Open Access
REVIEW
Role of dsRNA-Based Insecticides in Agriculture: Current Scenario and Future Prospects
1 Department of Phytopharmaceuticals, School of Agricultural and Bioengineering (SoABE), Centurion University of Technology and Management, Paralakhemundi, 761211, India
2 School of Biotechnology, Centurion University of Technology and Management, Bhubaneswar, 752050, India
* Corresponding Author: Satyabrata Nanda. Email:
(This article belongs to the Special Issue: Influence of Biotic and Abiotic Stresses Signals on Plants and their Performance at Different Environments)
Phyton-International Journal of Experimental Botany 2024, 93(12), 3217-3235. https://doi.org/10.32604/phyton.2024.057956
Received 01 September 2024; Accepted 20 November 2024; Issue published 31 December 2024
Abstract
Insect pests cause severe crop damage, resulting in substantial economic losses and threats to global food security. Conventional insecticides are low-cost chemical agents that kill the target insects and some non-specific beneficial organisms. Due to their toxic and non-biodegradable nature, these conventional insecticides persist in the environment, thus causing pollution and accumulating in the food chain. The development of novel insecticidal products based on double-stranded (dsRNA)-based RNA interference (RNAi) technology is a sustainable tool to effectively control insect pests. The dsRNA-based insecticides are known for their specificity, non-toxicity, and biodegradability. The current review introduces the dsRNA-based RNAi technique as a novel tool to control crop insect pests. The review highlights the mechanism behind dsRNA uptake into insect cells. Furthermore, it discusses the commercial aspects of different dsRNA-based products available in the market, their penetration rates, and public acceptance. The review details the latest developments in the field and the regulatory landscape regarding the technology. The advantages and limitations of dsRNA-based insecticides are discussed, and future research directions to overcome the potential challenges have been briefly suggested. The dsRNA-based insecticidal products may be a better alternative to conventional insecticides, thus delineating the resistance among insects and increasing agricultural productivity.Keywords
The introduction of the Green Revolution in the 1940s was a stepping stone in agriculture that led to high agricultural productivity and made several nations self-sufficient in food production. The Rockefeller Foundation spearheaded the revolution, thus making Mexico a self-reliant nation in wheat production by 1956 [1]. The increase in agricultural productivity is quite evident as the population grows. The farm sector has resorted to injudicious farming practices to meet global food demands and achieve higher productivity rates [2]. These include higher dependence on nitrogen fertilizers and intensive cropping practices, resulting in higher pest infestations and crop damage [2]. Insecticides are harmful substances that control insect infestations in crops and crop products. The sole aim of the insecticides has been to maximize crop yields and minimize post-harvest losses [3]. Insecticides play a significant role in safeguarding crops, ensuring sufficient food supply to the growing global population of more than 9 billion people [4]. Most of the insecticides used in agriculture are of chemical origin and contain heavy metals and sulfur. Besides chemical insecticides, organic substances such as plant extracts and metabolites have also been used as an alternative. However, most farmers prefer inorganic chemical pesticides to organic ones because of their low cost and stability, the most important of which are neonicotinoids, organophosphates, and carbamates, which account for almost all insecticidal products [5].
The use of conventional insecticides has resulted in a significant number of deaths among agricultural workers, with estimates ranging from 5000 to 20,000 per year. These pesticides are primarily toxic in nature, which has contributed to the alarming number of fatalities. The ingestion of these pesticides has been linked to several adverse health effects, including renal failure, cardiovascular disease, and respiratory problems [6]. Furthermore, the excessive utilization of these insecticides has resulted in the emergence of insect and pest resistance. The development of resistance can occur via two distinct mechanisms. Exposure to insecticides may sometimes result in the mutation of specific genes, thus leading to the development of insensitivity to the chemicals among the insects. Alternatively, insects can alter the quantity and quality of their detoxifying genes and enzymes, thus creating metabolic resistance [7]. Some insecticides may persist in the environment and accumulate in different parts of the food chain, causing widespread toxicity [8]. There is an urgent need to develop new alternatives to conventional insecticides to prevent the spread of environmental toxicity and counteract the development of insect resistance.
RNA interference (RNAi) technology has emerged as a reasonably sustainable alternative to conventional insecticides, thus balancing agricultural productivity and environmental health. This process induces instability in the messenger RNA (mRNA) and disrupts transcription and translation in specific targeted cells or organisms mediated by microRNAs (miRNAs), Small interfering RNAs (siRNAs), and Argonuate (Ago) family protein complexes. RNAi mechanism leads to disruption of gene functions and a reduction in the levels of gene products, mainly proteins [9]. RNAi has contributed significantly to advancing our insights into insect biology. The importance of RNAi in modern agriculture is clearly demonstrated by the growing number of publications in this field. The top three countries with the highest number of publications in this field are the China, the USA, and India [10]. Several attractive features of RNAi make it an efficient and responsible solution for breeders to induce varietal plant improvement rather than going for more complicated and costly procedures like CRISPR/Cas9 or TALENS. RNAi can achieve a knockdown effect (by blocking or degrading mRNA transcripts) in a gene rather than complete gene knockout. Besides their unique characteristic features of high-spread mobility inside the plant system, they can also be used as a topical formulation, thus making them a highly competitive product in the market [11]. Furthermore, the demand for these products in the agricultural field is increasing due to their sustainable approach, wherein they can induce mortality in specific target insect pests or lead to the loss of a particular function in the organism without killing them [12]. The increase in agricultural productivity has also led to large-scale insect infestations, with these insects accounting for 18%–20% of global crop loss, estimated to be worth 470 billion USD [13]. RNAi represent a potential tool to the control of crop loss and sustainably improve agricultural productivity in such a situation.
Double-stranded RNA plays a significant role in RNAi. Large-scale delivery of dsRNA can specifically target genes in insects responsible for development and overall survival [14]. Particular genes accountable for energy metabolism, digestion, internal cellular activities, chitin production, hormone balance, immunity, and insecticidal resistance can be targeted. Besides, some other target genes, primarily responsible for different molecular processes like replication, transcription, translation, and fertility, can also be considered [15]. Table 1 accounts for different knockdown targets of dsRNA delivery and their impact on insect pests.
The mechanism of RNAi in insect pests can be categorized into two main phases (Fig. 1). The first phase involves the uptake of the dsRNA by the insect cells, which is then processed by the core RNAi system. It has been demonstrated that insect cells are capable of efficiently uptaking dsRNA through clathrin-mediated endocytosis [42]. Additionally, specific proteins, such as SID-1 like proteins, RNA binding proteins (RBP), or extracellular vesicles may also facilitate the uptake process by fusing the dsRNA molecules through the plasma membrane. These molecules are also thought to play a significant role during the transfer of the silencing signal from one cell to another cell of an insect pest, thereby conferring systemic silencing [2]. Three SID1-related genes were studied in Spodoptera litura by Gong et al. [43]. Analysis using qPCR found homologoues of the SID1-like genes to be involved in the transfer of dsRNA into the cell of S. litura. In another study, qPCR analysis revealed the expression of SID1 genes in various tissues of Aphis glycines, thus implicating its important role in RNAi-based gene knockdown in the species [44]. In insects like Apis mellifera, the expression levels of the SIL gene increased upon exposure to dsRNA, thus confirming its role in RNAi. Knockdown of the SIL mRNA in Leptinotarsa decemlineata resulted in reduced RNAi efficiencies [42]. However, in certain other insects, the SID1 proteins are found to have no particular role in the process of RNAi. In a study, the silencing of three orthologs of SID1 in Tribolium castaneum had no effect on RNAi efficiency [45]. Similarly, SID1 proteins or their homologs have not been found to play any role in RNAi [46]. This suggests variable roles of the protein or its homologs in different insects. In certain insects, it may facilitate the uptake of dsRNA, while in some other insects, it may not. Once inside the cell, the dsRNA is cleaved into sRNA with the aid of the enzyme DICER. Subsequently, the sRNA is then further loaded onto specific proteins of the AGO (Argonaute) family, forming the RNA-induced silencing complex (RISC). The sRNA’s guide strand facilitates the attachment of the RISC complex to the target RNA, which results in the degradation or inhibition of translation of the target mRNA and, consequently, causes post-transcriptional gene silencing. The process primarily occurs in the cytoplasm of the insect cell.; however, it can also occur by modifying chromatin within the nucleus [47,48].
Figure 1: Mechanism of RNAi in insects. The dsRNA can be taken up by insect cells by either of the three mechanisms or pathways—(A) Delivery mediated by SIL, (B) RBP, or (C) receptor-mediated endocytosis. Step 1: Uptake of dsRNA by any of the pathways. Steps 2 and 3: Cleaving of long dsRNA fragments into sRNA. Step 4: sRNA binds to proteins of the AGO family. Step 5: sRNA and AGO form an RISC complex that guides the sRNA to the target sequence. Step 6: The sRNA binds with the target gene sequence, thus degrading it to induce a silencing effect. (SIL: SID1-like proteins, RBP: RNA binding protein, AGO: Argonaute, RISC: RNA-induced silencing complex)
The RNAi process can be broadly classified into two types—Cell Autonomous RNAi (CAR) and Non-Cell Autonomous RNAi (NCAR) (Fig. 2). In CAR, the silencing effect of the dsRNA is limited to a single cell where it is applied [49]. In NCAR, the silencing can be further divided into environmental RNAi and systemic RNAi. Environmental RNAi involves the uptake of exogenously supplied dsRNA by cells from the environment. This can affect those particular cells that uptake it. Sometimes, the silencing effect can also pass from the primary cells that have taken up the dsRNA to secondary cells and tissues [50]. Environmental RNAi is primarily achieved in insects by feeding them the dsRNA or soaking them in a dsRNA solution. Systemic RNAi involves the delivery of dsRNA to a single healthy cell from where the silencing signal can further be spread to other cells and tissues [49]. In certain insects like red flour beetle, extracellular vesicles have been found to transport dsRNA from one cell to another, thus causing a silencing effect [51]. REXD-1, TBC-3, and SID-5 genes in C. elegans help promote systemic RNAi by transporting dsRNA intracellularly [52].
Figure 2: The cell-autonomous and non-cell-autonomous RNAi processes
Several methods achieve delivery of dsRNA, including microinjection, ingestion, topical application, and nanoparticle delivery. Microinjection can directly apply the dsRNA to the target insect tissues or hemolymph [53]. This ensures the safe passage of the dsRNA and prevents its degradation from the harsh gut environment of the insect. However, the microinjection technique is too laborious and requires experienced personnel. It is also impossible to use the technique in the field [54].
Ingestion is one of the simplest methods for delivering dsRNA into insects and is a feasible method for field application [55]. The midgut cells can take up the dsRNA and be transported to other tissues [56]. The delivery agent is basically a liquid formulation that can be applied to the foliar parts of the plant by soaking or mixing with insect diets to facilitate their ingestion by the target insects. The dsRNA for the purpose is mostly synthesized in vitro or by certain microbes [57]. The development of transgenic plants with the ability to continuously produce dsRNA has been instrumental in inducing RNAi in insects feeding upon the plant parts. Plant chloroplasts lack RNAi machinery, thus allowing the production and accumulation of stable dsRNA [58]. Non-transgenic delivery options are less time-consuming, and cheaper as compared to the transgenic approaches. Transgenic plant development reduces the need for pesticides but faces the problem of practical application in different plants and low public acceptance [59].
Topical applications, also referred to as spray-based formulations, penetrate the cuticle of insects to cause mortality [60]. Topical applications are quite easy to use but face the drawbacks of low silencing efficiency in insects with thicker cuticles, limiting the dsRNA’s penetration [29].
Nanoparticles have been found to act as an efficient delivery vehicle for promoting the efficient uptake and stability of the dsRNA. Chitosan nanoparticles, liposomes, and cationic dendrimers have been found to prevent the degradation of dsRNA in the environment as well as inside the insect’s gut epithelium and further help in the translocation into different cells. Nanoparticles are no doubt one of the best options for the safe and efficient delivery of dsRNA, but they are too costly and may pose certain risks [61].
3 Current Scenario in dsRNA-Based Insecticides
There have been multiple research studies to formulate different dsRNA-based insecticides specifically targeting insect pests in crops. The subsequent sub-sections deal with these insecticides’ commercial, R&D, and regulatory aspects.
3.1 Commercially Available dsRNA Insecticides
The use of dsRNA-based products in agriculture is still in its nascent stage, with very few of them commercialized to date. The first commercially available RNAi product was SmartStax Pro, a pioneering transgenic corn crop developed by Bayer Crop Science (earlier known as Monsanto). The product in question comprised a combination of two Bt proteins and a dsRNA, which targeted the snf7 gene and conferred resistance against western corn rootworms [62]. Once the Bt proteins enter the gut epithelium, they cause death by inducing gut paralysis. In contrast, the dsRNA leads to the downregulation of the snf7 gene, which plays a significant role in the protein trafficking process, ultimately resulting in death [63]. Some of the recent product developments in the field are provided in Table 2.
It is too early to compare the different dsRNA-based products that have been commercialized or are in the process of development. This is because of the slow penetration of such products into the market and the lack of sufficient customer feedback data. Silencing through the RNAi mechanism is broadly classified into two types. When the dsRNA-based formulation is delivered on plant surfaces by spraying, it is known as spray-induced gene silencing (SIGS). When the exogenous dsRNA is inserted into the plants to confer resistance against specific pests, it is called host-induced gene silencing (HIGS) [67]. Plant-induced gene silencing is known to offer long-lasting protection from insects. However, it faces several difficulties associated with the genetic transformation of plants and regulatory issues, which are not a constraint in the case of spray-based dsRNA products [68]. Here, the authors anticipate spray-based dsRNA products to have an advantage owing to their non-transgenic nature and ease of use.
There has been a slow penetration of the dsRNA-based biopesticides used to treat insect pests. Although several patents and intellectual property (IP) applications have been filed in the field of dsRNA-based insecticides, but certain other limiting factors are responsible for the slow commercialization of these products. These factors include the cost of production, the requirement for high-end infrastructure, skilled labor, and stringent regulatory norms [69].
There has been a notable increase in research activity within the field of dsRNA-based insecticides. The field of sustainable management of agricultural productivity and pest infestations has been a point of attraction for numerous researchers. In recent years, several dsRNA-based insecticidal formulations and strategies have been developed with the specific aim of targeting particular insects affecting crops. Recent research has produced a dsRNA-based insecticide (Calantha), which contains ledprona as an active ingredient against Leptinotarsa decemlineata (Colorado potato beetle) through RNAi interference. Low doses of the insecticide were found to interfere with the pupation of the larvae (fourth instar) and reduce mobility and fertility among the adults [70]. A recent study by Li et al. [71] aimed to knockdown the Rdl2 gene in Plutella xylostella, thus increasing the insect’s sensitivity towards γ-aminobutyric acid receptor targeting compounds like fipronil, pyrazoloquinazolines, and isoxazolines via RNAi mediated gene silencing.
The stability of the dsRNA within the insect gut poses a significant bottleneck in the process of RNAi. The gut environment of most insect pests is highly alkaline, thus leading to degradation of the dsRNA. Besides, several microbiota present in the gut can also lead to the degradation of the dsRNA. Lepidopterans express specific nucleases not found in other insects and can quickly degrade the dsRNA. A gene termed ‘up56’ was identified in O. furnacalis and encodes for a protein previously uncharacterized and found to be homologous in seven other lepidopteran species (while absent in other insects) with an ability to degrade dsDNA, dsRNA, and ssRNA, both in-vivo and in-vitro. Guan et al. (2018) named the gene as RNAse efficiency-related nuclease (Rease) [72]. Similarly, Hemipterans can degrade the dsRNA in their saliva and within the digestive tract [73]. The degradation of naked dsRNA molecules under several biotic and abiotic stresses invites the development of various carrier molecules for safe delivery, efficient uptake, and systematic distribution of the same in insect target cells. Several recent research studies have focused more on the efficient delivery of the dsRNA molecule to confer effective silencing of the target genes.
Protection of dsRNA is quite essential, so as to prevent its degradation and improve the RNAi efficiency. This can be achieved by several techniques, which include encapsulation of the dsRNA within liposomes (lipid bilayer-based nanoparticles) [74], nanoparticles [75], or embedded onto nano clay sheets [76]. Delivery of dsRNA could also be facilitated through root absorption in plants. The dsRNA is integrated into the crop irrigation system [77]. Insecticidal dsRNA can also be supplied via microorganisms like yeasts and E. coli [78] and other gut microbiota of the insect [79]. Table 3 provides different methods employed for efficient dsRNA delivery. The advanced delivery methods protect the dsRNA from degradation under high alkaline gut pH and help the molecule escape from the endosomes, thus protecting them. Moreover, these delivery agents also protect the dsRNA from harsh environmental conditions like fluctuations in temperature, pH, soil microbes, thereby ensuring the efficacy of the RNAi approach.
The regulatory landscape of dsRNA-based insecticides is quite complicated. The stringent regulatory frameworks are the main reason for the availability of very few dsRNA-based insecticidal products in the market. dsRNA-based crops are considered to be genetically modified and hence are strictly evaluated. Being tagged as genetically modified, these crops lose acceptance among the common masses. The European Union mentions that genetic engineering approaches are linked to specific safety concerns and must be regulated. On the other hand, the regulatory system in Canada mentions that plants that only have novel traits or foods can be sold in a marketplace [87]. The United States also follows a similar approach wherein GM crops are riskier than crops cultivated conventionally and will be subjected to different safety evaluations [87]. To date, there have been no clear guidelines on using dsRNA-based insecticides. Suppose a biotech product in the form of a plant protectant is developed and is to be registered as an insecticide. In that case, the developer must obtain an experimental usage permit from the Environmental Protection Agency (EPA) before field testing on a minimum of 10 acres of land [88]. In the US, dsRNA-based products are considered biopesticides, while in the EU, they fall under the category of chemical pesticides. Similarly, in Australia, these products are labeled under agricultural chemicals. In December 2023, the USEPA approved the registration of the dsRNA pesticide Ledprona developed by GreenLight BioSciences [69].
4 Advantages of dsRNA-Based Insecticides
Despite many uncertainties, dsRNA-based insecticides offer a number of advantages over their conventional ones (Fig. 3). Conventional insecticides are mostly inorganic in composition and can easily get transferred into the food chain as a consequence of their non-biodegradable nature and prolonger persistence in the environment. However, in this case, dsRNA-based insecticides are mostly biodegradable and non-toxic. This is due to the fact that these molecules are developed with the specific intention of targeting particular genes, thereby being specific to a particular insect pest. In comparison to their toxic inorganic counterparts, biodegradable dsRNA-based insecticides are less harmful to the environment. Inorganic insecticides poison a wide range of insects and disrupt soil ecology and fertility [89]. In contrast, dsRNA-based pesticides are natural products that can control insect infestations in crops by acting as a sustainable tool. They can be easily included under integrated pest management (IPM) strategies to confer resistance in plants or eradicate specific insect pests without harming the beneficial ones.
Figure 3: Advantages of dsRNA-based insecticides over conventional chemical insecticides
The RNAi technology via delivery of dsRNA faces several challenges and limitations. The stability of the dsRNA is questionable in the environment. Several environmental factors like temperature, photodegradation due to exposure to UV, microbial degradation, and wash-off due to rain may affect the stability of the molecules [90–93]. Off-target effects are another limitation of dsRNA-based insecticides. Although these insecticides are specifically designed to target a specific gene in a particular insect, sometimes, any other organism with the same sequence identity as that of the dsRNA will be affected [94–97]. In a study conducted by Pan et al. [98], dsRNA designed to target the V-ATPase gene in D. virgifera was found to induce RNAi in four other species that shared several 21 nucleotides continuous matches with the dsRNA sequence. One of the major bottlenecks in dsRNA-based insecticides is the development of resistance among the insects. Insects can bring about mutations to the target genes or to the genes involved in core RNAi machinery, thus leading to degradation of the dsRNA. Insects have been thought to bring about alterations in critical genes involved in RNAi mechanisms, thereby developing resistance that is quite impossible to mitigate. Recent studies found that D. v. virgifera and L. decemlineata developed resistance against Snf7 dsRNA and IAP dsRNA without any mutations to the dsRNA target site [99,100]. This confirms that the mutation of genes involved in the uptake and transport of dsRNA in both organisms is a potential reason behind the development of resistance. The production of dsRNA, which involves acquiring trained researchers, chemicals, and equipment, increases the cost. Increased production costs lead to increased market prices, a significant reason behind the non-acceptance of the product among marginal farmers. There is a fear amongst the common masses regarding the acceptability of dsRNA-based products similar to that of genetically modified ones. Moreover, certain industrial insecticide producers feel the onset of these next-generation bioinsecticides is a looming threat to their existing business.
Several research studies are now focussed on delivering the dsRNA molecules and their stability inside the insect pests and the environment [101–103,53]. Moreover, preparing the bio-insecticidal formulation is a vital part of the journey. The addition of certain surfactants generally improves the applicability of these products. Similarly, LDH-nano clays enhance the stability of up to 20 days in the foliar parts of a crop. Natural polymers like chitosan are frequently utilized as encapsulating agents, thus confirming efficient uptake of the dsRNA and protecting it from harsh alkaline pH conditions in the pest gut. The dsRNA molecules are known to be quite unstable in the soil due to several factors like temperature, other chemicals, and microbial degradation. Star cationic polymers have been developed as an efficient delivery agent, which can improve the molecule’s stability for up to 3 weeks [95]. Plant plastids have emerged as one of the most fascinating options for expressing and delivering dsRNAs in insects. Whitfield et al. successfully induced the production of long dsRNAs in the chloroplasts of potato plants [104]. The dsRNA produced from the transplastomic plants efficiently targeted the β-actin gene of the Colorado potato beetle, thus providing crop protection [105]. Plastid based RNAi approach has proven successful in tobacco plants against non-chewing herbivores like Frankliniella occidentalis thus causing high mortality [106]. The gut microbiota in insects also plays a major role in sustaining them under varied environmental conditions. They supplement insects with important nutrients, provide protection from pathogens, and help circumvent plant defense systems [107]. These microbial symbionts also help insects degrade pesticides, thus conferring high levels of resistance [108]. Targeting bacterial symbiosis in target insects has been found to efficiently cause RNAi effects. Sap-feeding insects like pea aphids demonstrated reduced growth and reproduction when two genes, namely amiD1 and LdcA1, acquired from bacterial symbiont Buchnera, were targeted [109]. The symbiotic relationship between the insect and its gut microbes has been efficiently explored for RNAi by genetically modifying the bacterial symbiont. Genetically modified yeast expressing γ-tubulin dsRNA in Drosophila suzuki have been found to reduce survivability in larvae and locomotor and reproductive activity in adults [110].
The development of dsRNA-based insecticides was initially concentrated on a few selective crops and their insect pests. However, recent studies have broadened the research horizon by experimenting with insect pests of several plants and animals, such as honey bees. The amount of dsRNA required for a successful application needs to be analyzed. As a rough estimation, 10 g of dsRNA is required per hectare of cultivable land to manage insects [111]. However, the dose may vary from insect to insect and crop to crop, thus requiring the confirmation of the doses differently in each case [112,113]. The dsRNA can also be used with certain pesticides to improve their efficiency. The synergistic effects of combining various pest control strategies can be analyzed to enhance RNAi efficiency.
The Organization for Economic Co-operation and Development states that dsRNA-based products have no ill effects on humans or the environment [114]. However, the non-target effects of RNAi make it highly important to design the dsRNA properly to avoid sequence identity. Further research needs to be carried out to improve the stability of these bioinsecticides in a way similar to conventional ones. In some cases, resistance to dsRNA can develop due to reduced uptake of dsRNA. To counter such issues, paperclip RNAs (pcRNAs) have been developed. These are the short-sized dsRNAs, and due to their closed-end structure, they can be quickly taken up through a clathrin-independent manner. These can also be applied to insects that do not respond to dsRNA-based RNAi machinery [115–118]. Developing such alternatives to dsRNA that can effectively induce silencing amongst the insects becomes essential.
The production of dsRNA-based insecticides is costly and needs collaboration between industrial, academic, and governing bodies to develop and market new sustainable pest control products. The players in the agribusiness sector will have to play a crucial part in commercializing RNAi-based insecticides.
The increasing global population is well substantiated by a corresponding increase in agricultural production. Large-scale use of fertilizers and deviation from proper agricultural practices have led to a rise in the population of insect pests in crops. These insects destroy crops and their products, thus accounting for substantial annual losses. Conventional insecticides have been successful to some extent in controlling these infestations. However, there has been an upsurge in resistance development in pests. Conventional chemical pesticides are also responsible for causing large-scale environmental pollution and negatively impacting certain beneficial organisms. The development of novel bioinsecticides has led to using dsRNA molecules as bioinsecticides to induce lethality among pests through the RNAi mechanism. The dsRNA-based bioinsecticides are characterized by their non-toxic, biodegradable nature and specificity. These modern-day bioinsecticides are being widely explored for sustainable pest management in agriculture. These molecules can induce mortality and target specific functions like mobility, reproduction, fertility, replication, and many other insect factors. The dsRNA molecule is sensitive to several biotic and abiotic stresses and prone to easy degradation. Several research and advancements in the field have led to the development of different methods for safely delivering these molecules and their efficient uptake within insect cells. Using dsRNA as an RNAi agent is also associated with several drawbacks related to its stability, efficiency, dosage, resistance, and off-target effects. Several research projects are being run to overcome these limitations. Few dsRNA products have been commercialized in the global market. The market penetration of such products and their acceptance is relatively slow due to the high costs involved, the need for a skilled workforce, and the fear of using genetically modified products. The stringent regulatory compliances of different countries and regions towards dsRNA-based insecticidal products hinder their commercialization. The paper advocates in favor of dsRNA-based insecticides as a sustainable tool for use in integrated pest management and highlights future research directions. Moreover, the authors emphasize the need for collaboration among industries, academics, and government bodies for efficient commercialization and acceptance of these products.
Acknowledgement: The authors thank Centurion University of Technology and Management for providing the necessary support. SN is grateful to SERB, the government of India, for supporting his lab research activities.
Funding Statement: The authors received no specific funding for this study.
Author Contributions: Conceptualization, Pratyush Kumar Das and Satyabrata Nanda; writing, Pratyush Kumar Das; writing—review and editing, Pratyush Kumar Das and Satyabrata Nanda; supervision, Satyabrata Nanda. All authors reviewed the results and approved the final version of the manuscript.
Availability of Data and Materials: Not applicable.
Ethics Approval: Not applicable.
Conflicts of Interest: The authors declare no conflicts of interest to report regarding the present study.
References
1. Ameen A, Raza S. Green revolution: a review. Int J Adv Sci Res. 2017;3(12):129–37. doi:10.7439/ijasr.v3i12.4410. [Google Scholar] [CrossRef]
2. Das PK, Panda G, Patra K, Jena N, Dash M. The role of polyplexes in developing a green, sustainable approach in agriculture. RSC Adv. 2022;12(53):34463–81. doi:10.1039/D2RA06541J. [Google Scholar] [PubMed] [CrossRef]
3. Araújo MF, Castanheira EM, Sousa SF. The buzz on insecticides: a review of uses, molecular structures, targets, adverse effects, and alternatives. Molecules. 2023;28(8):3641. doi:10.3390/molecules28083641. [Google Scholar] [PubMed] [CrossRef]
4. Macke J, Bozhikin I, Sarate JAR. Feeding a growing population without deforestation: agroforestry system partnerships and mechanisms. Agrofor Syst. 2021;95(4):687–706. doi:10.1007/s10457-021-00621-x. [Google Scholar] [CrossRef]
5. Mora-Gutiérrez A, Rubio C, Romero-López ÁA, Rubio-Osornio M. Neurotoxic effects of insecticides chlorpyrifos, carbaryl, imidacloprid, in different animal species. Rijeka, Croatia: IntechOpen; 2021. [Google Scholar]
6. Dwivedi SA, Sonawane VK, Pandit TR. Review on the impact of insecticides utilization in crop ecosystem: their prosperity and threats. In: Insecticides-impact and benefits of its use for humanity. England, UK: IntechOpen; 2022. doi:10.5772/intechopen.100385. [Google Scholar] [CrossRef]
7. Bass C, Nauen R. The molecular mechanisms of insecticide resistance in aphid crop pests. Insect Biochem Mol Biol. 2023;156:103937. [Google Scholar] [PubMed]
8. Kaur R, Choudhary D, Bali S, Bandral SS, Singh V, Ahmad MA, et al. Pesticides: an alarming detrimental to health and environment. Sci Total Environ. 2024;915:170113. doi:10.1016/j.scitotenv.2024.170113. [Google Scholar] [PubMed] [CrossRef]
9. Palli SR. RNAi turns 25: contributions and challenges in insect science. Front Insect Sci. 2023;3:1209478. doi:10.3389/finsc.2023.1209478. [Google Scholar] [PubMed] [CrossRef]
10. Mezzetti B, Smagghe G, Arpaia S, Christiaens O, Dietz-Pfeilstetter A, Jones H, et al. RNAi: what is its position in agriculture? J Pest Sci. 2020;93(4):1125–30. doi:10.1007/s10340-020-01238-2. [Google Scholar] [CrossRef]
11. Taning CN, Arpaia S, Christiaens O, Dietz-Pfeilstetter A, Jones H, Mezzetti B, et al. RNA-based biocontrol compounds: current status and perspectives to reach the market. Pest Manage Sci. 2020;76(3):841–5. doi:10.1002/ps.5686. [Google Scholar] [PubMed] [CrossRef]
12. Cruz C, Tayler A, Whyard S. RNA interference-mediated knockdown of male fertility genes in the Queensland fruit fly Bactrocera tryoni (Diptera: tephritidae). Insects. 2018;9(3):96. doi:10.3390/insects9030096. [Google Scholar] [PubMed] [CrossRef]
13. Sharma S, Kooner R, Arora R. Insect pests and crop losses. In: Smriti S, Rubaljot K, Ramesh A, editors. Breeding insect resistant crops for sustainable agriculture. Singapore: Springer; 2017. p. 45–66. doi:10.1007/978-981-10-6056-4_2. [Google Scholar] [CrossRef]
14. Terenius O, Papanicolaou A, Garbutt JS, Eleftherianos I, Huvenne H, Kanginakudru S, et al. RNA interference in Lepidoptera: an overview of successful and unsuccessful studies and implications for experimental design. J Insect Physiol. 2011;57(2):231–45. doi:10.1016/j.jinsphys.2010.11.006. [Google Scholar] [PubMed] [CrossRef]
15. Lu Y, Deng X, Zhu Q, Wu D, Zhong J, Wen L, et al. The dsRNA delivery, targeting and application in pest control. Agronomy. 2023;13(3):714. doi:10.3390/agronomy13030714. [Google Scholar] [CrossRef]
16. Haller S, Widmer F, Siegfried BD, Zhuo X, Romeis J. Responses of two ladybird beetle species (Coleoptera: Coccinellidae) to dietary RNAi. Pest Manage Sci. 2019;75(10):2652–62. doi:10.1002/ps.5370. [Google Scholar] [PubMed] [CrossRef]
17. Ma Z, Zhang Y, Li M, Chao Z, Du X, Yan S, et al. A first greenhouse application of bacteria-expressed and nanocarrier-delivered RNA pesticide for Myzus persicae control. J Pest Sci. 2023;96(1):181–93. doi:10.1007/s10340-022-01485-5. [Google Scholar] [CrossRef]
18. Meng J, Lei J, Davitt A, Holt JR, Huang J, Gold R, et al. Suppressing tawny crazy ant (Nylanderia fulva) by RNAi technology. Insect Sci. 2020;27(1):113–21. doi:10.1111/1744-7917.12604. [Google Scholar] [PubMed] [CrossRef]
19. Baum JA, Bogaert T, Clinton W, Heck GR, Feldmann P, Ilagan O, et al. Control of coleopteran insect pests through RNA interference. Nat Biotechnol. 2007;25(11):1322–6. doi:10.1038/nbt1359. [Google Scholar] [PubMed] [CrossRef]
20. Jin H, Abouzaid M, Lin Y, Hull JJ, Ma W. Cloning and RNAi-mediated three lethal genes that can be potentially used for Chilo suppressalis (Lepidoptera: Crambidae) management. Pestic Biochem Physiol. 2021;174:104828. doi:10.1016/j.pestbp.2021.104828. [Google Scholar] [PubMed] [CrossRef]
21. Upadhyay SK, Chandrashekar K, Thakur N, Verma PC, Borgio JF, Singh PK, et al. RNA interference for the control of whiteflies (Bemisia tabaci) by oral route. J Biosci. 2011;36:153–61. doi:10.1007/s12038-011-9009-1. [Google Scholar] [PubMed] [CrossRef]
22. Kyre BR, Rodrigues TB, Rieske LK. RNA interference and validation of reference genes for gene expression analyses using qPCR in southern pine beetle, Dendroctonus frontalis. Sci Rep. 2019;9(1):5640. doi:10.1038/s41598-019-42072-6. [Google Scholar] [PubMed] [CrossRef]
23. Bensoussan N, Dixit S, Tabara M, Letwin D, Milojevic M, Antonacci M, et al. Environmental RNA interference in two-spotted spider mite, Tetranychus urticae, reveals dsRNA processing requirements for efficient RNAi response. Sci Rep. 2020;10(1):19126. doi:10.1038/s41598-020-75682-6. [Google Scholar] [PubMed] [CrossRef]
24. Malik HJ, Raza A, Amin I, Scheffler JA, Scheffler BE, Brown JK, et al. RNAi-mediated mortality of the whitefly through transgenic expression of double-stranded RNA homologous to acetylcholinesterase and ecdysone receptor in tobacco plants. Sci Rep. 2016;6(1):38469. doi:10.1038/srep38469. [Google Scholar] [PubMed] [CrossRef]
25. Ramkumar G, Asokan R, Prasannakumar NR, Kariyanna B, Karthi S, Alwahibi MS, et al. RNA interference suppression of v-ATPase B and juvenile hormone binding protein genes through topically applied dsRNA on tomato leaves: developing biopesticides to control the South American pinworm, Tuta absoluta (Lepidoptera: Gelechiidae). Front Physiol. 2021;12:742871. doi:10.3389/fphys.2021.742871. [Google Scholar] [PubMed] [CrossRef]
26. Khan AM, Ashfaq M, Khan AA, Naseem MT, Mansoor S. Evaluation of potential RNA-interference-target genes to control cotton mealybug, Phenacoccus solenopsis (Hemiptera: Pseudococcuidae). Insect Sci. 2018;25(5):778–86. doi:10.1111/1744-7917.12455. [Google Scholar] [PubMed] [CrossRef]
27. Yan S, Qian J, Cai C, Ma Z, Li J, Yin M, et al. Spray method application of transdermal dsRNA delivery system for efficient gene silencing and pest control on soybean aphid Aphis glycines. J Pest Sci. 2020;93:449–59. doi:10.1007/s10340-019-01157-x. [Google Scholar] [CrossRef]
28. Yang S, Zou Z, Xin T, Cai S, Wang X, Zhang H, et al. Knockdown of hexokinase in Diaphorina citri Kuwayama (Hemiptera: Liviidae) by RNAi inhibits chitin synthesis and leads to abnormal phenotypes. Pest Manage Sci. 2022;78(10):4303–13. doi:10.1002/ps.7049. [Google Scholar] [PubMed] [CrossRef]
29. Chao Z, Ma Z, Zhang Y, Yan S, Shen J. RNA interference cannot be operated in lepidopteran insect? A nanocarrier breaks bottlenecks at all developmental stages of Spodoptera frugiperda. 2022. doi:10.21203/rs.3.rs-2127285/v1. [Google Scholar] [CrossRef]
30. Shang F, Ding BY, Ye C, Yang L, Chang TY, Xie J, et al. Evaluation of a cuticle protein gene as a potential RNAi target in aphids. Pest Manage Sci. 2020;76(1):134–40. doi:10.1002/ps.5599. [Google Scholar] [PubMed] [CrossRef]
31. Hu X, Richtman NM, Zhao JZ, Duncan KE, Niu X, Procyk LA, et al. Discovery of midgut genes for the RNA interference control of corn rootworm. Sci Rep. 2016;6(1):30542. doi:10.1038/srep30542. [Google Scholar] [PubMed] [CrossRef]
32. Vatanparast M, Kazzazi M, Mirzaie-Asl A, Hosseininaveh V. RNA interference-mediated knockdown of some genes involved in digestion and development of Helicoverpa armigera. Bull Entomol Res. 2017;107(6):777–90. doi:10.1017/S0007485317000293. [Google Scholar] [PubMed] [CrossRef]
33. Li M, Ma Z, Peng M, Li L, Yin M, Yan S, et al. A gene and drug co-delivery application helps to solve the short life disadvantage of RNA drug. Nano Today. 2022;43:101452. doi:10.1016/j.nantod.2022.101452. [Google Scholar] [CrossRef]
34. Dhandapani RK, Duan JJ, Palli SR. Orally delivered dsRNA induces knockdown of target genes and mortality in the Asian long-horned beetle, Anoplophora glabripennis. Arch Insect Biochem Physiol. 2020;104(4):e21679. doi:10.1002/arch.21679. [Google Scholar] [PubMed] [CrossRef]
35. Li H, Guan R, Guo H, Miao X. New insights into an RNAi approach for plant defence against piercing-sucking and stem-borer insect pests. Plant, Cell Environ. 2015;38(11):2277–85. doi:10.1111/pce.12546. [Google Scholar] [PubMed] [CrossRef]
36. Sharath Chandra G, Asokan R, Manamohan M, Krishna Kumar N. Enhancing RNAi by using concatemerized double-stranded RNA. Pest Manage Sci. 2019;75(2):506–14. doi:10.1002/ps.5149. [Google Scholar] [PubMed] [CrossRef]
37. Qu X, Wang S, Lin G, Li M, Shen J, Wang D. The synergistic effect of thiamethoxam and synapsin dsRNA targets neurotransmission to induce mortality in Aphis gossypii. Int J Mol Sci. 2022;23(16):9388. doi:10.3390/ijms23169388. [Google Scholar] [PubMed] [CrossRef]
38. McGruddy RA, Smeele ZE, Manley B, Masucci JD, Haywood J, Lester PJ. RNA interference as a next-generation control method for suppressing Varroa destructor reproduction in honey bee (Apis mellifera) hives. Pest Manage Sci. 2024;80(9):4770–8. doi:10.1002/ps.8193. [Google Scholar] [PubMed] [CrossRef]
39. Sohail S, Tariq K, Zheng W, Ali MW, Peng W, Raza MF, et al. RNAi-mediated knockdown of Tssk1 and Tektin1 genes impair male fertility in Bactrocera dorsalis. Insects. 2019;10(6):164. doi:10.3390/insects10060164. [Google Scholar] [PubMed] [CrossRef]
40. Raje KR, Peterson BF, Scharf ME. Screening of 57 candidate double-stranded RNAs for insecticidal activity against the pest termite Reticulitermes flavipes (Isoptera: rhinotermitidae). J Econ Entomol. 2018;111(6):2782–7. doi:10.1093/jee/toy294. [Google Scholar] [PubMed] [CrossRef]
41. Fu S, Liu Z, Chen J, Sun G, Jiang Y, Li M, et al. Silencing arginine kinase/integrin β1 subunit by transgenic plant expressing dsRNA inhibits the development and survival of Plutella xylostella. Pest Manage Sci. 2020;76(5):1761–71. doi:10.1002/ps.5701. [Google Scholar] [PubMed] [CrossRef]
42. Cappelle K, de Oliveira CF, Van Eynde B, Christiaens O, Smagghe G. The involvement of clathrin-mediated endocytosis and two Sid-1-like transmembrane proteins in double-stranded RNA uptake in the Colorado potato beetle midgut. Insect Mol Biol. 2016;25(3):315–23. doi:10.1111/imb.12222. [Google Scholar] [PubMed] [CrossRef]
43. Gong L, Wang Z, Wang H, Qi J, Hu M, Hu Q. Core RNAi machinery and three Sid-1 related genes in Spodoptera litura (Fabricius). Int J Agric Biol. 2015;17(5):937–44. [Google Scholar]
44. Bansal R, Michel AP. Core RNAi machinery and Sid1, a component for systemic RNAi, in the hemipteran insect, Aphis glycines. Int J Mol Sci. 2013;14(2):3786–801. doi:10.3390/ijms14023786. [Google Scholar] [PubMed] [CrossRef]
45. Tomoyasu Y, Miller SC, Tomita S, Schoppmeier M, Grossmann D, Bucher G. Exploring systemic RNA interference in insects: a genome-wide survey for RNAi genes in Tribolium. Genome Biol. 2008;9:1–22. doi:10.1186/gb-2008-9-1-r10. [Google Scholar] [PubMed] [CrossRef]
46. Wang H, Gong L, Qi J, Hu M, Zhong G, Gong L. Molecular cloning and characterization of a SID-1-like gene in Plutella xylostella. Arch Insect Biochem Physiol. 2014;87(3):164–76. doi:10.1002/arch.21189. [Google Scholar] [PubMed] [CrossRef]
47. Hanamasagar Y, Ramesha NM, Mahapatra S, Panigrahi CK, Vidhya CS, Agnihotri N, et al. Advancing RNAi for sustainable insect management: targeted solutions for eco-friendly pest control. J Exp Agric Int. 2024;46(6):740–75. doi:10.9734/jeai/2024/v46i62531. [Google Scholar] [CrossRef]
48. Ortolá B, Daròs JA. RNA interference in insects: from a natural mechanism of gene expression regulation to a biotechnological crop protection promise. Biology. 2024;13(3):137. doi:10.3390/biology13030137. [Google Scholar] [PubMed] [CrossRef]
49. Huvenne H, Smagghe G. Mechanisms of dsRNA uptake in insects and potential of RNAi for pest control: a review. J Insect Physiol. 2010;56(3):227–35. doi:10.1016/j.jinsphys.2009.10.004. [Google Scholar] [PubMed] [CrossRef]
50. Bensoussan N, Milojevic M, Bruinsma K, Dixit S, Pham S, Singh V, et al. Localized efficacy of environmental RNAi in Tetranychus urticae. Sci Rep. 2022;12(1):14791. doi:10.1038/s41598-022-19231-3. [Google Scholar] [PubMed] [CrossRef]
51. Mingels L, Wynant N, Santos D, Peeters P, Gansemans Y, Billen J, et al. Extracellular vesicles spread the RNA interference signal of Tribolium castaneum TcA cells. Insect Biochem Mol Biol. 2020;122:103377. doi:10.1016/j.ibmb.2020.103377. [Google Scholar] [PubMed] [CrossRef]
52. Yoshida K, Suehiro Y, Dejima K, Yoshina S, Mitani S. Distinct pathways for export of silencing RNA in Caenorhabditis elegans systemic RNAi. Iscience. 2023;26(10):108067. doi:10.1016/j.isci.2023.108067. [Google Scholar] [PubMed] [CrossRef]
53. Garbatti Factor B, de Moura Manoel Bento F, Figueira A. Methods for delivery of dsRNAs for agricultural pest control: the case of lepidopteran pests. In: RNAi strategies for pest management: methods and protocols. Switzerland: Springer Nature; 2022. p. 317–45. doi:10.1007/978-1-0716-1633-8_23. [Google Scholar] [CrossRef]
54. Pinheiro DH, Taylor CE, Wu K, Siegfried BD. Delivery of gene-specific dsRNA by microinjection and feeding induces RNAi response in Sri Lanka weevil, Myllocerus undecimpustulatus undatus Marshall. Pest Manage Sci. 2020;76(3):936–43. doi:10.1002/ps.5601. [Google Scholar] [PubMed] [CrossRef]
55. Fletcher SJ, Reeves PT, Hoang BT, Mitter N. A perspective on RNAi-based biopesticides. Front Plant Sci. 2020;11:51. doi:10.3389/fpls.2020.00051. [Google Scholar] [PubMed] [CrossRef]
56. Gurusamy D, Howell JL, Chereddy SC, Koo J, Palli SR. Transport of orally delivered dsRNA in southern green stink bug, Nezara viridula. Arch Insect Biochem Physiol. 2020;104(4):e21692. doi:10.1002/arch.21692. [Google Scholar] [PubMed] [CrossRef]
57. Hu J, Xia Y. Increased virulence in the locust-specific fungal pathogen Metarhizium acridum expressing dsRNAs targeting the host F1F0-ATPase subunit genes. Pest Manage Sci. 2019;75(1):180–6. doi:10.1002/ps.5085. [Google Scholar] [PubMed] [CrossRef]
58. Jin S, Singh ND, Li L, Zhang X, Daniell H. Engineered chloroplast dsRNA silences cytochrome p450 monooxygenase, V-ATPase and chitin synthase genes in the insect gut and disrupts Helicoverpa armigera larval development and pupation. Plant Biotechnol J. 2015;13(3):435–46. doi:10.1111/pbi.12355. [Google Scholar] [PubMed] [CrossRef]
59. Zhang J, Khan SA, Heckel DG, Bock R. Next-generation insect-resistant plants: rNAi-mediated crop protection. Trends Biotechnol. 2017;35(9):871–82. doi:10.1016/j.tibtech.2017.04.009. [Google Scholar] [PubMed] [CrossRef]
60. Zheng Y, Hu Y, Yan S, Zhou H, Song D, Yin M, et al. A polymer/detergent formulation improves dsRNA penetration through the body wall and RNAi-induced mortality in the soybean aphid Aphis glycines. Pest Manage Sci. 2019;75(7):1993–9. doi:10.1002/ps.5313. [Google Scholar] [PubMed] [CrossRef]
61. Yan S, Ren BY, Shen J. Nanoparticle-mediated double-stranded RNA delivery system: a promising approach for sustainable pest management. Insect Sci. 2021;28(1):21–34. doi:10.1111/1744-7917.12822. [Google Scholar] [PubMed] [CrossRef]
62. Head GP, Carroll MW, Evans SP, Rule DM, Willse AR, Clark TL, et al. Evaluation of SmartStax and SmartStax PRO maize against western corn rootworm and northern corn rootworm: efficacy and resistance management. Pest Manage Sci. 2017;73(9):1883–99. doi:10.1002/ps.4554. [Google Scholar] [PubMed] [CrossRef]
63. Kumar Gadde A, Reddy AJ, Chowdary DD, Kumar DS, Rao CS. RNA interference (RNAi) based biopesticides. In: Current development in biosciences & agriculture. India: National Press Associates; 2023. [Google Scholar]
64. Sachin GP. Gene silencing—an effective tool in insect pest management. 2019. Available from: http://14.139.185.57:8080/jspui/bitstream/123456789/13745/1/sachin%20final%20report.pdf. [Accessed 2024]. [Google Scholar]
65. Cagliari D, Dias NP, Galdeano DM, Dos Santos EÁ, Smagghe G, Zotti MJ. Management of pest insects and plant diseases by non-transformative RNAi. Front Plant Sci. 2019;10:1319. doi:10.3389/fpls.2019.01319. [Google Scholar] [PubMed] [CrossRef]
66. Fischer R. Research agreements with Dow Agrosciences. Press release, Fraunhofer-Gesellschaft zur F€orderung der angewandten Forschung e.V., Munich, Germany; 2012. Available from: https://www.fraunhofer.de/en/press/research-news/2012/january/research-agreement-with-dow-agrosciences.html. [Accessed 2024]. [Google Scholar]
67. Koch A, Höfle L, Werner BT, Imani J, Schmidt A, Jelonek L, et al. SIGS vs HIGS: a study on the efficacy of two dsRNA delivery strategies to silence Fusarium FgCYP51 genes in infected host and non-host plants. Mol Plant Pathol. 2019;20(12):1636–44. doi:10.1111/mpp.12866. [Google Scholar] [PubMed] [CrossRef]
68. Christiaens O, Dzhambazova T, Kostov K, Arpaia S, Joga MR, Urru I, et al. Literature review of baseline information on RNAi to support the environmental risk assessment of RNAi-based GM plants. EFSA Support Publ. 2018;15(5):1424E. doi:10.2903/sp.efsa.2018.EN-1424. [Google Scholar] [CrossRef]
69. Dalakouras A, Koidou V, Papadopoulou K. DsRNA-based pesticides: considerations for efficiency and risk assessment. Chemosphere. 2024;352:141530. doi:10.1016/j.chemosphere.2024.141530. [Google Scholar] [PubMed] [CrossRef]
70. Pallis S, Alyokhin A, Manley B, Rodrigues T, Barnes E, Narva K. Effects of low doses of a novel dsRNA-based biopesticide (Calantha) on the Colorado potato beetle. J Econ Entomol. 2023;116(2):456–61. doi:10.1093/jee/toad034. [Google Scholar] [PubMed] [CrossRef]
71. Li BJ, Wang KK, Yu Y, Wei JQ, Zhu J, Wang JL, et al. PxRdl2 dsRNA increased the insecticidal activities of GABAR-targeting compounds against Plutella xylostella. Pestic Biochem Physiol. 2023;195:105548. doi:10.1016/j.pestbp.2023.105548. [Google Scholar] [PubMed] [CrossRef]
72. Guan RB, Li HC, Fan YJ, Hu SR, Christiaens O, Smagghe G, et al. A nuclease specific to lepidopteran insects suppresses RNAi. J Biol Chem. 2018;293(16):6011–21. doi:10.1074/jbc.RA117.001553. [Google Scholar] [PubMed] [CrossRef]
73. Lomate PR, Bonning BC. Distinct properties of proteases and nucleases in the gut, salivary gland and saliva of southern green stink bug, Nezara viridula. Sci Rep. 2016;6(1):27587. doi:10.1038/srep27587. [Google Scholar] [PubMed] [CrossRef]
74. Castellanos NL, Smagghe G, Sharma R, Oliveira EE, Christiaens O. Liposome encapsulation and EDTA formulation of dsRNA targeting essential genes increase oral RNAi-caused mortality in the Neotropical stink bug Euschistus heros. Pest Manage Sci. 2019;75(2):537–48. doi:10.1002/ps.5167. [Google Scholar] [PubMed] [CrossRef]
75. Thairu MW, Skidmore IH, Bansal R, Nováková E, Hansen TE, Li-Byarlay H, et al. Efficacy of RNA interference knockdown using aerosolized short interfering RNAs bound to nanoparticles in three diverse aphid species. Insect Mol Biol. 2017;26(3):356–68. doi:10.1111/imb.12301. [Google Scholar] [PubMed] [CrossRef]
76. Jain RG, Fletcher SJ, Manzie N, Robinson KE, Li P, Lu E, et al. Foliar application of clay-delivered RNA interference for whitefly control. Nat Plants. 2022;8(5):535–48. doi:10.1038/s41477-022-01152-8. [Google Scholar] [PubMed] [CrossRef]
77. Ghosh SK, Hunter WB, Park AL, Gundersen-Rindal DE. Double strand RNA delivery system for plant-sap-feeding insects. PLoS One. 2017;12(2):e0171861. doi:10.1371/journal.pone.0171861. [Google Scholar] [PubMed] [CrossRef]
78. Hough J, Howard JD, Brown S, Portwood DE, Kilby PM, Dickman MJ. Strategies for the production of dsRNA biocontrols as alternatives to chemical pesticides. Front Bioeng Biotechnol. 2022;10:980592. doi:10.3389/fbioe.2022.980592. [Google Scholar] [PubMed] [CrossRef]
79. Xu L, Xu S, Sun L, Zhang Y, Luo J, Bock R, et al. Synergistic action of the gut microbiota in environmental RNA interference in a leaf beetle. Microbiome. 2021;9:1–4. doi:10.1186/s40168-021-01066-1. [Google Scholar] [PubMed] [CrossRef]
80. Yang J, Zhang Y, Zhang Z, Ren M, Wang Y, Duan Y, et al. The development of an egg-soaking method for delivering dsRNAs into spider mites. Pestic Biochem Physiol. 2024;201:105905. doi:10.1016/j.pestbp.2024.105905. [Google Scholar] [PubMed] [CrossRef]
81. Linyu W, Lianjun Z, Ning L, Xiwu G, Xiaoning L. Effect of RNAi targeting CYP6CY3 on the growth, development and insecticide susceptibility of Aphis gossypii by using nanocarrier-based transdermal dsRNA delivery system. Pestic Biochem Physiol. 2021;177:104878. doi:10.1016/j.pestbp.2021.104878. [Google Scholar] [PubMed] [CrossRef]
82. Li J, Meng S, Zhang Z, Wang Y, Li Z, Yan S, et al. Nanoparticle-mediated calmodulin dsRNA and cyantraniliprole co-delivery system: high-efficient control of two key pear pests while ensuring safety for natural enemy insects. Int J Biol Macromol. 2024;277:134478. doi:10.1016/j.ijbiomac.2024.134478. [Google Scholar] [PubMed] [CrossRef]
83. Qiao H, Zhao J, Wang X, Xiao L, Zhu-Salzman K, Lei J, et al. An oral dsRNA delivery system based on chitosan induces G protein-coupled receptor kinase 2 gene silencing for Apolygus lucorum control. Pestic Biochem Physiol. 2023;194:105481. doi:10.1016/j.pestbp.2023.105481. [Google Scholar] [PubMed] [CrossRef]
84. Zhang Y, Ke Z, Xu L, Yang Y, Chang L, Zhang J. A faster killing effect of plastid-mediated RNA interference on a leaf beetle through induced dysbiosis of the gut bacteria. Plant Commun. 2024;5(9):100974. doi:10.1016/j.xplc.2024.100974. [Google Scholar] [PubMed] [CrossRef]
85. Martinez Z, De Schutter K, Van Damme EJ, Vogel E, Wynant N, Broeck JV, et al. Accelerated delivery of dsRNA in lepidopteran midgut cells by a Galanthus nivalis lectin (GNA)-dsRNA-binding domain fusion protein. Pestic Biochem Physiol. 2021;175:104853. doi:10.1016/j.pestbp.2021.104853. [Google Scholar] [PubMed] [CrossRef]
86. Christiaens O, Tardajos MG, Martinez Reyna ZL, Dash M, Dubruel P, Smagghe G. Increased RNAi efficacy in Spodoptera exigua via the formulation of dsRNA with guanylated polymers. Front Physiol. 2018;9:316. doi:10.3389/fphys.2018.00316. [Google Scholar] [PubMed] [CrossRef]
87. European Parliament. Directive 2001/18/EC of the European Parliament and of the Council of 12 March 2001 on the deliberate release into the environment of genetically modified organisms; 2001. Available from: https://eur-lex.europa.eu/homepage.html. [Accessed 2024]. [Google Scholar]
88. Mat Jalaluddin NS, Othman RY, Harikrishna JA. Global trends in research and commercialization of exogenous and endogenous RNAi technologies for crops. Crit Rev Biotechnol. 2019;39(1):67–78. doi:10.1080/07388551.2018.1496064. [Google Scholar] [PubMed] [CrossRef]
89. Holmsgaard PN, Dealtry S, Dunon V, Heuer H, Hansen LH, Springael D, et al. Response of the bacterial community in an on-farm biopurification system, to which diverse pesticides are introduced over an agricultural season. Environ Pollut. 2017;229:854–62. doi:10.1016/j.envpol.2017.07.026. [Google Scholar] [PubMed] [CrossRef]
90. Qiao Z, Ye Y, Chang PH, Thirunarayanan D, Wigginton KR. Nucleic acid photolysis by UV254 and the impact of virus encapsidation. Environ Sci Technol. 2018;52(18):10408–15. doi:10.1021/acs.est.8b02308. [Google Scholar] [PubMed] [CrossRef]
91. Yang W, Wang B, Lei G, Chen G, Liu D. Advances in nanocarriers to improve the stability of dsRNA in the environment. Front Bioeng Biotechnol. 2022;10:974646. doi:10.3389/fbioe.2022.974646. [Google Scholar] [PubMed] [CrossRef]
92. Qiao L, Niño-Sánchez J, Hamby R, Capriotti L, Chen A, Mezzetti B, et al. Artificial nanovesicles for dsRNA delivery in spray-induced gene silencing for crop protection. Plant Biotechnol J. 2023;21(4):854–65. doi:10.1111/pbi.14001. [Google Scholar] [PubMed] [CrossRef]
93. Zhang K, Wei J, Huff Hartz KE, Lydy MJ, Moon TS, Sander M, et al. Analysis of RNA interference (RNAi) biopesticides: double-stranded RNA (dsRNA) extraction from agricultural soils and quantification by RT-qPCR. Environ Sci Technol. 2020;54(8):4893–902. doi:10.1021/acs.est.9b07781. [Google Scholar] [PubMed] [CrossRef]
94. Schowalter TD, Noriega JA, Tscharntke T. Insect effects on ecosystem services—Introduction. Basic Appl Ecol. 2018;26:1–7. doi:10.1016/j.baae.2017.09.011. [Google Scholar] [CrossRef]
95. Chen J, Peng Y, Zhang H, Wang K, Zhao C, Zhu G, et al. Off-target effects of RNAi correlate with the mismatch rate between dsRNA and non-target mRNA. RNA Biol. 2021;18(11):1747–59. doi:10.1080/15476286.2020.1868680. [Google Scholar] [PubMed] [CrossRef]
96. Chen J, Sheng CW, Peng Y, Wang K, Jiao Y, Palli SR, et al. Transcript level and sequence matching are key determinants of off-target effects in RNAi. J Agric Food Chem. 2023;72(1):577–89. doi:10.1021/acs.jafc.3c07434. [Google Scholar] [PubMed] [CrossRef]
97. Romeis J, Widmer F. Assessing the risks of topically applied dsRNA-based products to non-target arthropods. Front Plant Sci. 2020;11:679. doi:10.3389/fpls.2020.00679. [Google Scholar] [PubMed] [CrossRef]
98. Pan H, Yang X, Romeis J, Siegfried BD, Zhou X. Dietary RNAi toxicity assay exhibits differential responses to ingested dsRNAs among lady beetles. Pest Manage Sci. 2020;76(11):3606–14. doi:10.1002/ps.5894. [Google Scholar] [PubMed] [CrossRef]
99. Khajuria C, Ivashuta S, Wiggins E, Flagel L, Moar W, Pleau M, et al. Development and characterization of the first dsRNA-resistant insect population from western corn rootworm, Diabrotica virgifera virgifera LeConte. PLoS One. 2018;13(5):e0197059. doi:10.1371/journal.pone.0197059. [Google Scholar] [PubMed] [CrossRef]
100. Yoon JS, Mogilicherla K, Gurusamy D, Chen X, Chereddy SC, Palli SR. Double-stranded RNA binding protein, Staufen, is required for the initiation of RNAi in coleopteran insects. Proc Nat Acad Sci. 2018;115(33):8334–9. doi:10.1073/pnas.1809381115. [Google Scholar] [PubMed] [CrossRef]
101. Nitnavare RB, Bhattacharya J, Singh S, Kour A, Hawkesford MJ, Arora N. Next generation dsRNA-based insect control: success so far and challenges. Front Plant Sci. 2021;12:673576. doi:10.3389/fpls.2021.673576. [Google Scholar] [PubMed] [CrossRef]
102. Santos-Ortega Y, Flynt A. Double-strand RNA (dsRNA) delivery methods in insects: diaphorina citri. In: RNAi strategies for pest management: methods and protocols. New York, NY, USA: Humana; 2022. p. 253–77. doi:10.1007/978-1-0716-1633-8_19. [Google Scholar] [CrossRef]
103. Das J, Kumar R, Shah V, Sharma AK. Simple cost-effective larval injection method for dsRNA delivery to induce RNAi response in Helicoverpa armigera (Hübner). J Appl Entomol. 2023;147(4):289–98. doi:10.1111/jen.13100. [Google Scholar] [CrossRef]
104. Whitfield R, Anastasaki A, Truong NP, Cook AB, Omedes-Pujol M, Loczenski Rose V, et al. Efficient binding, protection, and self-release of dsRNA in soil by linear and star cationic polymers. ACS Macro Lett. 2018;7(8):909–15. doi:10.1021/acsmacrolett.8b00420. [Google Scholar] [PubMed] [CrossRef]
105. Zhang J, Khan SA, Hasse C, Ruf S, Heckel DG, Bock R. Full crop protection from an insect pest by expression of long double-stranded RNAs in plastids. Science. 2015;347(6225):991–4. doi:10.1126/science.1261680. [Google Scholar] [PubMed] [CrossRef]
106. Wu M, Dong Y, Zhang Q, Li S, Chang L, Loiacono FV, et al. Efficient control of western flower thrips by plastid-mediated RNA interference. Proc Nat Acad Sci. 2022;119(15):e2120081119. doi:10.1073/pnas.2120081119. [Google Scholar] [PubMed] [CrossRef]
107. Rupawate PS, Roylawar P, Khandagale K, Gawande S, Ade AB, Jaiswal DK, et al. Role of gut symbionts of insect pests: a novel target for insect-pest control. Front Microbiol. 2023;14:1146390. doi:10.3389/fmicb.2023.1146390. [Google Scholar] [PubMed] [CrossRef]
108. Akami M, Njintang NY, Gbaye OA, Andongma AA, Rashid MA, Niu CY, et al. Gut bacteria of the cowpea beetle mediate its resistance to dichlorvos and susceptibility to Lippia adoensis essential oil. Sci Rep. 2019;9(1):6435. doi:10.1038/s41598-019-42843-1. [Google Scholar] [PubMed] [CrossRef]
109. Chung SH, Jing X, Luo Y, Douglas AE. Targeting symbiosis-related insect genes by RNAi in the pea aphid-Buchnera symbiosis. Insect Biochem Mol Biol. 2018;95:55–63. doi:10.1016/j.ibmb.2018.02.004. [Google Scholar] [PubMed] [CrossRef]
110. Murphy KA, Tabuloc CA, Cervantes KR, Chiu JC. Ingestion of genetically modified yeast symbiont reduces fitness of an insect pest via RNA interference. Sci Rep. 2016;6(1):22587. doi:10.1038/srep22587. [Google Scholar] [PubMed] [CrossRef]
111. Joga MR, Zotti MJ, Smagghe G, Christiaens O. RNAi efficiency, systemic properties, and novel delivery methods for pest insect control: what we know so far. Front Physiol. 2016;7:553. doi:10.3389/fphys.2016.00553. [Google Scholar] [PubMed] [CrossRef]
112. Mehlhorn S, Hunnekuhl VS, Geibel S, Nauen R, Bucher G. Establishing RNAi for basic research and pest control and identification of the most efficient target genes for pest control: a brief guide. Front Zool. 2021;18:1–6. doi:10.1186/s12983-021-00444-7. [Google Scholar] [PubMed] [CrossRef]
113. Lucena-Leandro VS, Abreu EF, Vidal LA, Torres CR, Junqueira CI, Dantas J, et al. Current scenario of exogenously induced RNAi for lepidopteran agricultural pest control: from dsRNA design to topical application. Int J Mol Sci. 2022;23(24):15836. [Google Scholar] [PubMed]
114. OECD. Considerations for the Human Health Risk Assessment of Externally Applied dsRNA-Based Pesticides. ENV/CBC/MONO 2023; 2023. Available from: https://www.oecd.org/en/publications/considerations-for-the-human-health-risk-assessment-of-externally-applied-dsrna-based-pesticides_54852048-en.html. [Accessed 2024]. [Google Scholar]
115. Abbasi R, Heschuk D, Kim B, Whyard S. A novel paperclip double-stranded RNA structure demonstrates clathrin-independent uptake in the mosquito Aedes aegypti. Insect Biochem Mol Biol. 2020;127:103492. doi:10.1016/j.ibmb.2020.103492. [Google Scholar] [PubMed] [CrossRef]
116. Verhaeghe L. Evaluating different double-stranded RNA structures for their ability to control pest flea beetles. 2021. Available from: http://hdl.handle.net/1993/37814. [Accessed 2024]. [Google Scholar]
117. Chen A, Halilovic L, Shay JH, Koch A, Mitter N, Jin H. Improving RNA-based crop protection through nanotechnology and insights from cross-kingdom RNA trafficking. Curr Opin Plant Biol. 2023;76:102441. doi:10.1016/j.pbi.2023.102441. [Google Scholar] [PubMed] [CrossRef]
118. Laisney J, Rose VL, Watters K, Donohue KV, Unrine JM. Delivery of short hairpin RNA in the neotropical brown stink bug, Euschistus heros, using a composite nanomaterial. Pestic Biochem Physiol. 2021;177:104906. doi:10.1016/j.pestbp.2021.104906. [Google Scholar] [PubMed] [CrossRef]
Cite This Article
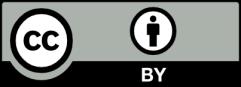
This work is licensed under a Creative Commons Attribution 4.0 International License , which permits unrestricted use, distribution, and reproduction in any medium, provided the original work is properly cited.