling pathways plays a crucial role in resource allocation, defense, and growth trade-offs in plants. The crosstalk nodes of these hormones form the core of the hormone regulatory network. How do these different crosstalk nodes accurately respond to various external signals? Answering these unresolved questions is vital for a deeper understanding of the interactions between tobacco, other plants, and herbivores.
Past studies have traditionally used reverse genetics methods to investigate the role of PSMs in defense responses. However, recent advancements in natural history-guided multi-omics analysis technologies (such as genomics, proteomics, transcriptomics, metabolomics, and phenomics) have allowed for the linkage of phenotypes, metabolites, and genes. This linkage provides a foundation for exploring the genetic basis of plant defense metabolites. By utilizing predictive models based on high-throughput omics data, researchers can generate, test, correct, and apply new biological hypotheses to the development of insect-resistant crops through synthetic engineering. The comprehensive study of plant and insect interactions also yields valuable knowledge for integrated pest management, as well as the development of plant repellents or environmentally friendly pesticides.
Acknowledgement: None.
Funding Statement: This work was supported by the Project of China National Tobacco Corporation (Grant Number 110202102007) and the Project of Hubei Tobacco Company (Grant Number 027Y2021-005).
Author Contributions: Writing—original draft preparation, G.S., and X.Z.; illustration, Y.L.; writing—review and editing, D.L., L.C., and Z.C.; supervision and funding acquisition, Z.C. and S.L. All authors have read and agreed to the published version of the manuscript.
Availability of Data and Materials: Not applicable.
Ethics Approval: Not applicable.
Conflicts of Interest: The authors declare that they have no known competing financial interests or personal relationships, as well as the Shiyan Branch of Hubei Tobacco Company that could have appeared to influence the work reported in this paper.
References
1. Rodriguez R, Redman R. More than 400 million years of evolution and some plants still can’t make it on their own: plant stress tolerance via fungal symbiosis. J Exp Bot. 2008;59:1109–14. [Google Scholar] [PubMed]
2. del Campo ML, Miles CI, Schroeder FC, Mueller C, Booker R, Renwick JA. Host recognition by the tobacco hornworm is mediated by a host plant compound. Nat. 2001;411:186–9. [Google Scholar]
3. del Campo M, Renwick JAA. Induction of host specificity in larvae of Manduca sexta: chemical dependence controlling host recognition and developmental rate. Chemoecol. 2000;10:115–21. [Google Scholar]
4. Ray S, Casteel CL. Effector-mediated plant-virus-vector interactions. Plant Cell. 2022;34:1514–31. [Google Scholar] [PubMed]
5. Backmann P, Grimm V, Jetschke G, Lin Y, Vos M, Baldwin IT, et al. Delayed chemical defense: timely expulsion of herbivores can reduce competition with neighboring plants. Am Nat. 2019;193:125–39. [Google Scholar] [PubMed]
6. Li R, Llorca LC, Schuman MC, Wang Y, Wang L, Joo Y, et al. ZEITLUPE in the roots of wild tobacco regulates jasmonate-mediated nicotine biosynthesis and resistance to a generalist herbivore. Plant Physiol. 2018;177(2):833–46. [Google Scholar] [PubMed]
7. Lee G, Joo Y, Kim SG, Baldwin IT. What happens in the pith stays in the pith: tissue-localized defense responses facilitate chemical niche differentiation between two spatially separated herbivores. Plant J. 2017;92(3):414–25. [Google Scholar] [PubMed]
8. Joo Y, Kim H, Kang M, Lee G, Choung S, Kaur H, et al. Pith-specific lignification in Nicotiana attenuata as a defense against a stem-boring herbivore. New Phytol. 2021;232(1):332–44. [Google Scholar] [PubMed]
9. Kaur H, Heinzel N, Schöttner M, Baldwin IT, Gális I. R2R3-NaMYB8 regulates the accumulation of phenylpropanoid-polyamine conjugates, which are essential for local and systemic defense against insect herbivores in Nicotiana attenuata. Plant Physiol. 2010;152(3):1731–47. [Google Scholar] [PubMed]
10. Bai Y, Yang C, Halitschke R, Paetz C, Kessler D, Burkard K, et al. Natural history–guided omics reveals plant defensive chemistry against leafhopper pests. Science. 2022;375:eabm2948. [Google Scholar] [PubMed]
11. Schuman MC, Barthel K, Baldwin IT. Herbivory-induced volatiles function as defenses increasing fitness of the native plant Nicotiana attenuata in nature. elife. 2012;1:e00007. [Google Scholar] [PubMed]
12. Kessler Á, Baldwin IT. Defensive function of herbivore-induced plant volatile emissions in nature. Science. 2001;291(5511):2141–4. [Google Scholar] [PubMed]
13. Paudel Timilsena B, Seidl‐Adams I, Tumlinson JH. Herbivore-specific plant volatiles prime neighboring plants for nonspecific defense responses. Plant Cell Environ. 2020;43(3):787–800. [Google Scholar] [PubMed]
14. Wink M. Evolution of secondary metabolites from an ecological and molecular phylogenetic perspective. Phytochem. 2003;64(1):3–19. [Google Scholar]
15. Purrington CB. Costs of resistance. Curr Opin Plant Biol. 2000;3(4):305–8. [Google Scholar] [PubMed]
16. Wang L, Wu J. The essential role of jasmonic acid in plant-herbivore interactions--using the wild tobacco Nicotiana attenuata as a model. J Genet Genomics. 2013;40(12):597–606. [Google Scholar] [PubMed]
17. Onkokesung N, Baldwin IT, Gális I. The role of jasmonic acid and ethylene crosstalk in direct defense of Nicotiana attenuata plants against chewing herbivores. Plant Signal Behav. 2010;5:1305–7. [Google Scholar] [PubMed]
18. Figon F, Baldwin IT, Gaquerel E. Ethylene is a local modulator of jasmonate-dependent phenolamide accumulation during Manduca sexta herbivory in Nicotiana attenuata. Plant Cell Environ. 2021;44:964–81. [Google Scholar] [PubMed]
19. Diezel C, von Dahl CC, Gaquerel E, Baldwin IT. Different lepidopteran elicitors account for cross-talk in herbivory-induced phytohormone signaling. Plant Physiol. 2009;150:1576–86. [Google Scholar] [PubMed]
20. Xu S, Zhou W, Baldwin IT. The rapidly evolving associations among herbivore associated elicitor-induced phytohormones in Nicotiana. Plant Signal Behav. 2015;10:e1035850. [Google Scholar] [PubMed]
21. Ehrlich PR, Raven PH. Butterflies and plants: a study in coevolution. Evol. 1964;18:586–608. [Google Scholar]
22. Divekar PA, Narayana S, Divekar BA, Kumar R, Gadratagi BG, Ray A, et al. Plant secondary metabolites as defense tools against herbivores for sustainable crop protection. Int J Mol Sci. 2022;23(5):2690. [Google Scholar] [PubMed]
23. Halitschke R, Keßler A, Kahl J, Lorenz A, Baldwin IT. Ecophysiological comparison of direct and indirect defenses in Nicotiana attenuata. Oecologia. 2000;124(3):408–17. [Google Scholar] [PubMed]
24. Steppuhn A, Gase K, Krock B, Halitschke R, Baldwin IT. Nicotine’s defensive function in nature. PLoS Biol. 2004;2:E217. [Google Scholar] [PubMed]
25. Halpern SL, Adler LS, Wink M. Leaf herbivory and drought stress affect floral attractive and defensive traits in Nicotiana quadrivalvis. Oecologia. 2010;163(4):961–71. [Google Scholar] [PubMed]
26. Lichman BR. The scaffold-forming steps of plant alkaloid biosynthesis. Nat Prod Rep. 2021;38(1):103–29. [Google Scholar] [PubMed]
27. Kaminski KP, Bovet L, Laparra H, Lang G, de Palo D, Sierro N, et al. Alkaloid chemophenetics and transcriptomics of the Nicotiana genus. Phytochemistry. 2020;177:112424. [Google Scholar] [PubMed]
28. Saitoh F, Noma M, Kawashima N. The alkaloid contents of sixty Nicotiana species. Phytochem. 1985;24:477–80. [Google Scholar]
29. Pinto CF, Torrico-Bazoberry D, Penna M, Cossio-Rodríguez R, Cocroft R, Appel H, et al. Chemical responses of Nicotiana tabacum (Solanaceae) induced by vibrational signals of a generalist herbivore. J Chem Ecol. 2019;45:708–14. [Google Scholar] [PubMed]
30. Matsuda K, Kanaoka S, Akamatsu M, Sattelle DB. Diverse actions and target-site selectivity of neonicotinoids: structural insights. Mol Pharmacol. 2009;76:1–10. [Google Scholar] [PubMed]
31. Wink M, Theile V. Alkaloid tolerance in Manduca sexta and phylogenetically related sphingids (Lepidoptera: Sphingidae). Chemoecology. 2002;12:29–46. [Google Scholar]
32. Barbosa P, Saunders JA, Kemper J, Trumbule R, Olechno J, Martinat P. Plant allelochemicals and insect parasitoids effects of nicotine on Cotesia congregata (say) (Hymenoptera: Braconidae) and Hyposoter annulipes (Cresson) (Hymenoptera: Ichneumonidae). J Chem Ecol. 1986;12:1319–28. [Google Scholar] [PubMed]
33. Singh S, Kaur I, Kariyat R. The multifunctional roles of polyphenols in plant-herbivore interactions. Int J Mol Sci. 2021;22(3):1442. [Google Scholar] [PubMed]
34. Jun SY, Sattler SA, Cortez GS, Vermerris W, Sattler SE, Kang CH. Biochemical and structural analysis of substrate specificity of a phenylalanine ammonia-lyase. Plant Physiol. 2018;176(2):1452–68. doi:10.1104/pp.17.01608. [Google Scholar] [PubMed] [CrossRef]
35. Vogt T. Phenylpropanoid biosynthesis. Mol Plant. 2010;3(1):2–20. [Google Scholar] [PubMed]
36. Dong N, Lin HX. Contribution of phenylpropanoid metabolism to plant development and plant-environment interactions. J Integr Plant Biol. 2021;63(1):180–209. [Google Scholar] [PubMed]
37. Kundu A, Vadassery J. Chlorogenic acid-mediated chemical defence of plants against insect herbivores. Plant Biol J. 2019;21(2):185–9. [Google Scholar]
38. Liu X, Vrieling K, Klinkhamer PGL. Interactions between plant metabolites affect herbivores: a study with pyrrolizidine alkaloids and chlorogenic acid. Front Plant Sci. 2017;8:903. [Google Scholar] [PubMed]
39. Vanholme R, de Meester B, Ralph J, Boerjan W. Lignin biosynthesis and its integration into metabolism. Curr Opin Biotechnol. 2019;56:230–9. [Google Scholar] [PubMed]
40. Diezel C, Kessler D, Baldwin IT. Pithy protection: Nicotiana attenuata’s jasmonic acid-mediated defenses are required to resist stem-boring weevil larvae. Plant Physiol. 2011;155:1936–46. [Google Scholar] [PubMed]
41. Roumani M, Besseau S, Gagneul D, Robin C, Larbat R. Phenolamides in plants: an update on their function, regulation, and origin of their biosynthetic enzymes. J Exp Bot. 2021;72:2334–55. [Google Scholar] [PubMed]
42. Hunziker P, Lambertz SK, Weber K, Crocoll C, Halkier BA, Schulz A. Herbivore feeding preference corroborates optimal defense theory for specialized metabolites within plants. Proc Natl Acad Sci USA. 2021;118:e2111977118. [Google Scholar]
43. Onkokesung N, Gaquerel E, Kotkar H, Kaur H, Baldwin IT, Galis I. MYB8 controls inducible phenolamide levels by activating three novel hydroxycinnamoyl-coenzyme A: polyamine transferases in Nicotiana attenuata. Plant Physiol. 2012;158:389–407. [Google Scholar] [PubMed]
44. Matsui K. Green leaf volatiles: hydroperoxide lyase pathway of oxylipin metabolism. Curr Opin Plant Biol. 2006;9(3):274–80. [Google Scholar] [PubMed]
45. Halitschke R, Stenberg JA, Kessler D, Kessler A, Baldwin IT. Shared signals-‘alarm calls’ from plants increase apparency to herbivores and their enemies in nature. Ecol Lett. 2008;11(1):24–34. [Google Scholar] [PubMed]
46. Allmann S, Baldwin IT. Insects betray themselves in nature to predators by rapid isomerization of green leaf volatiles. Sci. 2010;329(5995):1075–8. [Google Scholar]
47. de Moraes CM, Mescher MC, Tumlinson JH. Caterpillar-induced nocturnal plant volatiles repel conspecific females. Nat. 2001;410(6828):577–80. [Google Scholar]
48. Li CF, Li QR, Bai M, Lv YS YS, Jiao Y Y. Advances in the biosynthesis of terpenoids and their ecological functions in plant resistance. Int J Mol Sci. 2023;24:11561. [Google Scholar] [PubMed]
49. Jassbi AR, Zare S, Asadollahi M, Schuman MC. Ecological roles and biological activities of specialized metabolites from the genus Nicotiana. Chem Rev. 2017;117:12227–80. [Google Scholar] [PubMed]
50. Hummelbrunner LA, Isman MB. Acute, sublethal, antifeedant, and synergistic effects of monoterpenoid essential oil compounds on the tobacco cutworm, Spodoptera litura (Lep., Noctuidae). J Agric Food Chem. 2001;49:715–20. [Google Scholar] [PubMed]
51. Schuman MC, Heinzel N, Gaquerel E, Svatos A, Baldwin IT. Polymorphism in jasmonate signaling partially accounts for the variety of volatiles produced by Nicotiana attenuata plants in a native population. New Phytol. 2009;183:1134–48. [Google Scholar] [PubMed]
52. Halitschke R, Schittko U, Pohnert G, Boland W, Baldwin IT. Molecular interactions between the specialist herbivore Manduca sexta (Lepidoptera, Sphingidae) and its natural host Nicotiana attenuata. III. Fatty acid-amino acid conjugates in herbivore oral secretions are necessary and sufficient for herbivore-specific plant responses. Plant Physiol. 2001;125:711–7. [Google Scholar] [PubMed]
53. SimpsonJr RD, Johnson AW, Severson RF. Leaf surface chemistry of tobacco budworm resistant and susceptible tobacco grown in the field and greenhouse at different fertilization rates. Environ Sci Biol. 1985;29:44–6. [Google Scholar]
54. Jassbi AR, Gase K, Hettenhausen C, Schmidt A, Baldwin IT. Silencing geranylgeranyl diphosphate synthase in Nicotiana attenuata dramatically impairs resistance to tobacco hornworm. Plant Physiol. 2008;146(3):974–86. [Google Scholar] [PubMed]
55. Jassbi AR, Zamanizadehnajari S, Kessler D, Baldwin IT. A new acyclic diterpene glycoside from Nicotiana attenuata with a mild deterrent effect on feeding manduca sexta larvae. Zeitschrift für Naturforschung B. 2006;61(9):1138–42. [Google Scholar]
56. Heiling S, Schuman MC, Schoettner M, Mukerjee P, Berger B, Schneider B, et al. Jasmonate and ppHsystemin regulate key Malonylation steps in the biosynthesis of 17-Hydroxygeranyllinalool diterpene glycosides, an abundant and effective direct defense against herbivores in Nicotiana attenuata. Plant Cell. 2010;22(1):273–92. [Google Scholar] [PubMed]
57. Wu J, Baldwin IT. New insights into plant responses to the attack from insect herbivores. Annu Rev Genet. 2010;44(1):1–24. [Google Scholar] [PubMed]
58. Heidel AJ, Baldwin IT. Microarray analysis of salicylic acid-and jasmonic acid-signalling in responses of Nicotiana attenuata to attack by insects from multiple feeding guilds. Plant Cell Environ. 2004;27(11):1362–73. [Google Scholar]
59. Halitschke R, Baldwin IT. Antisense LOX expression increases herbivore performance by decreasing defense responses and inhibiting growth-related transcriptional reorganization in Nicotiana attenuata. Plant J. 2003;36(6):794–807. [Google Scholar] [PubMed]
60. Portman SL, Kariyat RR, Johnston MA, Stephenson AG, Marden JH. Inbreeding compromises host plant defense gene expression and improves herbivore survival. Plant Signal Behav. 2015;10(5):e998548. [Google Scholar] [PubMed]
61. Kang JH, Wang L, Giri A, Baldwin IT. Silencing threonine deaminase and JAR4 in Nicotiana attenuata impairs jasmonic acid-isoleucine-mediated defenses against Manduca sexta. Plant Cell. 2006;18(11):3303–20. [Google Scholar] [PubMed]
62. Fonseca S, Chini A, Hamberg M, Adie B, Porzel A, Kramell R, et al. (+)-7-iso-Jasmonoyl-L-isoleucine is the endogenous bioactive jasmonate. Nat Chem Biol. 2009;5(5):344–50. [Google Scholar] [PubMed]
63. Wang L, Halitschke R, Kang JH, Berg A, Harnisch F, Baldwin IT. Independently silencing two JAR family members impairs levels of trypsin proteinase inhibitors but not nicotine. Planta. 2007;226:159–67. [Google Scholar] [PubMed]
64. Kallenbach M, Bonaventure G, Gilardoni PA, Wissgott A, Baldwin IT. Empoasca leafhoppers attack wild tobacco plants in a jasmonate-dependent manner and identify jasmonate mutants in natural populations. Proc Natl Acad Sci USA. 2012;109:E1548–57. [Google Scholar] [PubMed]
65. Skibbe M, Qu N, Galis I, Baldwin IT. Induced plant defenses in the natural environment: Nicotiana attenuata WRKY3 and WRKY6 coordinate responses to herbivory. Plant Cell. 2008;20:1984–2000. [Google Scholar] [PubMed]
66. Shoji T, Hashimoto T, Saito K. Genetic regulation and manipulation of nicotine biosynthesis in tobacco: strategies to eliminate addictive alkaloids. J Exp Bot. 2024;75(6):1741–53. [Google Scholar]
67. Shoji T, Yamada Y, Hashimoto T. Jasmonate induction of putrescine N-methyltransferase genes in the root of Nicotiana sylvestris. Plant Cell Physiol. 2000;41:831–9. [Google Scholar] [PubMed]
68. Baldwin IT, Schmelz EA. Constraints on an induced defense: the role of leaf area. Oecol. 1994;97:424–30. [Google Scholar]
69. Baldwin IT. Mechanism of damage-induced alkaloid production in wild tobacco. J Chem Ecol. 1989;15:1661–80. [Google Scholar] [PubMed]
70. Baldwin IT, Karb MJ, Ohnmeiss TE. Allocation of 15N from nitrate to nicotine: production and turnover of a damage-induced mobile defense. Environ Sci Biol. 1994;75:1703–13. [Google Scholar]
71. Baldwin IT. Inducible nicotine production in native nicotiana as an example of adaptive phenotypic plasticity. J Chem Ecol. 1999;25:3–30. [Google Scholar]
72. Hu C, Li YT, Hao YX, Hao GF. Molecular interaction network of plant-herbivorous insects. Adv Agrochem. 2023;3:74–82. [Google Scholar]
73. Onkokesung N, Gális I, von Dahl CC, Matsuoka K, Saluz HP, Baldwin IT. Jasmonic acid and ethylene modulate local responses to wounding and simulated herbivory in Nicotiana attenuata leaves. Plant Physiol. 2010;153:785–98. [Google Scholar] [PubMed]
74. Yang DH, Hettenhausen C, Baldwin IT, Wu J. BAK1 regulates the accumulation of jasmonic acid and the levels of trypsin proteinase inhibitors in Nicotiana attenuata’s responses to herbivory. J Exp Bot. 2011;62:641–52. [Google Scholar] [PubMed]
75. Yang DH, Baldwin IT, Wu J. Silencing brassinosteroid receptor BRI1 impairs herbivory-elicited accumulation of jasmonic acid-isoleucine and diterpene glycosides, but not jasmonic acid and trypsin proteinase inhibitors in Nicotiana attenuata. J Integr Plant Biol. 2013;55(6):514–26. [Google Scholar] [PubMed]
76. Dinh ST, Baldwin IT, Galis I. The HERBIVORE ELICITOR-REGULATED1 gene enhances abscisic acid levels and defenses against herbivores in Nicotiana attenuata plants. Plant Physiol. 2013;162(4):2106–24. [Google Scholar] [PubMed]
77. Ghorbel M, Brini F, Sharma A, Landi M. Role of jasmonic acid in plants: the molecular point of view. Plant Cell Rep. 2021;40(8):1471–94. [Google Scholar] [PubMed]
78. Rayapuram C, Baldwin IT. Increased SA in NPR1-silenced plants antagonizes JA and JA-dependent direct and indirect defenses in herbivore-attacked Nicotiana attenuata in nature. Plant J. 2007;52(4):700–15. [Google Scholar] [PubMed]
79. Gilardoni PA, Hettenhausen C, Baldwin IT, Bonaventure G. Nicotiana attenuata LECTIN RECEPTOR KINASE1 suppresses the insect-mediated inhibition of induced defense responses during Manduca sexta herbivory. Plant Cell. 2011;23(9):3512–32. [Google Scholar] [PubMed]
80. Großkinsky DK, Naseem M, Abdelmohsen UR, Plickert N, Engelke T, Griebel T, et al. Cytokinins mediate resistance against Pseudomonas syringae in tobacco through increased antimicrobial phytoalexin synthesis independent of salicylic acid signaling. Plant Physiol. 2011;157(2):815–30. [Google Scholar]
81. Dervinis C, Frost CJ, Lawrence SD, Novak NG, Davis JM. Cytokinin primes plant responses to wounding and reduces insect performance. J Plant Growth Regul. 2010;29(3):289–96. [Google Scholar]
82. Schäfer M, Meza‐Canales ID, Brütting C, Baldwin IT, Meldau S. Cytokinin concentrations and CHASE-DOMAIN CONTAINING HIS KINASE 2 (NaCHK2)- and NaCHK3-mediated perception modulate herbivory-induced defense signaling and defenses in Nicotiana attenuata. New Phytol. 2015;207(3):645–58. [Google Scholar]
83. Heinrich M, Baldwin IT, Wu J. Two mitogen-activated protein kinase kinases, MKK1 and MEK2, are involved in wounding- and specialist lepidopteran herbivore Manduca sexta-induced responses in Nicotiana attenuata. J Exp Bot. 2011;62(12):4355–65. [Google Scholar] [PubMed]
84. Hettenhausen C, Schuman MC, Wu J. MAPK signaling: a key element in plant defense response to insects. Insect Sci. 2015;22(2):157–64. [Google Scholar] [PubMed]
85. Seo S, Okamoto M, Seto H, Ishizuka K, Sano H, Ohashi Y. Tobacco MAP kinase: a possible mediator in wound signal transduction pathways. Science. 1995;270(5244):1988–92. [Google Scholar] [PubMed]
86. Song X, Li Y, Cao X, Qi Y. MicroRNAs and their regulatory roles in plant-environment interactions. Annu Rev Plant Biol. 2019;70(1):489–525. [Google Scholar] [PubMed]
87. Pradhan M, Pandey P, Gase K, Sharaff M, Singh RK, Sethi A, et al. Argonaute 8 (AGO8) mediates the elicitation of direct defenses against herbivory. Plant Physiol. 2017;175(2):927–46. [Google Scholar] [PubMed]
88. Bozorov T, Pandey S, Dinh ST, Kim SG, Heinrich M, Gase K, et al. DICER-like proteins and their role in plant-herbivore interactions in Nicotiana attenuata. J Integr Plant Biol. 2012;54(3):189–206. [Google Scholar] [PubMed]
89. Pandey SP, Baldwin IT. RNA-directed RNA polymerase 1 (RdR1) mediates the resistance of Nicotiana attenuata to herbivore attack in nature. Plant J. 2007;50(1):40–53. [Google Scholar] [PubMed]
90. Meldau S, Baldwin IT, Wu J. SGT1 regulates wounding- and herbivory-induced jasmonic acid accumulation and Nicotiana attenuata’s resistance to the specialist lepidopteran herbivore Manduca sexta. New Phytol. 2011;189(4):1143–56. [Google Scholar] [PubMed]
91. Wünsche H, Baldwin IT, Wu J. S-Nitrosoglutathione reductase (GSNOR) mediates the biosynthesis of jasmonic acid and ethylene induced by feeding of the insect herbivore Manduca sexta and is important for jasmonate-elicited responses in Nicotiana attenuata. J Exp Bot. 2011;62(13):4605–16. [Google Scholar]
92. Li R, Jin J, Xu J, Wang L, Li J, Lou Y, Baldwin IT. Long non‐coding RNAs associate with jasmonate‐mediated plant defence against herbivores. Plant Cell Environ. 2021;44(3):982–94. [Google Scholar] [PubMed]
93. Mitra S, Baldwin IT. RuBPCase activase (RCA) mediates growth-defense trade-offs: silencing RCA redirects jasmonic acid (JA) flux from JA-isoleucine to methyl jasmonate (MeJA) to attenuate induced defense responses in Nicotiana attenuata. New Phytol. 2014;201(4):1385–95. [Google Scholar] [PubMed]
94. Giri AP, Wünsche H, Mitra S, Zavala JA, Muck A, Svatos A, et al. Molecular interactions between the specialist herbivore Manduca sexta (Lepidoptera, Sphingidae) and its natural host Nicotiana attenuata. VII. Changes in the plant’s proteome. Plant Physiol. 2006;142:1621–41. [Google Scholar] [PubMed]
95. Yang DH, Hettenhausen C, Baldwin IT, Wu J. Silencing Nicotiana attenuata calcium-dependent protein kinases, CDPK4 and CDPK5, strongly up-regulates wound- and herbivory-induced jasmonic acid accumulations. Plant Physiol. 2012;159:1591–607. [Google Scholar] [PubMed]
96. Wünsche H, Baldwin IT, Wu J. Silencing NOA1 elevates herbivory-induced jasmonic acid accumulation and compromises most of the carbon-based defense metabolites in Nicotiana attenuata. J Integr Plant Biol. 2011;53:619–31. [Google Scholar]
97. Woldemariam MG, Onkokesung N, Baldwin IT, Galis I. Jasmonoyl-L-isoleucine hydrolase 1 (JIH1) regulates jasmonoyl-L-isoleucine levels and attenuates plant defenses against herbivores. Plant J. 2012;72:758–67. [Google Scholar] [PubMed]
98. Oh Y, Baldwin IT, Gális I. NaJAZh regulates a subset of defense responses against herbivores and spontaneous leaf necrosis in Nicotiana attenuata plants. Plant Physiol. 2012;159:769–88. [Google Scholar] [PubMed]
99. Hettenhausen C, Baldwin IT, Wu J. Nicotiana attenuata MPK4 suppresses a novel jasmonic acid (JA) signaling-independent defense pathway against the specialist insect Manduca sexta, but is not required for the resistance to the generalist Spodoptera littoralis. New Phytol. 2013;199:787–99. [Google Scholar] [PubMed]
100. Mitra S, Baldwin IT. Independently silencing two photosynthetic proteins in Nicotiana attenuata has different effects on herbivore resistance. Plant Physiol. 2008;148:1128–38. [Google Scholar] [PubMed]
101. Woldemariam MG, Dinh ST, Oh Y, Gaquerel E, Baldwin IT, Galis I. NaMYC2 transcription factor regulates a subset of plant defense responses in Nicotiana attenuata. BMC Plant Biol. 2013;13:73. [Google Scholar] [PubMed]
102. Wu J, Hettenhausen C, Meldau S, Baldwin IT. Herbivory rapidly activates MAPK signaling in attacked and unattacked leaf regions but not between leaves of Nicotiana attenuata. Plant Cell. 2007;19:1096–122. [Google Scholar] [PubMed]
103. Pandey SP, Shahi P, Gase K, Baldwin IT. Herbivory-induced changes in the small-RNA transcriptome and phytohormone signaling in Nicotiana attenuata. Proc Natl Acad Sci USA. 2008;105:4559–64. [Google Scholar] [PubMed]
104. VanDoorn A, Kallenbach M, Borquez AA, Baldwin IT, Bonaventure G. Rapid modification of the insect elicitor N-linolenoyl-glutamate via a lipoxygenase-mediated mechanism on Nicotiana attenuata leaves. BMC Plant Biol. 2010;10:164. [Google Scholar] [PubMed]
Cite This Article
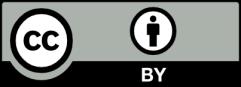
This work is licensed under a Creative Commons Attribution 4.0 International License , which permits unrestricted use, distribution, and reproduction in any medium, provided the original work is properly cited.