Open Access
ARTICLE
Genome-Wide Exploration of the Grape GLR Gene Family and Differential Responses of VvGLR3.1 and VvGLR3.2 to Low Temperature and Salt Stress
1 The Engineering Research Institute of Agriculture and Forestry, Ludong University, Yantai, 264025, China
2 College of Agriculture, Ludong University, Yantai, 264025, China
* Corresponding Author: Chunyan Yu. Email:
# These authors contributed equally to this work
Phyton-International Journal of Experimental Botany 2024, 93(3), 533-549. https://doi.org/10.32604/phyton.2024.049417
Received 06 January 2024; Accepted 05 February 2024; Issue published 28 March 2024
Abstract
Grapes, one of the oldest tree species globally, are rich in vitamins. However, environmental conditions such as low temperature and soil salinization significantly affect grape yield and quality. The glutamate receptor (GLR) family, comprising highly conserved ligand-gated ion channels, regulates plant growth and development in response to stress. In this study, 11 members of the VvGLR gene family in grapes were identified using whole-genome sequence analysis. Bioinformatic methods were employed to analyze the basic physical and chemical properties, phylogenetic trees, conserved domains, motifs, expression patterns, and evolutionary relationships. Phylogenetic and collinear analyses revealed that the VvGLRs were divided into three subgroups, showing the high conservation of the grape GLR family. These members exhibited 2 glutamate receptor binding regions (GABAb and GluR) and 3–4 transmembrane regions (M1, M2, M3, and M4). Real-time quantitative PCR analysis demonstrated the sensitivity of all VvGLRs to low temperature and salt stress. Subsequent localization studies in Nicotiana tabacum verified that VvGLR3.1 and VvGLR3.2 proteins were located on the cell membrane and cell nucleus. Additionally, yeast transformation experiments confirmed the functionality of VvGLR3.1 and VvGLR3.2 in response to low temperature and salt stress. These findings highlight the significant role of the GLR family, a highly conserved group of ion channels, in enhancing grape stress resistance. This study offers new insights into the grape GLR gene family, providing fundamental knowledge for further functional analysis and breeding of stress-resistant grapevines.Keywords
Supplementary Material
Supplementary Material FileGlutamate receptor-like (GLR) protein, a crucial Ca2+ channel protein, facilitates Ca2+ influx from the extracellular space to the cytoplasm, inducing changes in the Ca2+ concentration [1]. There are two categories of glutamate receptors: metabotropic glutamate receptors (mGluRs) and ionotropic glutamate receptors (iGluRs). IGluRs are ligand-gated cation channels that respond to L-amino acids (such as glutamic acid and glycine). They regulate excitatory nerve signal transmission and neuronal development in mammals [1] and are primarily expressed on the plasma membrane. The plant glutamate receptor (GLR) gene sequence is highly homologous to that of the animal iGluR. The earliest analysis of GLR in plants revealed 20 sequences homologous to iGluRs (AtGLRs) in Arabidopsis thaliana [2], categorized into three subfamilies: AtGLR1, AtGLR2, and AtGLR3 [3]. GLR proteins are highly conserved and feature two glutamate receptor binding regions (GABAb and GluR) and 3-4 transmembrane regions (M1, M2, M3, and M4).
Glutamate receptors (GLRs) have been extensively studied in many plants, including Arabidopsis, rice, pear, apple, tomato, alfalfa, and sugarcane [4–10]. GLRs play an important role in regulating plant growth, influencing developmental phase such as seed germination [9], root development [10], apical meristem division and apoptosis [11], stomatal opening [9], pollen tube growth and morphogenesis [12,13], photosensitivity/phototropism in plants [5], light signal transduction [14], C/N balance regulation [15], water balance [16], calcium ion transport, and calcium-regulated stomatal movement [17]. Beyond growth and development, GLRs participate in biological and abiotic stress responses, such as alleviation of the toxic effects of aluminum ions [18]. For instance, SlGLR3.3 and SlGLR3.5 enhance drought tolerance in tomatoes [19], and MtGLR is involved in nitric oxide production in alfalfa during drought [7]. OsGLR1 and OsGLR2 improve drought tolerance in rice under drought stress conditions [20]. In Arabidopsis, AtGLR1.1 controls development and water loss by regulating abscisic acid biosynthesis and signal transduction pathways [15]. Studies have also indicated that AtGLR3.7 responds to salt stress [21], whereas AtGLR1.2 and AtGLR1.3 enhance low temperature tolerance in plants by activating the downstream CBF/DREB1 pathway through jasmonic acid (JA) accumulation under low-temperature conditions [22–25].
Current research on GLR has been confined to the aforementioned species, however, the genome-wide exploration and expression patterns of GLRs in grapes remain unclear. This study utilized bioinformatic analysis methods to elucidate protein characteristics, offering valuable support for subsequent experiments and target selection. First, 11 grape GLR family genes were identified. To delve deeper into the structural characteristics, evolutionary relationships, and biological functions of the GLR genes in grapes, a comprehensive analysis was conducted. This analysis encompassed various bioinformatic features, such as structural domains, cis-acting promoter elements, protein structures, and protein-protein interactions. These findings suggest a pivotal role for VvGLR in conferring resistance to low temperature environments and salt stress.
2.1 Genomic Identification and Characterization of VvGLRs in Grapes
To identify the GLR gene family in grapes, the Arabidopsis GLR sequence from TAIR (https://www.arabidopsis.org) and grape GLR gene family information from the Ensemble database (https://plants.ensembl.org/index.html; release 51) served as a filter library. Using 20 Arabidopsis GLRs as a reference [2], local BLAST searches were conducted on grape protein sequences. Additionally, the login number and Hidden Markov model (HMM) file from pfam facilitated a hidden Markov model search for Lig_chan (PF00060) candidate proteins in two databases (Ensemble plants and Uniport) via HMMER 3.0. By comparing the outcomes of these screening methods and eliminating redundant protein sequences, grape GLR family members were ultimately identified [8]. The online platform Expasy (www.expasy.org/) was applied to examine the molecular weight (MW), isoelectric point (PI), and total mean hydrophobicity (GRAVY) of the proteins. The online website (http://www.csbio.sjtu.edu.cn/bioinf/Cell-PLoc-2) was applied to predicate the subcellular localization of the VvGLR gene family [8].
2.2 Chromosome Distribution, Conserved Motifs, Gene Structure, and Three-Dimensional Domain Analysis
For gene chromosome positioning mapping, the GFF format file from the Ensemble plant database was obtained, and Graphics in TBtools were utilized. To investigate the conserved domain characteristics of the GLR gene family [26], the MEME database was applied to search conserved motifs.
2.3 Phylogenetic Analysis of GLRs from Different Plant Species
Sequences of GLR proteins from maize, rice, and Arabidopsis were submitted to ClustalW multiple sequence alignment using MUSCLE in MEGA 7.0. The NJ approach was utilized to generate a phylogenetic tree [2,10,27]. The p-distance module was applied for amino acid model selection, and 1000 bootstraps were performed. The major branches consistently displayed values between 95% and 100% [3]. Subsequently, the tree was refined using the online platform ITOL (https://itol.embl.de/) [10].
2.4 Synteny Relationship Analysis
To describe the collinearity of GLRs among diverse plant species, the genomic sequences of Arabidopsis and grape were obtained from Ensembl Plant database. The collinearity of genes between grape and Arabidopsis was determined by TBtools using Multiple Collinearity Scan Toolkit module [28].
2.5 Analysis of cis-Acting Elements of Grape GLR Family Promoter
For predicting the cis-acting elements of the grape GLRs promoter, the 2,000 bp sequence upstream of translation initiation codon of VvGLRs was downloaded from the Ensembl Plant database, and the PlantCARE database (http://bioinformatics.psb.ugent.be/webtools/plantcare/html/) was used [29]. Subsequently, the obtained information was visualized using TBtools software [8].
2.6 Prediction of Protein Structure and Interactions Based on the Network
A three-dimensional structural domain research of the VvGLR protein was conducted through SWISS-MODEL database (https://swissmodel.expasy.org/). To ensure model accuracy, the protein with the highest similarity to AtGLR was served as the template. The online website STRING (https://cn.string-db.org) was employed to predict genes that interact with the VvGLR family [21]. The OmicStudio platform (https://www.omicstudio.cn/doc) was employed to perform GO enrichment on genes that interacted with each other [8].
To estimate gene expression, the TPM (per million transcripts) and FPKM (per million thousand base fragments) of GLRs in various grape parts were downloaded from the Grape eFP Browser (https://bar.utoronto.ca/efp_grape/cgi-bin/efpWeb.cgi). Subsequently TBtool was used to create heat maps for visualization [28].
2.8 Treatment of Plant Materials with Low Temperature and Salt Stress for Quantitative PCR Analysis
In this study, tissue-cultured ‘Pinot Noir’ grape plantlets were exposed to low temperatures of 4°C and −20°C in a growth chamber with 100 μmol m−2 μs−1 light intensity and 16 h of light and 8 h of darkness. The standard culture temperature was 23°C, with consistent culture conditions. For the salt stress treatment, seven-week old tissue-culture seedlings cultivated on MS medium were transferred to Hoagland nutrient solution for 48 h to eliminate the potential effects of changing the culture conditions. Subsequently, seedlings were transferred to a solution containing either 0 or 100 mmol/L of NaCl. After 48 h of treatment, for total RNA extraction, the first and second completely grown leaves from the top of seedlings were gathered.
Total RNA from different treatments plants was extracted using the FastPure Plant Total RNA Isolation Kit (Polysaccharides & Polyphenolics–rich) (Vazyme, Nanjing, China), and the first-strand cDNA was synthesized through reverse transcription kit (Vazyme, Nanjing, China). RNA concentration and purity were estimated utilizing NanoDrop 2000 (Thermo Fisher Logical, Wilmington, DE, USA) and gel electrophoresis. To eliminate genomic DNA (gDNA) interference, RNA extraction was digested with a gDNA wiper (Vazyme, Nanjing, China) before first-strand cDNA synthesis. The SuperReal PreMix Plus (Tiangen, Beijing, China) was used for real-time quantitative PCR (qRT-PCR) analysis. Comparative CT method was used to calculate the relative expression levels [30]. VvUBQ10 served as an endogenous reference gene for sample standardization, as described in a previous study [31]. To ensure experimental accuracy, each experiment was repeated three times. The gene-specific primers used for qRT-PCR are listed in Table S1.
2.9 Subcellular Localization of Grape GLR
To pinpoint the VvGLR gene, the full lengths of VvGLR3.1 and VvGLR3.2 were initially amplified, and a GFP subcellular localization vector was constructed. Agrobacterium tumefaciens strain GV3101 carrying 35 S:: GFP, 35 S:: GFP-VvGLR3.1, and 35 S:: GFP-VvGLR3.2 was introduced into tobacco epidermal cells containing the red fluorescent proteins of H2B and a plasma membrane-located gene [4,32,33]. Tobacco epidermal cells were harvested after 2–3 d. A confocal laser microscope was used to observe and capture the fluorescence.
2.10 Vector Construction, Yeast Transformation and Stress Experiments
The protein expression vector pYES2 from Saccharomyces cerevisiae was constructed using ClonExpress II One Step Cloning Kit (Vazyme, Nanjing, China). Complete coding sequences of VvGLR3.1 and VvGLR3.2 were inserted into pYES2 and transformed into the host yeast strain INVSC1 for recombinant protein expression [34–38]. Subsequent salt stress experiments were conducted using SG-Ura (Coolaber, Beijing, China) media with NaCl concentrations of 0, 250, 500, and 750 mmol/L. The low-temperature experiment involved an initial culture at 4°C, 0°C, and −4°C for 48 h, followed by a switch to normal temperature for growth, and the growth status was observed [25].
All data underwent variance analysis using IBM SPSS Statistics 20 [2]. Variance analysis was used to identify differences, and Student’s t-test was applied to estimate the differences between mean values. Statistical significance was considered at 5% (*p < 0.05) or 1% (**p < 0.01) probability levels [2].
3.1 Eleven VvGLRs Were Identified in the Grape Genome
In order to investigate the potential function of the GLR proteins in grapes, a total of 11 GLR coding genes were identified in the grape genome. Based on the naming rules of the GLR gene family [39] and considering their chromosomal positions, they were designated as VvGLR 1.1, VvGLR2.1–VvGLR2.5, and VvGLR3.1–VvGLR3.5 (Table 1). Predicted full-length VvGLR consisted of 868 to 973 amino acid residues, with molecular weights ranging from 96.13 (VvGLR 1.1) to 108.81 kDa (VvGLR 2.5) and isoelectric points spanning from 5.59 (VvGLR 2.1) to 8.78 (VvGLR 3.3). Based on the positive total average hydrophilicity (GRAVY), all VvGLRs were predicted to be stable and hydrophobic proteins (Table 1). Subcellular localization prediction indicated that all VvGLRs were located in the plasma membrane.
3.2 Structure of VvGLR Genes and Proteins Were Highly Conserved
Examination of the chromosomal distribution of the VvGLR family genes revealed an uneven and irregular spread across chromosomes 3, 4, 7, 11, 12, and 18 (Table S2). Specifically, VvGLR3.1 was situated on chromosome 3, VvGLR2.1, VvGLR2.2, VvGLR2.3, VvGLR2.4, and VvGLR2.5 were on chromosome 4, and VvGLR3.2, VvGLR1.1, and VvGLR3.5 were found on chromosomes 7, 11, and 18, respectively. Chromosome 12 housed VvGLR3.3 and VvGLR3.4 (Fig. 1A). Their three-dimensional domains, protein motifs, and gene architectures were identified by further investigation. Among them, VvGLR1.1, VvGLR2.1, VvGLR2.2, VvGLR2.3, VvGLR2.4, and VvGLR2.5 consisted of 5 exons, whereas the remaining VvGLR3.1, VvGLR3.2, VvGLR3.3, VvGLR3.4, and VvGLR3.5, contained 6 exons (Fig. 1B). Protein motif analysis highlighted the high conservation of GABAb and GluR domains in all VvGLRs, except for the Lig_chan domain on VvGLR2.1, VvGLR2.2, VvGLR2.3, and VvGLR3.1. MEME analysis divided the amino acid sequences of grape GLRs into 10 modules (Motif1–Motif10). With the exception of VvGLR3.1, VvGLR3.2, VvGLR3.3, and VvGLR3.5, which lacked motif1, each GLR contained nine conserved functional domains, motif2–motif10 (Fig. S1). In addition, all VvGLRs exhibited three to four transmembrane domains (M1–M4) (Figs. 1B and 1C). Based on the distribution of exons and introns, genes in both subfamilies of VvGLR, except for the third subfamily, contained 5 exons, whereas genes in the third subfamily contained 6 exons.
Figure 1: Analysis of chromosome distribution, phylogenetic relationships, conserved motifs and gene structure of VvGLRs. (A) VvGLR distribution on grape chromosomes. Chromosome sizes are indicated on the left using a scale. (B) Identification of conserved motifs in VvGLRs. Boxes with different colors represent various conserved motifs, and their relative position are displayed. A scale at the bottom is used to display sequence sizes (aa). (C) Exon-intron structures and phylogenetic tree of VvGLRs. Boxes with yellow, green, and black display un-translated regions (UTR), exons, and introns, respectively. The sequence sizes (bp) are shown on the bottom scale
3.3 VvGLRs Contained Highly Conserved Protein Structures and the Protein-Protein Interactions Were Mostly Enriched in Ion-Binding Pathways
To understand the protein structure, a VvGLR protein structure model was constructed using AtGLR as a template. Analysis revealed that despite variations in length and structure among the 11 GLR proteins, they uniformly contained conserved structural domains, GABAb and GluR, which are typical structural features of VvGLR proteins (Fig. 2).
Figure 2: Three-dimensional structures of VvGLR proteins, GLR protein interaction network in grapes, and GO enrichment analysis. (A) Three-dimensional structures of VvGLR proteins with conserved GABAb and GluR domains displayed by red and green, respectively. (B) Grape GLR proteins interaction network, where VvGLR family genes are marked with red circles. (C) Enrichment analysis of the proteins interacting with the VvGLR family. The color and size of the interactions indicate the reliability and frequency, respectively. A larger size signifies a higher interaction frequency, and a darker color signifies higher credibility
In the protein-protein interaction network, 14 proteins exhibited potential interactions with VvGLR proteins (Table S3). Functional enrichment analysis using the Gene Ontology database (https://geneontology.org/) demonstrated that these proteins were predominantly associated with biological processes, such as ion binding, response to stimuli, and catalytic activity. This result suggests that VvGLR proteins can respond to stimuli through ion binding.
3.4 VvGLRs Were Divided into Three Sub-Families
To elucidate the phylogenetic relationship between the GLR family proteins, a phylogenetic tree of the grape GLR gene family, along with 67 GLR protein sequences from two model plants (rice (20), Arabidopsis (20), and maize (16)), was constructed. Based on Arabidopsis and rice classifications, VvGLRs were categorized into three subfamilies, with 11 members distributed in Group I (1), Group II (5), and Group III (5). The phylogenetic tree illustrated that Groups I and II shared a common branch, and Group I predated Group II, aligning with the notion that Group II in Arabidopsis might have evolved from a gene copy of Group I [40]. Compared with Group I, most of the VvGLR genes were situated in Groups II and III, suggesting potential versatility (Fig. 3, Table S4).
Figure 3: Phylogenetic analysis of GLR proteins from various species. A phylogenetic tree composed of 67 GLR proteins were divided into three groups (Groups I–III). Arabidopsis, rice, grape, and corn are represented by blue circles, orange squares, purple pentagons, and purple triangles, respectively. At, Arabidopsis thaliana; Os, Oryza sativa; Vv, Vitis vinifera; Zm, Zea mays
3.5 Analysis of Collinearity in Grape VvGLR Family
Gene replication, including whole-genome, tandem, and fragment replication, is a common occurrence in plant evolution [40,41]. However, no tandem repetition events have been observed in the grape VvGLR family. Collinearity analysis of Arabidopsis AtGLR and grape VvGLR was conducted, revealing a total of six pairs of orthologous genes (VvGLR3.1 and AtGLR3.1, VvGLR3.3 and AtGLR3.4, VvGLR3.5 and AtGLR3.3, VvGLR3.3 and AtGLR3.5, VvGLR3.1 and AtGLR3.2, VvGLR2.3 and AtGLR2.4) (Fig. 4). Consistent with the earlier phylogenetic tree branches, these were identified as homologous gene pairs, suggesting potentially shared or similar functions in plant growth and development, or reaction to stressful environments.
Figure 4: The synteny analysis of VvGLRs. Gray rectangles represent Arabidopsis and grapes chromosomes, with the numbers and length labeled on every chromosome. The distribution of AtGLRs and VvGLRs on each chromosome are displayed. Synteny relationships between AtGLRs and VvGLRs are indicated with red lines. Gray lines indicate the syntenic relationships of other genes. Vv and at represent Vitis vinifera and Arabidopsis thaliana, respectively
3.6 VvGLRs Contained Plant Hormones and cis-Acting Elements in Response to Stress
Analysis of the 2000 bp promoter sequence revealed the presence of regulatory elements involved in stress and plant hormone responses. Notably, the promoter region contained ABRE, TGACG-motif, MBS/MYB/MYC, and TCA-element, indicating their roles in plant regulation and hormonal responses. Additionally, stress-related elements such as anti-inversion-related elements (TC-rich repeats), low-temperature response elements (CCGAAA), and environmental signal response elements (G-box) were identified (Fig. S2, Table S5). This suggests the potential involvement of the grape VvGLR family in the regulation of plant growth as well as in the response to various stress conditions.
3.7 Analysis of Tissue-Specific Expression Patterns of Grape VvGLR Family Genes
Analysis of gene expression data from various grape tissues revealed varying degrees of expression of the VvGLR family genes across all grape parts (Fig. 5, Table S6), with relatively high expression levels in leaves. Notably, VvGLR3.2, VvGLR3.3, VvGLR3.4, and VvGLR3.5 exhibited high expression in grape stems, vines, buds, shafts, and flesh. Transcriptome data indicated that during leaf development, VvGLR3.1 was highly expressed in young leaves, whereas in the fruit setting stage, VvGLR2.1, VvGLR1.1, VvGLR3.1, VvGLR2.2, and VvGLR3.4 displayed elevated expression. Additionally, during leaf aging, VvGLR2.3, VvGLR2.4, and VvGLR2.5 showed high expression levels.
Figure 5: Expression heat map illustrating the developmental expression profiles of VvGLRs in various graph tissues, including tendrils, leaves, seeds, stems, buds, flowers, roots, rachises, and flesh. The estimated values expressed in transcripts per million (TPM) represent the expression levels of VvGLRs. The relative expression level of each gene is represented by the colored spectrum, which goes raging from white to red
3.8 Differential Expression of VvGLRs under Cold and Salt Stress
To investigate the response and expression of the VvGLR gene to environmental stress, changes in GLR family gene expression were detected in the top two fully unfolded leaves of the grape seedlings. Real-time qRT-PCR analysis revealed the responsiveness of all VvGLRs to low temperature and salt stress (Fig. 6, Table S7). At 4°C, VvGLR2.2, VvGLR2.3 and VvGLR3.1 were upregulation, whereas VvGLR1.1, VvGLR2.4, VvGLR2.5 and VvGLR3.3 were downregulation. Notably, VvGLR2.1, VvGLR3.2, and VvGLR3.5 exhibited rapid upregulation at 4 or 6 h, followed by a return to baseline expression after 48 h. VvGLR2.3 and VvGLR3.1 displayed an expression trend of a slight initial decrease followed by an overall increase. Under −20°C treatment simulating frost damage, VvGLR2.3, VvGLR3.2, VvGLR3.3, VvGLR3.4 and VvGLR3.5 showed overall upregulation, whereas VvGLR1.1, VvGLR2.1, VvGLR2.2 and VvGLR2.5 were downregulated. Among them, VvGLR2.4 and VvGLR3.1 were initially upregulated and then recovered to baseline after 48 h. Upon treatment with 100 mmol/L NaCl stress, VvGLR2.4, VvGLR2.5, VvGLR3.1, VvGLR3.2, VvGLR3.3, VvGLR3.4 and VvGLR3.5 exhibited overall upregulation, whereas VvGLR1.1, VvGLR2.1, VvGLR2.2 and VvGLR2.3 displayed a decreasing trend. Notably, VvGLR3.1 and VvGLR3.2 demonstrated increased sensitivity to low temperature and salt stress.
Figure 6: Heatmap analysis of the relative expression of GLRs under low temperatures (4°C and −20°C) and salt stresses. Seven-week old grape seedlings were exposed to 4°C and −20°C low temperatures and 100 mmol/L NaCl for 0, 2, 4, 6, 8, 12, 24, and 48 h. Values represent the means ± standard deviation of three independent experiments (n = 3). A Student’s t-test was judged at the probability of either 5% (*p < 0.05) or 1% (**p < 0.01)
3.9 Proteins of VvGLR3.1 and VvGLR3.2 Were Located on the Cell Membrane and Cell Nucleus
According to the PSORT website, the VvGLR family of proteins is predicted to be located on the cell membrane. A subsequent in-depth investigation into the subcellular localization of the VvGLR protein revealed that the GFP empty vector exhibited fluorescence in the nucleus, cell membrane, and cytoplasm of tobacco epidermal cells. Conversely, the fusion vector containing VvGLR3.1 and VvGLR3.2 proteins emitted fluorescence exclusively on the cell membrane and in the nucleus (Fig. 7). These results indicate their functional presence on both the cell membrane and the nucleus.
Figure 7: Subcellular localization of VvGLR3.1 and VvGLR3.2. (VvGLR3.1-GFP and VvGLR3.2-GFP vectors along with plasma membrane localization protein were constructed and transiently transformed into tobacco epidermis). Scale bar = 20 μm
3.10 Overexpression of VvGLR3.1 and VvGLR3.2 in Transgenic Yeast Increased Their Sensitivity to Low Temperature and Salt Stress
Because of the sensitivity of VvGLR3.1 and VvGLR3.2 to low temperature and salt stress, overexpression vectors for VvGLR3.1 and VvGLR3.2 were constructed to verify their function in response to abiotic stress. These vectors were then introduced into INVSC1 yeast strains. Yeast overexpressing VvGLR3.1 and VvGLR3.2 exhibited increased sensitivity to low temperature and various concentrations of salt stress compared to the wild type, manifesting significantly impaired growth in comparison to the control group (Fig. 8). This result substantiates the responsiveness of VvGLR3.1 and VvGLR3.2 to low temperature and salt stress.
Figure 8: Growth of yeast overexpressing VvGLR3.1 and VvGLR3.2 under low temperature and salt stress conditions. Yeast cells harboring pYES2, pYES2-VvGLR3.1, or pYES2-VvGLR3.2, were grown in the SG-Ura synthetic dropout medium. Scale bar = 1 cm
The Arabidopsis glutamate receptor gene AtGLR, discovered in 1998 [13] and highly homologous to mammalian iGLuRs, has paved the way for identifying GLR family genes in various plants, including rice, pear, tomato, maize, and alfalfa [4–10]. Despite this, research on their stress responses in grapes remains limited.
Studies indicate that AtGLRs in Arabidopsis and GluRs in animals share four transmembrane domains (M1, M2, M3, and M4) [3,15]. By leveraging the homology and structural similarity between VvGLRs in grapes and AtGLRs and GluRs in animals, we identified 11 VvGLRs that were distributed across six grape chromosomes. Consistent with other reports on GLRs, all VvGLRs exhibited GABAb and GluR structural domains and 3–4 transmembrane domains, albeit with structural domain variations. Previous reports have indicated that the second transmembrane region M2 of iGLuRs cannot traverse the membrane [42]. This viewpoint was corroborated by transmembrane topology analysis of AtGLRs in Arabidopsis [43]. Therefore, some studies suggest that transmembrane M1 and M3, as well as non-transmembrane M2, correspond to the pore loop structure called the “hinge loop” in glutamate receptors [44], a common feature in ion channels [45,46]. Given the analogous gene structures and protein domains observed in VvGLR2.1 and VvGLR2.2, as well as VvGLR2.4 and VvGLR2.5, it is plausible that they have similar functions in grapes. Motif analysis revealed highly similar motifs across all grape GLR proteins, with no significant differences observed between members. This finding underscores the evolutionary stability of the grape GLR gene family. This study used phylogenetic analysis to examine the evolutionary history and relationships among species. The constructed phylogenetic tree categorized VvGLRs into three subgroups (Fig. 3). Notably, the majority of VvGLRs were concentrated in the second and third subgroups, comprising 90% of the total, aligning with similar patterns observed in other species [4–10].
Grape GLR exhibited 2 conserved domains and 3–4 transmembrane domains (Fig. 1B), mirroring the structural features of Arabidopsis and rice GLRs [2,12]. The motif distribution in the VvGLR varied among branches in the phylogenetic tree. Members of the same branch of the phylogenetic tree exhibited similar motif patterns. Phylogenetic and genetic structures indicated potential functional connections between them. This study highlights the correlation between the relative intron position/number and phylogenetic development. For example, VvGLR2.4 and VvGLR2.5 share a branch and similar gene structures. Phylogenetic relationships categorize VvGLRs into intron-poor and intron-rich groups (Fig. 1C), with introns often associated with variable splicing in response to environmental changes, a conserved feature across species, such as rice and Arabidopsis [47,48]. This indicated that VvGLR is conserved across different species in terms of its gene structure.
Although the distribution of genes on chromosomes varies, their regulatory relationships depend on their function [49,50]. Therefore, this study analyzed the phylogenetic relationships and regulatory elements of VvGLRs (Fig. S2 and Table S3). Furthermore, this study delved into the relationship between the phylogeny of highly homologous gene pairs with significant differences and the regulatory elements in the promoter, such as VvGLR2.1 and VvGLR2.3. Notably, this study examined the promoter region of VvGLR and identified numerous stress-responsive cis-acting elements. These elements have been identified in other species [2,12], many of which have been reported to respond to transcription factors and play regulatory roles [15,21].
Exploring the evolutionary and replication events of grape GLR genes sheds light on the intricate evolutionary processes of grapes. VvGLR was selectively located on chromosomes 3, 4, 7, 11, 12, and 18, suggesting evolutionary functions and intricate gene exchange. Gene expression patterns vary across vegetative and reproductive organs and developmental stages. Certain genes exhibit early-stage specificity, whereas others contribute to organ formation, providing pivotal insights into their biological functions [51–53]. This study meticulously analyzed the differential expression patterns of VvGLR across various grape tissues (Fig. 5), with notably elevated levels in the leaves and pulp, underscoring their roles in leaf development and pulp formation. Notably, VvGLR3.2 and VvGLR3.3 dominated in flowers, implicating their involvement in flowering regulation.
Previous studies have reported that GLRs are ubiquitously present in plant cells and participate in or mediate numerous physiological processes [3,47]. Subcellular localization prediction revealed their presence on the plasma membrane, aligning with their role as crucial ion channels that regulate Ca2+ flux [46]. Upon ligand activation, GLRs mediate Ca2+ influx across the plasma membrane to initiate downstream biological processes such as Ca2+ signal transduction during physiological and developmental events [54]. Consistent with GLRs reported in other species, VvGLRs were also predicted to be localized on the plasma membrane (Table 1). However, localization studies in Nicotiana tabacum confirmed that the proteins of VvGLR3.1 and VvGLR3.2 were located on both the cell membrane and cell nucleus. This finding, which is partially consistent with the predicted results, implies that VvGLR3.1 and VvGLR3.2 may share similar and distinct roles (Fig. 7). Maintaining cytoplasmic Ca2+ balance under normal and stressful growth conditions requires Ca2+ homeostasis, with various calcium transport proteins actively contributing, especially during non-biological stress and plant growth and development [55]. Previous studies have shown that GLR genes are found in various tissues, acting as receptor proteins pivotal in plant Ca2+ signal transduction and participating in diverse pathways throughout growth and development. Studies have indicated GLRs’ responsiveness to stresses such as drought [20], salt stress [21,47,48,56], low-temperature stress [19], wilt disease [57], antifungal infection [5], and long-distance signal transduction triggered by both biological and non-biological stressors [26,58–60]. The carboxyl-terminal region of the glutamate receptor protein GLR3.3 has been highlighted for its significant role in long-distance signal transduction induced by injury stimulation [60]. In this study, the VvGLR promoter region harbored multiple stress response elements, including those that respond to low temperatures, osmotic stress, and defense mechanisms. Real-time quantitative PCR analysis revealed the sensitivity of all VvGLRs to both low temperature and salt stress. Additionally, yeast transformation experiments proved the functionality of VvGLR3.1 and VvGLR3.2, showing their responsiveness to low temperatures and salt stress. Given the extensive gene counts and intricate structures within the grape GLR gene family, further comprehensive research is essential to uncover additional gene functions. This study serves as a foundational step for the in-depth exploration of gene functions and regulatory mechanisms.
This study identified and thoroughly analyzed 11 grape GLR genes that were categorized into three subfamilies. Expression pattern analysis revealed predominantly constitutive expression among the VvGLRs, with all members exhibiting upregulation or downregulation in response to cold and salt stress. Overexpression of VvGLR3.1 in Saccharomyces cerevisiae exhibited heightened sensitivity to both low temperatures and salt stress. Furthermore, Saccharomyces cerevisiae overexpressing VvGLR3.2 displayed a phenotype similar to that observed with VvGLR3.1. These findings offer valuable insights into the functional aspects of the VvGLRs gene, shedding light on its potential role in enhancing grape tolerance to abiotic stress.
Acknowledgement: Thanks to all the reviewers who participated in the review, as well as MJEditor (www.MJEditor.com) for providing English editing services during the writing of this manuscript.
Funding Statement: This research was funded by the Natural Science Foundation of Shandong Province of China (ZR2022MC144).
Author Contributions: The authors confirm their contribution to the paper as follows: study conception and design: Honghui Sun, Ruichao Liu, Yueting Qi, and Hongsheng Gao; data collection: Ning Jiang, Xueting Wang; analysis and interpretation of results: Honghui Sun, Ruichao Liu, and Ning Jiang; draft manuscript preparation: Hongxia Zhang, Chunyan Yu, and Xiaotong Guo. All authors reviewed the results and approved the final version of the manuscript.
Availability of Data and Materials: Data are contained within the article and supplementary material.
Ethics Approval: None.
Conflicts of Interest: The authors declare that they have no conflicts of interest to report regarding the present study.
Supplementary Materials: The supplementary material is available online at https://doi.org/10.32604/phyton.2024.049417.
References
1. Zhu S, Gouaux E. Structure and symmetry inform gating principles of ionotropic glutamate receptors. Neuropharmacology. 2017;112:11–5. [Google Scholar] [PubMed]
2. Tapken D, Hollmann M. Arabidopsis thaliana glutamate receptor ion channel function demonstrated by ion pore transplantation. J Mol Biol. 2008;383(1):36–48. [Google Scholar] [PubMed]
3. Chiu JC, Brenner ED, DeSalle R, Nitabach MN, Holmes TC, Coruzzi GM. Phylogenetic and expression analysis of the glutamate-receptor-like gene family in Arabidopsis thaliana. Mol Biol Evol. 2002;19(7):1066–82. [Google Scholar] [PubMed]
4. Singh SK, Chien CT, Chang IF. The Arabidopsis glutamate receptor-like gene GLR3.6 controls root development by repressing the Kip-related protein gene KRP4. J Exp Bot. 2016;67(6):1853–69. [Google Scholar] [PubMed]
5. Brenner ED, Martinez-Barboza N, Clark AP, Liang QS, Stevenson DW, Coruzzi GM. Arabidopsis mutants resistant to S(+)-β-methyl-α, β-diaminopropionic acid, a cycadderived glutamate receptor agonist. Plant Physiol. 2000;124(4):1615–24. [Google Scholar] [PubMed]
6. Philippe F, Verdu I, Morère-Le Paven MC, Limami AM, Planchet E. Involvement of Medicago truncatula glutamate receptor-like channels in nitric oxide production under short-term water deficit stress. Plant Physiol. 2019;236:1–6. [Google Scholar]
7. Aouini A, Matsukura C, Ezura H, Asamizu E. Characterisation of 13 glutamate receptor-like genes encoded in the tomato genome by structure, phylogeny and expression profiles. Gene. 2012;493(1):36–43. [Google Scholar] [PubMed]
8. Zhang J, Cui T, Su Y, Zang S, Zhao Z, Zhang C, et al. Genome-wide identification, characterization, and expression analysis of glutamate receptor-like gene (GLR) family in sugarcane. Plants. 2022;11(18):2440. [Google Scholar] [PubMed]
9. Kong D, Ju C, Parihar A, Kim S, Cho D, Kwak JM. Arabidopsis glutamate receptor Homolog3.5 modulates cytosolic Ca2+ level to counteract effect of abscisic acid in seed germination. Plant Physiol. 2015;167(4):1630–42. [Google Scholar] [PubMed]
10. Li J, Zhu S, Song X, Shen Y, Chen H, Yu J, et al. A rice glutamate receptor-like gene is critical for the division and survival of individual cells in the root apical meristem. Plant Cell. 2006;18(2):340–9. [Google Scholar] [PubMed]
11. Michard E, Lima PT, Borges F, Silva AC, Portes MT, Carvalho JE, et al. Glutamate receptor-like genes form Ca2+ channels in pollen tubes and are regulated by pistil D-serine. Science. 2011;332(6028):434–7. [Google Scholar] [PubMed]
12. Wudick MM, Portes MT, Michard E, Rosas-Santiago P, Lizzio MA, Nunes CO, et al. CORNICHON sorting and regulation of GLR channels underlie pollen tube Ca2+ homeostasis. Science. 2018;360(6388):533–6. [Google Scholar] [PubMed]
13. Lam HM, Chiu J, Hsieh MH, Meisel L, Oliveira IC, Shin M, et al. Glutamate-receptor genes in plants. Nature. 1998;396(6707):125–6. [Google Scholar] [PubMed]
14. Hebda A, Liszka A, Lewandowska A, Lyczakowski JJ, Gabryś H, Krzeszowiec W. Upregulation of GLRs expression by light in Arabidopsis leaves. BMC Plant Biol. 2022;22(1):197. [Google Scholar] [PubMed]
15. Kang J, Turano FJ. The putative glutamate receptor 1.1 (AtGLR1.1) functions as a regulator of carbon and nitrogen metabolism in Arabidopsis thaliana. Proc Natl Acad Sci USA. 2003;100(11):6872–7. [Google Scholar] [PubMed]
16. Kang J. The putative glutamate receptor 1.1 (AtGLR1.1) in Arabidopsis thaliana regulates abscisic acid biosynthesis and signaling to control development and water loss. Plant Cell Physiol. 2004;45(10):1380–9. [Google Scholar] [PubMed]
17. Kim SA, Junemyoung K, Seul-Ki J, Myeong-Hyeon W, Honggil N. Overexpression of the AtGluR2 gene encoding an Arabidopsis homolog of mammalian glutamate receptors impairs calcium utilization and sensitivity to ionic stress in transgenic plants. Plant Cell Physiol. 2001;42(1):74–84. [Google Scholar] [PubMed]
18. Sivaguru M, Pike S, Gassmann W, Baskin TIM. Aluminum rapidly depolymerizes cortical microtubules and depolarizes the plasma membrane: evidence that these responses are mediated by a glutamate receptor. Plant Cell Physiol. 2003;44(7):667–75. [Google Scholar] [PubMed]
19. Li H, Jiang X, Lv X, Ahammed GJ, Zhou Y. Tomato GLR3.3 and GLR3.5 mediate cold acclimation-induced chilling tolerance by regulating apoplastic H2O2 production and redox homeostasis. Plant Cell Environ. 2019;42(12):3326–39. [Google Scholar] [PubMed]
20. Lu G, Wang X, Liu J, Yu K, Gao Y, Liu H, et al. Application of T-DNA activation tagging to identify glutamate receptor-like genes that enhance drought tolerance in plants. Plant Cell. 2014;33(4):617–31. [Google Scholar]
21. Wang PH, Lee CE, Lin YS, Lee MH, Chen PY, Chang HC, et al. The glutamate receptor-like protein GLR3.7 interacts with 14-3-3ω and participates in salt stress response in Arabidopsis thaliana. Front Plant Sci. 2019;10:1169. [Google Scholar] [PubMed]
22. Achard P, Gong F, Cheminant S, Alioua M, Hedden P, Genschik P. The cold-inducible CBF1 factor-dependent signaling pathway modulates the accumulation of the growth-repressing DELLA proteins viaits effect on gibberellin metabolism. Plant Cell. 2008;20:2117–29. [Google Scholar] [PubMed]
23. Shi Y, Tian S, Hou L, Huang X, Zhang X, Guo H, et al. Ethylene signaling negatively regulates freezing tolerance by repressing expression of CBF and type-A ARR genes in Arabidopsis. Plant Cell. 2012;24:2578–95. [Google Scholar] [PubMed]
24. Hu Y, Jiang L, Wang F, Yua D. Jasmonate regulates the inducer of CBF expression-C-repeat binding factor/DRE binding factor1 cascade and freezing tolerance in Arabidopsis. Plant Cell. 2013;25(8):2907–24. [Google Scholar] [PubMed]
25. Zheng Y, Luo L, Wei J, Chen Q, Yang Y, Hu X, et al. The glutamate receptors AtGLR1.2 and AtGLR1.3 increase cold tolerance by regulating jasmonate signaling in Arabidopsis thaliana. Biochem Biophys Res Commun. 2018;506(4):895–900. [Google Scholar] [PubMed]
26. Yu B, Liu N, Tang S, Qin T, Huang J. Roles of glutamate receptor-like channels (GLRs) in plant growth and response to environmental stimuli. Plants. 2022;11(24):3450. [Google Scholar] [PubMed]
27. Kumar S, Stecher G, Li M, Knyaz C, Tamura K. MEGA X: molecular evolutionary genetics analysis across computing platforms. Mol Biol Evol. 2018;35(6):1547–9. [Google Scholar] [PubMed]
28. Chen C, Chen H, Zhang Y, Thomas HR, Frank MH, He Y, et al. TBtools: an integrative toolkit developed for interactive analyses of big biological data. Mol Plant Pathol. 2020;13(8):1194–202. [Google Scholar]
29. Higo K, Ugawa Y, Iwamoto M, Korenaga T. Plant cis-acting regulatory DNA elements (PLACE) database: 1999. Nucleic Acids Res. 1999;27(1):297–300. [Google Scholar] [PubMed]
30. Schmittgen TD. Analyzing real-time PCR data by the comparative CT method. Nat Protoc. 2008;3(6):1101–8. [Google Scholar] [PubMed]
31. González-Agüero M, García-Rojas M, di Genova A, Correa J, Maass A, Orellana A, et al. Identification of two putative reference genes from grapevine suitable for gene expression analysis in berry and related tissues derived from RNA-Seq data. BMC Genomics. 2013;14:878. [Google Scholar]
32. Martin K, Kopperud K, Chakrabarty R, Banerjee R, Brooks R, Goodin MM. Transient expression in Nicotiana benthamiana fluorescent marker lines provides enhanced definition of protein localization, movement and interactions in planta. Plant J. 2009;59(1):150–62. [Google Scholar] [PubMed]
33. Nelson BK, Cai X, Nebenführ A. A multicolored set of in vivo organelle markers for co-localization studies in Arabidopsis and other plants. Plant J. 2007;51(6):1126–36. [Google Scholar] [PubMed]
34. Yang LM, Yang Q, Liu PG, Li S. Expression of the HSP24 gene from Trichoderma harzianum in Saccharomyces cerevisiae. J Therm Biol. 2008;33(1):1–6. [Google Scholar]
35. Min X, Lin X, Ndayambaza B, Wang Y, Liu W. Coordinated mechanisms of leaves and roots in response to drought stress underlying full-length transcriptome profiling in Vicia sativa L. BMC Plant Biol. 2020;20(1):165. [Google Scholar] [PubMed]
36. Huang R, Xiao D, Wang X, Zhan J, Wang A, He L. Genome-wide identification, evolutionary and expression analyses of LEA gene family in peanut (Arachis hypogaea L.). BMC Plant Biol. 2022;22(1):155. [Google Scholar] [PubMed]
37. Zhao H, Yao P, Zhao J, Wu H, Wang S, Chen Y, et al. A Novel R2R3-MYB transcription factor FtMYB22 negatively regulates salt and drought stress through ABA-dependent pathway. Int J Mol Sci. 2022;23(23):14549. [Google Scholar] [PubMed]
38. Obata T, Kitamoto HK, Nakamura A, Fukuda A, Tanaka Y. Rice shaker potassium channel OsKAT1 confers tolerance to salinity stress on yeast and rice cells. Plant Physiol. 2007;144(4):1978–85. [Google Scholar] [PubMed]
39. Lacombe B, Becker D, Hedrich R, DeSalle R, Hollmann M, Kwak JM, et al. The identity of plant glutamate receptors. Science. 2001;292(5521):1486–7. [Google Scholar] [PubMed]
40. Singh A, Kanwar P, Yadav AK, Mishra M, Jha SK, Baranwal V, et al. Genome-wide expressional and functional analysis of calcium transport elements during abiotic stress and development in rice. FEBS J. 2014;281(3):894–915. [Google Scholar] [PubMed]
41. Cheng L, Yuan HY, Ren R, Zhao SQ, Han YP, Zhou QY, et al. Genome-wide identification, classification, and expression analysis of amino acid transporter gene family in Glycine Max. Front Plant Sci. 2016;7:515. [Google Scholar] [PubMed]
42. Bennett JA, Dingledine R. Topology profile for a glutamate receptor: three transmembrane domains and a channel-lining reentrant membrane loop. Neuron. 1995;14(2):373–84. [Google Scholar] [PubMed]
43. Chiu J, Desalle R, Lam HM, Meisel L, Coruzzi G. Molecular evolution of glutamate receptors: a primitive signaling mechanism that existed before plants and animals diverged. Mol Biol Evol. 1999;16(6):826–38. [Google Scholar] [PubMed]
44. Mayer ML. Glutamate receptors at atomic resolution. Nature. 2006;440(7083):456–62. [Google Scholar] [PubMed]
45. MacKinnon R. Pore loops: an emerging theme in ion channel structure. Neuron. 1995;14(5):889–92. [Google Scholar] [PubMed]
46. Pantoja O. Recent advances in the physiology of ion channels in plants. Annu Rev Plant Biol. 2021;72:463–95. [Google Scholar] [PubMed]
47. Chen PY, Hsu CY, Lee CE, Chang IF. Arabidopsis glutamate receptor GLR3.7 is involved in abscisic acid response. Plant Signal Behav. 2021;16(12):1997513. [Google Scholar] [PubMed]
48. Zhang H, Zhang Q, Zhai H, Li Y, Wang X, Liu Q, et al. Transcript profile analysis reveals important roles of jasmonic acid signalling pathway in the response of sweet potato to salt stress. Sci Rep. 2017;7:40819. [Google Scholar] [PubMed]
49. Filichkin SA, Hamilton M, Dharmawardhana PD, Singh SK, Sullivan C, Ben-Hur A, et al. Abiotic stresses modulate landscape of poplar transcriptome via alternative splicing, differential intron retention, and isoform ratio switching. Front Plant Sci. 2018;9:5. [Google Scholar] [PubMed]
50. Tran LS, Nakashima K, Sakuma Y, Simpson SD, Fujita Y, Maruyama K, et al. Isolation and functional analysis of Arabidopsis stress-inducible NAC transcription factors that bind to a drought-responsive cis-element in the early responsive to dehydration stress promoter. Plant Cell. 2004;16(9):2481–98. [Google Scholar] [PubMed]
51. Urao T, Yamaguchi-Shinozaki K, Urao S, Shinozaki K. An Arabidopsis MYB homolog is induced by dehydration stress and its gene product binds to the conserved MYB recognition sequence. Plant Cell. 1993;5(11):1529–39. [Google Scholar] [PubMed]
52. Han Y, Wan H, Cheng T, Wang J, Yang W, Pan H, et al. Comparative RNA-seq analysis of transcriptome dynamics during petal development in Rosa chinensis. Sci Rep. 2017;7:43382. [Google Scholar] [PubMed]
53. Monihan SM, Magness CA, Yadegari R, Smith SE, Schumaker KS. Arabidopsis CALCINEURIN B-LIKE10 functions independently of the SOS pathway during reproductive development in saline conditions. Plant Physiol. 2016;171(1):369–79. [Google Scholar] [PubMed]
54. Davenport R. Glutamate receptors in plants. Ann Bot. 2002;90(5):549–57. [Google Scholar] [PubMed]
55. Zhou L, Lan W, Chen B, Fang W, Luan S. A calcium sensor-regulated protein kinase, CALCINEURIN B-LIKE PROTEIN-INTERACTING PROTEIN KINASE19, is required for pollen tube growth and polarity. Plant Physiol. 2015;167(4):1351–60. [Google Scholar] [PubMed]
56. Cheng Y, Zhang X, Sun T, Tian Q, Zhang WH. Glutamate receptor Homolog34 is involved in regulation of seed germination under salt stress in Arabidopsis. Plant Cell Physiol. 2018;59(5):978–88. [Google Scholar] [PubMed]
57. Liu S, Zhang X, Xiao S, Ma J, Shi W, Qin T, et al. A single-nucleotide mutation in a GLUTAMATE RECEPTOR-LIKE gene confers resistance to fusarium wilt in Gossypium hirsutum. Adv Sci. 2021;8(7):2002723. [Google Scholar]
58. Shao Q, Gao Q, Lhamo D, Zhang H, Luan S. Two glutamate- and pH-regulated Ca2+ channels are required for systemic wound signaling in Arabidopsis. Sci Signal. 2020;13(640):eaba1453. [Google Scholar] [PubMed]
59. Mousavi SA, Chauvin A, Pascaud F, Kellenberger S, Farmer EE. GLUTAMATE RECEPTOR-LIKE genes mediate leaf-to-leaf wound signalling. Nature. 2013;500(7463):422–6. [Google Scholar] [PubMed]
60. Wu Q, Stolz S, Kumari A, Farmer EE. The carboxy-terminal tail of GLR3.3 is essential for wound-response electrical signaling. New Phytol. 2022;236(6):2189–201. [Google Scholar] [PubMed]
Cite This Article
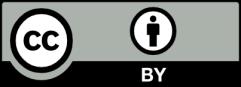
This work is licensed under a Creative Commons Attribution 4.0 International License , which permits unrestricted use, distribution, and reproduction in any medium, provided the original work is properly cited.