Open Access
REVIEW
Gasotransmitters as Key Members of the Signaling Network Regulating Stomatal Response: Interaction with Other Molecules
1 Yuriev Plant Production Institute, National Academy of Agrarian Sciences of Ukraine, Kharkiv, 61060, Ukraine
2 Department of Plant Protection, Poltava State Agrarian University, Poltava, 36003, Ukraine
3 Institute of Cell Biology and Genetic Engineering, National Academy of Sciences of Ukraine, Kyiv, 03143, Ukraine
* Corresponding Authors: Yuriy E. Kolupaev. Email: ; Tetiana O. Yastreb. Email:
(This article belongs to the Special Issue: Regulation of Phytohormone Biosynthesis and Signaling)
Phyton-International Journal of Experimental Botany 2024, 93(12), 3151-3195. https://doi.org/10.32604/phyton.2024.057922
Received 31 August 2024; Accepted 07 October 2024; Issue published 31 December 2024
Abstract
Stomatal closure, which serves to limit water loss, represents one of the most rapid and critical reactions of plants, occurring not only in response to drought but also to a range of other stressors, including salinity, extreme temperatures, heavy metals, gaseous toxicants, and pathogen infection. ABA is considered to be the main regulator of stomatal movements in plants under abiotic stress. In the last two decades, however, the list of plant hormones and other physiologically active substances that affect stomatal status has expanded considerably. It is believed that stomata are regulated by a complex multicomponent network of compounds consisting of hormones and signaling mediators. A special place among them is occupied by gasotransmitters, endogenously synthesized gaseous compounds with signaling functions. The most studied of them are nitrogen monoxide (NO), hydrogen sulfide (H2S), and carbon monoxide (CO). Carbon dioxide (CO2), not yet classified as a classical gasotransmitter, is also considered an important gaseous regulatory molecule. Information has been obtained on the ability of each of these gases to induce stomatal responses in plants. Gasotransmitters are also involved as mediators in stomatal responses induced by various plant hormones and other compounds, particularly ABA, jasmonic and salicylic acids, brassinosteroids, and polyamines. This review examines the functional interactions between gasotransmitters at the level of influencing each other’s synthesis and interactions with other mediators, especially ROS and calcium ions, in their involvement in providing stomatal responses. The latest information on proteins involved in stomatal regulation and undergoing post-translational modification under the action of gasotransmitters, including protein kinases, ion channel proteins, aquaporins, pro- and antioxidant enzymes, is also summarized. The possibilities of practical use of gasotransmitter donors as stress protective agents contributing to the normalization of plant water metabolism are considered.Keywords
It is well established that stomata are characteristic motile specialized structures of the epidermis, encased in two guard cells. The processes of stomatal opening and closing regulate gas and water exchange between the environment and plants, which is essential for their basic growth functions. The state of the stomatal apparatus has a significant impact on the efficiency of water utilization by plants, as 90% of transpiration occurs through stomata [1]. Stomatal reactions are currently regarded as a means by which plants may achieve a compromise between CO2 uptake for photosynthesis and water vapor loss [1,2]. Concurrently, stomatal closure represents a protective response of plants to a multitude of external factors, including those that induce water deficiency (e.g., drought, salinity), as well as other stressors, in particular, toxic gases and UV radiation [3,4]. The stomatal response of plants to biotic factors, primarily pathogens, which are identified by guard cell sensors according to pathogen-associated molecular patterns, is also of great significance. In recent years, it has become clear that the defense response of plants to pathogens is not only the closure of stomata, which limits their penetration but also the post-invasive opening of stomata, which deprives penetrating pathogens of a moist environment, thus limiting their reproduction [5]. This defense mechanism is extremely complex and, in particular, it involves blocking the abscisic acid (ABA) signaling pathway, which prevents stomatal opening [6].
The current understanding of stomatal regulation is evolving to encompass a complex signaling network in which the pathways triggered by various stimuli interact with each other through a number of closely related components [7]. This implies that the signaling pathways regulating stomata converge and diverge at specific points while maintaining dynamic cross-talk [8].
The size of the stomatal aperture is regulated by the turgor of guard cells, which, due to the asymmetric thickening of the cell wall, opens the stomatal aperture in the turgescence state and closes it when the turgor is lost [9]. The maintenance of high osmotic pressure within guard cells is dependent on the formation of malate through the carboxylation of phosphoenolpyruvate and the active transport of potassium and chloride ions. The closure of stomata is initiated by alterations in the activity of ion channels and the release of ions from guard cells, which can result in a selective reduction in their volume by 40% in as little as 10 min [9]. One of the earliest reactions necessary for stomatal closure is an increase in cytosolic calcium concentration in guard cells [7]. Subsequently, this triggers the activation of K+ outward-rectifying potassium (K+out) channels, which facilitate the efflux of potassium ions from guard cells, in addition to S-type anion channels—slow anion-associated channel 1 (SLAC1)—which are activated by calcium-dependent kinases [10,11]. Closure of stomatal guard cells may also involve rapidly activated R-type anion channels [12]. For example, the so-called quick anion channels, QUAC1, have also been identified in Arabidopsis [13]. Such channels are thought to be involved in the stomatal response to changes in calcium concentration, ABA action, and CO2. Nevertheless, activation of SLAC1 anion channels in guard cells [14,15] is regarded as a pivotal event that precipitates the closure of stomatal slits in response to a multitude of stimuli, predominantly ABA. Conversely, the light-induced opening of stomata is regulated by K+ inward-rectifying potassium (K+in) channels, such as KAT1, KAT2, AKT1, and AKT2, which are localized in the plasma membrane of guard cells [14].
Changes in the state of ion channels are the result of the transduction of appropriate chemical stimuli, which in turn may be formed as a result of the plant’s detection of changes in the environment. The range of endogenous chemical compounds for which the ability to change the state of stomata has been established is constantly expanding. In addition to the main hormone responsible for rapid responses of stomatal closure during drought, ABA [3], the role of ethylene [8], jasmonates, salicylic acid, brassinosteroids [13,16,17], gamma-aminobutyric acid [18], melatonin [19–21], polyamines, and other compounds with hormonal effects have been studied in recent decades [13,20]. The effect of these compounds on the stomatal state depends on their concentration, duration of change, and balance of endogenous levels of the compounds, which can act either synergistically or antagonistically [16].
In addition to plant hormones and stress metabolites with hormonal activity, key signaling mediators are involved in the regulation of the stomatal state. Reactive oxygen species (ROS), calcium ions, and nitric oxide are considered the most important components of the signaling network for stomatal closure [8]. Nitric oxide (NO) belongs to gasotransmitters (GTs).
The term “gasotransmitters” is used to describe a class of small gaseous molecules that are synthesized by living organisms and perform signaling functions. These compounds are synthesized by cellular enzymatic systems. In contrast to hormones, their effects are not receptor-dependent; rather, they act on multiple intracellular targets of protein nature [22]. Along with NO, carbon monoxide (CO) and hydrogen sulfide (H2S) are considered the main GTs in plant cells [22,23]. To date, it has been demonstrated that all three GTs can modulate the state of stomata [24]. Furthermore, insights into the molecular mechanisms of GTs involvement in the regulation of stomatal state are emerging. One such mechanism involves post-translational modifications (PTM) of target proteins [25–27]. The list of gaseous compounds that influence stomatal status is not limited to the three “classical” GTs mentioned above (NO, H2S, and CO). Carbon dioxide (CO2) plays an important role in the stomatal regulation [28]. This gaseous compound is not yet considered a “classical” GT. However, some researchers have recently classified CO2 as a GT because it is converted to HCO3– by the enzyme carboanhydrase, which may act as an intracellular signaling mediator [29]. The data on possible changes in the expression of many dozen genes in plants in response to changes in CO2 concentration indicate the existence of different and largely unknown mechanisms of its influence on the state of stomata [28].
Models proposed in recent years for the regulation of stomata by GTs, although elucidating some pathways of their action, do not reveal the mechanisms of their involvement in the extremely complex network regulating the stomatal apparatus [8,30].
The objective of this review was to summarize recent findings on the mechanisms of GT participation in the regulation of stomata. This is to be done in the context of their functional interaction with other key signaling mediators (ROS and calcium ions), as well as their involvement in the realization of the stomatal effects of plant hormones and some stress metabolites. Analysis of such information may contribute to the search for new strategies to improve the efficiency of water use by plants and their resistance to many abiotic stress factors.
2 NO and Plant Stomatal Responses
2.1 A Brief Summary of Synthesis, Metabolism, and Signaling Functions of NO
Nitric oxide is a relatively short-lived gaseous uncharged molecule that exists in radical form because of an unpaired electron in the outer orbital [31]. For NO to perform signaling functions, the functioning of mechanisms to maintain its homeostasis in plant cells is essential. The balance between the processes of NO synthesis and its conversion into other less reactive nitrogen species determines the endogenous levels of this signaling molecule in plants [32].
Current evidence indicates the existence of two pathways of NO synthesis in plants: oxidative and reductive. The former is also referred to as arginine-dependent, whereas the latter is nitrate-dependent [33].
The mechanisms of NO synthesis via the oxidative pathway remain a matter of debate, as homologs of animal NO synthase have only been identified in green algae (specifically, Ostreococcus tauri and Ostreococcus lucimarinus) and not in higher plants [34]. It is postulated that the NO synthase (NOS) gene was lost during plant evolution [35].
Consequently, it is probable that higher plants possess proteins that are distinct from NOS but are nevertheless capable of generating NO through the use of L-arginine as a substrate. This reaction, similar to that catalyzed by animal NOS, can occur in the presence of NADPH, FMN, FAD, calmodulin, and calcium ions [36,37]. It is hypothesized that such NO-synthesizing catalytic systems exist in organelles, such as chloroplasts, mitochondria, and peroxisomes [38–40] (Table 1). In addition to L-arginine, polyamines are also regarded as potential substrates for NO formation within the oxidative pathway (Table 1). It has been postulated that these transformations can be catalyzed by di- and polyamine oxidases, which are predominantly localized in the cell walls [39]. Recently, another mechanism of NO synthesis in plants has been discovered involving the conversion of indole-3-acetaldoxime, a precursor of indole-3-acetic acid [41]. The authors showed that the formation of NO from this product in Arabidopsis thaliana can be catalyzed by peroxidase. Whether the discovery of such a mechanism of NO generation will resolve the long-standing discussion on the oxidative mechanisms of NO synthesis in plants remains to be elucidated.
The reductive pathway utilizes nitrate or nitrite as substrates in reactions catalyzed by nitrate reductase, plasma membrane-associated nitrite-NO reductase, and xanthine oxidoreductase localized in peroxisomes [38]. The nitrate reductase-dependent NO synthesis pathway is believed to be the primary metabolic route (Table 1) [34]. It plays a role in plant adaptation to stressors, including dehydration [35]. In addition to the enzymatic pathway, NO can also be synthesized via non-enzymatic mechanisms. At acidic pH values, nitrite can be chemically reduced to NO [32]. It has been demonstrated that ascorbic acid reduces nitrite to form NO and dehydroascorbic acid in chloroplasts and the apoplast (Table 1).
The intracellular content of free NO is regulated not only by its synthesis but also by its metabolism. One of the principal transformations of NO is its interaction with the reduced form of glutathione (GSH) to form S-nitrosoglutathione (GSNO) [32]. This compound serves as a primary reservoir for NO and is an efficient donor for protein S-nitrosylation. In plant cells, GSNO levels are regulated by S-nitrosoglutathione reductase 1 (GSNOR1) [42]. This enzyme is primarily localized in the cytosol, where it catalyzes the conversion of GSNO to the oxidized form of glutathione (GSSG) and ammonia (NH3) using NADH as a reducing equivalent. Therefore, the potential amount of NO that can be utilized for post-translational modification of proteins from GSNO as a reservoir is inversely dependent on the activity of GSNOR1. In this regard, this enzyme can be considered both a negative regulator of NO signaling and one of the factors preventing the development of nitrosative stress. In other words, GSNOR1 is currently regarded as a means of precisely regulating NO signaling pathways in plants [43]. Modulation of the gene expression and activity of S-nitrosoglutathione reductase 1 in plants under various stresses has been demonstrated. For example, gene expression and activity of this enzyme are decreased in Arabidopsis and sunflower plants in response to mechanical stress and jasmonic acid action [44]. In contrast, Arabidopsis HOT5 mutants defective in the GSNOR1 gene were found to be more sensitive to the effects of heat stress than wild-type plants and were characterized by excessive accumulation of ROS and RNS [45]. Under salt stress, variations in GSNOR1 activity have been observed in different plant species, depending on the severity and duration of exposure [43].
In addition to regulating the amount of NO associated with GSNOR1 activity, its binding to non-symbiotic forms of hemoglobin and conversion into nitrate is considered an effective means of neutralizing excess nitric oxide [23]. The role of hemoglobins in regulating the amount of NO in plant cells has also been confirmed using molecular genetic methods. For example, transgenic barley plants overexpressing the barley hemoglobin class 1 gene HvHb1 exhibited significantly reduced NO content compared to wild-type plants [46].
In recent years, NO conversion to nitroxyl (HNO/NO–) has been considered another mechanism for the modification of its intracellular levels. Such RNS can be formed as a result of the chemical interaction of NO with another GT, H2S [47]. More recently, using a specially designed fluorescent probe based on the fluorophore dicyanoisophorone, HNO was successfully visualized for the first time in plants, onion epidermal cells, and tobacco seedlings [48]. At the same time, the contribution of NO elimination pathways to the regulation of signaling processes under plant stress has been much less studied than the role of NO synthesis. However, these processes may be important for stomatal regulation [1] (see below).
The main molecular mechanism of NO involvement in the signaling network of plant cells is currently considered to be the PTM of proteins. Three types of protein PTMs induced by NO are distinguished: S-nitrosation, nitration of tyrosine residues, and nitrosylation of metal-containing proteins [23,37].
S-nitrosation is a reversible process that alters the functional activity of target proteins. This process is independent of enzymatic involvement [49]. However, this process is highly specific and dependent not only on the proximity between NO and the target protein but also on the amino acid sequence and conformation of the protein [50]. In Arabidopsis plants, a site-specific nitrosoproteomic approach identified 926 proteins as potential targets of S-nitrosylation [51]. A more recent nitrosoproteomic study of tomato plants under salt stress and GSNO action revealed 1054 targets for such PTM [52].
Another mechanism of protein PTM that is influenced by NO is the nitration of tyrosine residues. This process is primarily mediated by peroxynitrite (ONOO–) and nitrogen dioxide radical (NO2) [53]. Nitration of tyrosine residues within proteins has traditionally been regarded as an irreversible process and a marker of nitrosative stress [54]. Nevertheless, the existence of tyrosine denitrase, capable of reducing 3-nitrotyrosine in mammalian cells, indicates the potential involvement of tyrosine nitration in NO-mediated signaling pathways within these cells [55].
Another NO-induced PTM is the nitrosylation of metal-containing proteins. This occurs when NO interacts with transition metal ions, which are part of metalloproteins, leading to the formation of metal-nitrosyl complexes. Such processes induce reversible conformational alterations in proteins, thereby modifying their structures and/or functional activities [49].
PTM of target proteins also appears to be the main mechanism of functional interaction between NO and other components of the signaling network, particularly ROS, hydrogen sulfide, and calcium ions [36,56,57]. The ability of NO to enhance Ca2+ entry into the cytosol of plant cells was demonstrated in several studies two decades ago by treating cells with NO donors [58]. At present, two principal pathways through which NO influences Ca2+ channel activity are recognized: a cGMP-independent pathway and a cGMP-dependent pathway [59]. It is postulated that in the cGMP-independent mechanism, the effect of NO on Са2+ homeostasis is achieved through S-nitrosylation of Са2+ channels and transporters. However, there is currently no data available regarding the specific membrane proteins of plant cells that are modified by NO and are involved in the entry of calcium into the cytosol, despite the existence of such information for animal cells over an extended period [59].
Evidence supporting the hypothesis that a mechanism of cGMP-mediated activation of calcium entry by NO exists in higher plants is still insufficient. Three mechanisms are considered plausible [59]: (a) direct activation of cyclic nucleotide-regulated channels by cGMP, resulting in calcium entry into the cytosol; (b) cGMP-mediated activation of protein kinases that phosphorylate calcium channel proteins; and (c) cGMP-mediated enhancement of cyclic ADP-ribose (cADPR) synthesis, which also activates protein kinases that modify calcium channel proteins. Despite the lack of direct molecular genetic evidence for a cGMP-dependent signaling pathway in higher plants [60], it has been established that this pathway links NO and Са2+ signaling in green algae [61]. A flavin monooxygenase (At1g62580) that binds NO and can generate cGMP from GTP in vitro in a NO-dependent manner has been identified in Arabidopsis using a domain-specific (H-NOX) search motif [62]. In conclusion, despite the lack of clarity surrounding the mechanisms by which NO facilitates calcium entry into the cytosol, a substantial body of experimental evidence has emerged indicating that the majority of the physiological effects of NO as a signaling mediator in higher plant cells are realized with the involvement of cytosolic calcium [37,59,63]. In addition, the effects of NO on stomata are mediated by cGMP and calcium (see below).
The signaling effects of NO are also realized in a close relationship with ROS. It has long been established that NO and superoxide anion radicals can interact to form peroxynitrite (ONOO−), which is recognized as a toxic compound and an agent of nitrosative stress, as well as a participant in signal transduction [60]. Nevertheless, it is reasonable to conclude that the direct interaction between NO and ROS represents only one example of interconnection between these mediators. The PTM of proteins under the influence of both NO and ROS may underlie diverse modulations of cell signaling processes. On one hand, NO and ROS may compete for targets of such modifications. In contrast, enzymes that generate or neutralize ROS or NO may be targets for PTMs [64]. For example, it has long been demonstrated that NO engages in the S-nitrosation reaction of NADPH oxidase at Cys890, leading to its inhibition of ROS generation [65]. Additionally, the peroxisomal ROS-generating enzyme glycolate oxidase can be inhibited by S-nitrosation [66]. Such processes exemplify the antagonistic relationship between NO and ROS as signaling mediators.
A variety of antioxidant enzymes can undergo post-translational modifications under the action of NO, with the outcome of these PTMs potentially leading to either activation or inhibition of protein activity [64]. This allowed us to consider such PTMs as a mechanism of fine-tuning in redox signaling. For example, cytosolic ascorbate peroxidase in peas and Arabidopsis can undergo S-nitrosation at the ascorbate-binding site (Cys32), which enhances enzyme activity [67]. Simultaneously, NO can inhibit ascorbate peroxidase and catalase activity through tyrosine nitration [67]. Conversely, catalase is susceptible to both nitration and S-nitrosation, resulting in a reduction in enzyme activity [68]. It is conceivable that such processes are instrumental in augmenting the local intracellular accumulation of ROS, which may serve as signaling molecules [64]. Additionally, evidence suggests that ROS may facilitate the enhancement of NO synthesis. For example, activation of NO formation with the participation of nitrate reductase under the influence of hydrogen peroxide in Arabidopsis has been reported [69], although the mechanisms of such activation have not yet been specifically investigated.
In addition to affecting enzymes that generate and neutralize ROS, NO can alter redox homeostasis by interacting with reduced glutathione to form GSNO [60]. Another potential mechanism through which NO affects cellular redox homeostasis is the modulation of the activity of enzymes involved in maintaining the NADPH pool [70]. It is commonly accepted that in a specific cellular context, ROS and NO compete for particular Cys residues and are influenced by the cellular redox environment [37].
In addition to ROS, hydrogen sulfide has a very close functional interaction with NO. For example, the treatment of maize seedlings with a NO donor, which induced the development of heat tolerance, was accompanied by increased expression of the gene encoding L-cysteine desulfhydrase (DES1), increased enzyme activity, and activation of hydrogen sulfide synthesis [71]. In contrast, hydrogen sulfide has been shown to cause persulfidation of the cysteine residues of nitrate reductase and decrease its activity [72]. However, the results of a study in which the effect of the hydrogen sulfide donor NaHS on salt tolerance of alfalfa plants and expression of antioxidant enzyme genes was eliminated by the NO scavenger PTIO (2-phenyl-4,4,5,5-tetramethylimidazoline-1-oxyl 3-oxide) [73] did not agree with the data on the negative regulation of NO homeostasis by hydrogen sulfide. Additionally, the data revealed that the induction of heat tolerance in wheat seedlings was associated with positive regulation of NO synthesis by exogenous hydrogen sulfide. NaHS treatment was accompanied by a transient increase in nitrate reductase activity and NO content in roots [74]. Conversely, the nitrate reductase inhibitor sodium tungstate was observed to negate the increase in NO content in roots induced by the hydrogen sulfide donor. Notably, the mechanisms of hydrogen sulfide influence on NO synthesis enzymes have not been studied in the aforementioned studies. It is therefore possible that the described phenomena are not directly related to the effect of H2S on these enzymes but are instead mediated by other components of the signaling network.
2.2 Main Mechanisms of NO Influence on Stomatal State
The capacity of guard cells to produce NO has been established for over two decades [75]. An increase in the NO content in guard cells has been observed in response to a number of agents that induce stomatal closure. These include plant hormones such as ABA, methyl jasmonate, salicylic acid, ethylene, and strigolactone as well as other signaling mediators such as calcium ions and hydrogen peroxide, as well as pathogen elicitors and environmental factors such as UV-B and CO2 (for reviews, see [8,76]). Concurrently, numerous studies have examined the influence of diverse NO donors, including sodium nitroprusside (SNP), sodium nitrite, and L-arginine, on stomatal functioning [75,77]. Nevertheless, it remains unclear whether NO can act as an independent effector, causing changes in the state of stomata, or whether it can only mediate the effects of other compounds in intact plants. The molecular mechanisms by which NO regulates the ion channels that determine the state of stomata are not fully understood. Finally, the interaction of NO with other members of the signaling network involved in regulating stomata has not yet been fully elucidated.
It is hypothesized that the primary mechanism underlying NO-mediated regulation of stomatal movement is the alteration of calcium homeostasis within guard cells [8]. As noted above, it is believed that in plant cells, as in animal cells, cGMP, which is formed from GTP by soluble cytoplasmic guanylate cyclase (GC), serves as a signaling mediator between NO and calcium [78]. This mechanism plays a crucial role in stomatal closure (Fig. 1). As previously stated, the flavin monooxygenase At1g62580 (AtNOGC1), which can generate cGMP in a NO-dependent manner, has been identified in Arabidopsis [62]. In the nogc1 mutant, the knockout of NO-dependent guanylate cyclase resulted in the failure of ABA and the NO donor 1-hydroxy-2-oxo-3-(3-aminopropyl)-3-isopropyl-1-triazene (NOC5) to induce stomatal closure [79].
Figure 1: Possible mechanisms of nitric oxide influence on the state of ion channels of stomatal guard cells. Nitric oxide (NO) binds to and activates guanylate cyclase 1 (NOGC1), which promotes the accumulation of cyclic guanosine monophosphate (cGMP). cGMP can interact with reactive nitrogen species (RNS) to form 8-nitro-cGMP, which can activate ADP-ribosyl cyclase (ADPRC). This leads to the accumulation of cyclic ADP-ribose (cADPR), which opens tonoplast calcium channels. An increase in cytosolic calcium concentration (Ca2+cyt) activates calcium-dependent protein kinases (CDPKs), which, through protein phosphorylation, activate K+out channels as well as slow-type anion channel 1 (SLAC1) and inhibit K+in channels. NO also causes activation of phospholipase C (PLC) and phospholipase D (PLD), resulting in the accumulation of inositol 1,4,5-trisphosphate (IP3) and phosphatidic acid (PA). IP3 opens intracellular calcium channels and promotes Ca2+cyt. PA inhibits K+ influx channels and also causes activation of NADPH oxidase (RBOH, Respiratory Burst Oxidase Homolog). In addition, RBOH is activated by increasing Ca2+cyt concentration, which increases the generation of reactive oxygen species (ROS). ROS are involved in RNS formation and SLAC1 activation. Blunt-ended arrows indicate inhibition. The dotted arrows indicate hypothetical pathways without direct experimental confirmation. Other abbreviations: GTP—guanosine triphosphate
One potential mechanism by which cGMP affects calcium homeostasis may be its conversion to 8-nitroguanosine 3’,5’-cyclic monophosphate (8-nitro-cGMP), which occurs as a consequence of direct interaction with NO [79]. It was demonstrated that under illuminated conditions, exogenous 8-nitro-cGMP induced stomatal closure in a dose-dependent manner. In contrast, 8-bromo-GMP, a cell-penetrating analog of cGMP, did not induce stomatal closure. The authors posit that 8-nitro-cGMP, rather than cGMP, plays a pivotal role in NO-signaling-dependent stomatal closure [79]. Concurrently, the signaling effects of 8-nitro-cGMP are manifested through calcium-dependent processes (Fig. 1). In particular, the cell-penetrating calcium chelator has been shown to eliminate stomatal closure induced by 8-nitro-cGMP or NO donors [79]. Notably, ABA and NO did not induce stomatal closure in mutant plants lacking NOGC1. This indicates that NO signaling by AtNOGC1 is a prerequisite for GC activation and, concurrently, for the formation of 8-nitro-cGMP [26].
The accumulation of cGMP and 8-nitro-cGMP results in an increase in the activity of ADP-ribosyl cyclase and a corresponding increase in the content of another signaling mediator in cells, namely cADP-ribose (Fig. 1). The action of cADP-ribose is associated with stimulation of intracellular calcium channels, which are primarily located in the tonoplast, and increased calcium entry into the cytosol. This leads to the activation of calcium-dependent protein kinases [8]. Antagonists of cADP synthesis (nicotinamide and 8-bromo-cADP-ribose) inhibit NO- and 8-nitro-cGMP-dependent closure of stomata in Arabidopsis [79]. These results demonstrate the participation of ADP-ribosyl cyclase in 8-nitro-cGMP signaling. The experimental data indicate that protein kinases activated by calcium entering the cytosol through ADP-ribose-stimulated channels play a role in the activation of SLAC1, which provides anion efflux from the guard cells of the stomata [11]. Additionally, cADP-ribose affects ion fluxes in guard cells by inactivating K+in channels, a process that involves the participation of corresponding protein kinases [37].
As an additional mechanism to enhance calcium entry into the cytosol of guard cells by NO action, the activation of phospholipases C and D (PLC and PLD) has been proposed as a means of accumulating phosphatidic acid and inositol 1,4,5-trisphosphate (IP3), which can also open intracellular calcium channels [8]. Moreover, phosphatidic acid, which is formed by phospholipid hydrolysis, is known to inhibit K+in channels but activates channels for anion efflux from guard cells (Fig. 1) [37]. The details of the mechanisms by which NO influences guard cell ion channel state associated with changes in phospholipase activity remain largely unexplored. It is also likely that phosphatidic acid formed as a result of phospholipase activation, in turn, activates the catalytic subunit of NADPH oxidase RBOH (Fig. 1). This may enhance the generation of ROS, which is also involved in stomatal closure, particularly through conversion to RNS.
In addition to the calcium-mediated pathways of NO influence on the stomatal state described above, some pathways are not directly related to calcium entry into the cytosol. These mechanisms are based on the PTM of proteins induced by NO. In recent years, a complex of mechanisms for the regulation of the stomatal state associated with the activity of aquaporins has been actively studied. At the whole-plant level, the upward transduction of water stress signals involves the aquaporin-mediated transformation of chemical signals into hydraulic signals. The role of NO in the regulation of aquaporins under water stress has only been studied fragmentarily. However, based on bioinformatics methods and computational prediction of oxidative post-translational modifications (oxiPTM) of cysteine residues in plant aquaporins, a concept was formulated according to which the activity of aquaporins can be regulated by PTM, in particular S-nitrosation [80]. This effect is thought to contribute to the entry of hydrogen peroxide through aquaporins from the apoplast into the cytoplasm of guard cells [81]. This in turn causes anion efflux from guard cells due to oxidative modification of anion channels. Experimental data have also been obtained in rice and kiwifruit plants, indicating the ability of NO donors to stimulate the expression of plasma membrane aquaporin genes [82]. This is thought to provide additional pathways of NO influence on stomatal status associated with the modulation of aquaporin quantity and activity [80].
Studies are currently underway to identify guard cell proteins that undergo S-nitrosation. For example, iodoTMTRAQ quantitative proteomics technology has been used to analyze protein modifications associated with S-nitrosation in Arabidopsis thaliana guard cells in response to flg22, an elicitor-active peptide derived from bacterial flagellin [83]. A total of 41 S-nitrosated peptides were identified at different stages of the stomatal response, corresponding to 35 proteins with a wide range of functions, including energy metabolism, transport, stress response, photosynthesis, and intercellular communication. To our knowledge, no such proteomic studies have been performed on the effect of exogenous NO directly or abiotic stresses that enhance NO generation on the state of stomatal proteins.
2.3 Involvement of NO in the Realization of ABA Effects
Several mechanisms by which NO influences the stomatal state have been described previously. These mechanisms may be components in the realization of stomatal effects of known plant hormones, as well as a number of stress metabolites capable of modulating the stomatal state.
ABA is involved in plant responses to various abiotic and biotic factors and is therefore considered the most important and versatile plant stress hormone [84]. Under drought, salinity, or cold stress conditions, ABA accumulation leads to stomatal closure to conserve water and the concomitant activation of genes that promote osmotic adaptation [85].
The development of processes after the perception of an increase in ABA concentration, culminating in a final response (closure of stomata), is mediated by a common signaling pathway that engages the participation of protein kinases, secondary messengers, ion channels, ion outflow, and loss of turgor in guard cells [86,87].
OST1 is thought to be a convergence point for signaling different stressors [86]. At the same time, there is experimental evidence that stomatal closure can occur without OST1 [88]. However, the “canonical” ABA signaling pathway is currently regarded as a pathway that commences with its interaction with the receptor PYR/PYL/RCAR, which blocks PP2C phosphatase activity (Fig. 2). Consequently, the protein kinase OST1, which remains phosphorylated, activates several components, most notably NADPH oxidase and anion channels (QUAC1 and SLAC1), which causes anion efflux from guard cells. Consequently, ABA-dependent activation of RBOHF occurs via phosphorylation at Ser13 and Ser174 by OST1 [89], resulting in augmented ROS generation in guard cells. Molecular genetic studies have indicated that two isoforms of the catalytic subunits of NADPH oxidase, RBOHD, and RBOHF, are involved in the stomatal effects of ABA [64].
Figure 2: Involvement of nitric oxide and reactive oxygen species in realization of stomatal effects of abscisic acid. Abscisic acid (ABA) binding to PYR/PYL/RCAR receptor causes inactivation of PP2C phosphatase. As a result, OST1 (Open Stomata 1) protein kinase, in its phosphorylated state, activates NADPH oxidase (RBOH) as well as slow-type anion channel 1 (SLAC1) and quick-activating anion channel 1 (QUAC1). Activation of RBOH results in generation of reactive oxygen species (ROS), which leads to increased nitric oxide (NO) synthesis under influence of nitrate reductase (NR) and mediated by mitogen-activated protein kinase (MPK 3/6). NO triggers a cascade of mediators, including cyclic guanosine monophosphate (cGMP) and cyclic ADP-ribose (cADPR), which in turn lead to an increase in cytosolic calcium concentration (Ca2+cyt) and activation of calcium-dependent protein kinases (CDPKs). These, through protein phosphorylation, activate SLAC1 and K+out channels but inhibit K+in channels. NO also causes phosphatidic acid (PA) accumulation via increased phospholipase C (PLC) and phospholipase D (PLD) activity. This activates RBOH and ROS generation. Interrupted arrows indicate the presence of unspecified mediators. Dotted arrows indicate hypothetical pathways without direct experimental confirmation
Key secondary messengers (ROS, cytosolic Ca2+, and NO) have multiple effects on each other during regulation of stomatal movement (Fig. 2). The increase in ROS levels through activation of MAP kinase 3/6 causes increased NO generation, which is mainly associated with increased NR activity [90]. Furthermore, NO increases cytosolic Ca2+ levels through mechanisms involving cGMP and cADP-ribose (as described in detail above). An increase in cytosolic Ca2+ can activate calcium-dependent protein kinases (CDPKs), which facilitate further Ca2+ influx from the external environment into guard cells. Consequently, Ca2+-activated protein kinases stimulate SLAC1 while inhibiting potassium entry into cells [86,91]. The involvement of NO in ABA-induced stomatal closure was substantiated by data obtained from an Arabidopsis nia1nia2 mutant with impaired NO production via a nitrate reductase-dependent pathway. In such plants, the impact of ABA on stomatal closure is markedly diminished, although not entirely abolished [90]. The involvement of NO-induced activation of PLC and PLD and phosphatidic acid in the inactivation of K+in channels has also been suggested [92] (Fig. 2).
Thus, NO is considered a calcium- and ROS-related mediator in the “canonical” ABA signaling pathway. However, data have also been obtained indicating a possible negative effect of NO on ABA signaling that induces stomatal closure. In particular, ABA receptor proteins PYR/PYL/RCAR have been shown to be nitrated on tyrosine residues and S-nitrosated on cysteine residues in Arabidopsis [93]. Tyrosine nitration, but not cysteine S-nitrosation, reduces receptor activity in vitro. In addition, ABA receptors with nitrated tyrosine residues undergo proteasomal degradation. There is also evidence for a possible negative regulation of the protein kinase OST1 by NO (Fig. 2) [94]. It has been shown that NO can S-nitrosate OST1 in vitro and in vivo at Cys137, adjacent to the catalytic site of the kinase, thereby eliminating its phosphorylation activity [95]. Another mechanism for limiting the stomatal effects of ABA by NO may be the S-nitrosation of RBOH, which leads to reduced generation of ROS, which acts as one of the mediators of ABA signaling (Fig. 2) [65].
Using Vicia faba leaves, van Meeteren et al. [96] compared the effects of ABA and gaseous NO, or its donor, on the state of stomata in epidermal strips and whole leaves. Epidermal strips showed that stomatal closure was induced by ABA but not by NO, which casts doubt on the role of NO in ABA-mediated stomatal closure. At the same time, a study of the response of intact leaves to NO showed the opposite sign of stomatal reaction in a dose-dependent manner: the stomatal aperture widened when exposed to low concentrations of NO and narrowed when exposed to high concentrations. The authors also found different stomatal closure kinetics when the leaves were treated with ABA and NO. Based on these results, the authors suggested that NO may not be a key factor in ABA-induced rapid stomatal closure, but that it fine-tunes the stomatal aperture through different pathways [96]. It should be noted that in long-term experiments, treatment of plants with NO donors can mitigate the stress-induced effects of stomatal closure. This seems to result not from the direct participation of NO in signaling processes in guard cells, but from its indirect effects on a multitude of stress-protective systems. For example, in [97], an increase in stomatal aperture in wheat plants subjected to salt stress was observed following the application of the NO donor SNP. The authors ascribed this effect to an increase in GSH content, which plays a role in cellular redox homeostasis and may, therefore, influence the condition of the stomata.
Thus, it is now thought that NO can both stimulate and inhibit ABA-induced stomatal closure, thereby participating in the fine regulation of hormone stimulus intensity [98].
2.4 Involvement of NO in Realization of Stomatal Effects of Jasmonic Acid
One of the key groups of “stress” plant hormones is jasmonic acid (JA) and its ester methyl jasmonate (MeJA). To date, a large body of experimental evidence has accumulated indicating the action of JA as a factor in the regulation of plant growth and development [99,100], as a signal that activates the expression of plant defense genes upon pathogen infection [101], and as a hormone involved in the adaptive responses of plants to the action of various abiotic stressors, such as extreme temperatures, drought, salinity, heavy metal ions, and others [101–103]. Jasmonate-induced stomatal closure is considered an important defense response to prevent pathogen entry through the leaves [104]. However, this effect of JA and its derivatives is also considered a mechanism to enhance plant resistance to abiotic stresses, mainly drought [105].
In contrast to ABA, the regulatory mechanisms of JA-mediated stomatal movements have been the subject of much less research. It is believed that the effect of JA on the state of stomata may be associated with the induction of ABA synthesis under its influence and/or with the formation of cross-signaling between MeJA and ABA [106].
Arabidopsis mutants have revealed several signaling components involved in the cross-talk between the two hormones. For example, inhibition of the protein phosphatase PP2C, the next negative component of ABA signal transduction after the ABA receptor, was shown to be necessary to induce stomatal closure not only under the action of ABA but also in response to MeJA (Fig. 3). Thus, ABA-insensitive PP2C mutants abi2-1 were unable to close their stomata in response to MeJA treatment [107]. In a more recent study, Ye et al. [108] demonstrated that endogenous ABA plays a role in MeJA-induced stomatal closure in A. thaliana. It is also upstream of mediators, such as ROS and NO, in the signaling pathway of exogenous MeJA-induced stomatal closure. This is evidenced by the absence of an increase in the content of ROS and NO in guard cells in response to the action of MeJA in the ABA-deficient aba2-2 mutant. In addition, the ABA synthesis inhibitor fluridone abolishes the effects of increased ROS and NO levels in response to MeJA treatment in wild-type plants [108].
Figure 3: Involvement of nitric oxide and reactive oxygen species in the realization of the stomatal effects of jasmonic acid. Jasmonic acid (JA) or methyl jasmonate (MeJA) are thought to activate ABA synthesis and inhibit PP2C. As a result, they activate ABA-specific signaling pathways that include protein phosphorylation involving OST1 and ROS synthesis involving RBOH. The accumulation of ROS leads to an increase in NO synthesis under the influence of NO synthase-like enzyme (NOS/NOA) as well as NR. The increase of NO content in cells causes changes in calcium homeostasis with the participation of mechanisms characteristic for the action of ABA or exogenous NO donors. Other abbreviations: COI—CORONATINE-INSENSITIVE 1 (JA receptor). Other designations as in Fig. 2
Other experimental data also indicated the necessity of the involvement of an additional ABA signal in the realization of the stomatal effects of MeJA. Thus, it has been demonstrated that Arabidopsis protein kinase OST1 mutants (ost1-2) do not exhibit stomatal closure, both in response to ABA treatment and MeJA action [106]. Additionally, the guard cells of the leaves from these plants did not demonstrate enhanced generation of hydrogen peroxide and NO when exposed to both ABA and MeJA. However, this study did not observe an increase in OST1 activity in wild-type plants in response to MeJA, despite the detection of such an effect in response to ABA. Furthermore, a quadruple mutant of ABA receptor genes (pyr1 pyl1 pyl2 pyl4) was demonstrated to possess the ability to close stomata in response to MeJA. Furthermore, in this mutant, as in the wild-type plants, the levels of ROS and NO increased in guard cells in response to MeJA treatment. The authors put forth the hypothesis that there are parallel MeJA signaling pathways involving different sets of ABA receptors. In addition, the authors suggested that a key component of jasmonate signaling, the transcription factor JIN1/MYC2, may also be involved in guard cell signaling as a node of functional interaction between ABA and MeJA, and a subset of ABA receptors may play a role in this process [106]. This notion is supported by evidence that ABA treatment of the leaf epidermis has no effect on stomatal aperture in Arabidopsis jin1 mutants [109]. Nevertheless, NO, as a member of the signaling network regulating stomata, appears to act downstream of JIN1/MYC2. Thus, the effect of stomatal closure in Arabidopsis jin1 mutants in response to NO donors and sodium nitrite was nearly indistinguishable from that observed in wild-type Col-0 plants [110].
However, experimental data suggest a link between NO signaling and JIN1/MYC2 function. The involvement of MYC family genes in NO signaling has long been suggested based on bioinformatics analyses [111]. In our study, we showed that Arabidopsis jin1 mutants, in contrast to wild-type plants, had showed almost no effect on increasing the activities of the antioxidant enzymes superoxide dismutase, catalase, and peroxidase, sugar content, and mitigating oxidative damage in response to treatment with the NO donor SNP under salt stress [112]. This fact does not provide direct evidence for the involvement of JIN1/MYC2 in NO signaling. The dependence of the relatively prolonged physiological effects of exogenous NO on the activity of JIN1/MYC2 may, for example, be related to the positive effect of NO on the level of JA in signal transduction of which the JIN1/MYC2 protein is the key protein [103]. The mutual enhancement of JA and NO synthesis in plants according to the principle of a self-amplifying loop has been described in literature [113]. Unfortunately, studies on the functional interaction between JA and NO using molecular genetic approaches are still lacking.
Nevertheless, the role of NO as a mediator in the effect of JA on stomata can be considered proven. An increase in the amount of NO in guard cells in different plant species under the action of JA or MeJA was first observed some time ago [107,114]. The increase in NO content is thought to occur predominantly via the involvement of nitrate reductase [115]. However, a study by Liu et al. [114] performed on Vicia faba leaf epidermal cells demonstrated that JA-induced stomatal closure was abolished by an animal NO synthase inhibitor (L-NAME). Conversely, inhibition of nitrate reductase did not eliminate the effects of JA [114]. Our studies revealed that the MeJA-induced reduction in the aperture of the stomatal slit and the number of open stomata in Arabidopsis plants was nearly abolished by pretreatment of the epidermal cells with the NO scavenger PTIO and partially by inhibitors of animal NO synthase (L-NAME) and nitrate reductase (sodium tungstate) [77]. Therefore, the results demonstrate the significance of NO formation through two principal pathways in mediating the effects of MeJA on stomatal state (Fig. 3). It is plausible that the contribution of different NO synthesis pathways in guard cells may vary depending on the species and age of the plant, as well as the specific experimental conditions employed.
2.5 Involvement of NO in Stomatal Reactions Induced by Salicylic Acid
Salicylic acid (SA) is a plant hormone that plays a role in regulating a number of physiological processes, including seed germination, flowering, transpiration, thermogenesis, response to pathogen infection, and adaptation to various abiotic stressors [116–119]. The influence of SA results in activation of the antioxidant system, synthesis of stress proteins, and accumulation of multifunctional low-molecular-weight protective compounds. Additionally, SA can induce stomatal closure in plants [119–122]. Exposure to exogenous SA or alterations in its endogenous content result in changes in the content of numerous signaling mediators and other plant hormones within cells [120]. Conversely, activation of the signaling network is frequently accompanied by an increase in SA content [123]. Concurrently, SA functionally interacts with other stress-related plant hormones, primarily ABA and JA [122,124].
Together with ABA and JA, SA is involved in the regulation of stomatal states. An association between an increase in endogenous SA levels in plants and a decrease in stomatal aperture size has been previously reported [125]. Impaired stomatal closure has also been observed in salicylate-deficient plant genotypes [126]. Simultaneously, siz1 mutants that accumulate increased amounts of SA show stomatal closure without any treatment [127].
In recent years, evidence has shown that the stomatal effects of SA are realized with the involvement of components of the ethylene signaling pathway [128]. SA induced 1-aminocyclopropane-1-carboxylic acid synthase (ACS) gene expression, resulting in ethylene production (Fig. 4). Following the interaction of ethylene with its own receptor, ETR1 (ETHYLENE RESPONSE 1), the protein kinase CTR1 (CONSTITUTIVE TRIPLE RESPONSE 1) is inactivated, which promotes NADPH oxidase activation and ROS formation. ROS can also be generated by extracellular peroxidases. There is evidence that downstream ROS signaling in guard cells may also involve ethylene signaling proteins (ETHYLENE INSENSITIVE, EIN), leading to NO production catalyzed by the nitrate reductases NIA1 and NIA2 [128]. At the same time, there is evidence of partial suppression of SA-induced NO accumulation in guard cells by an animal NO synthase inhibitor (L-NAME) and its abolition of stomatal closure in Arabidopsis [129,130].
Figure 4: Involvement of nitric oxide and reactive oxygen species in realization of stomatal effects of salicylic acid. Salicylic acid (SA) activates 1-aminocyclopropane-1-carboxylic acid synthase (ACS) gene expression and subsequent ethylene (ET) synthesis, which in turn enhances ROS synthesis involving RBOH and extracellular peroxidase (PO). This leads to activation of NO synthesis involving NR and NOA1 (NOS/NOA), and changes in calcium homeostasis. This activates Ca2+-dependent protein kinases CPK3 and CPK6 that phosphorylate ion channels K+out and SLAC1. Other abbreviations: CTR1—CONSTITUTIVE TRIPLE RESPONSE 1 protein kinase; EIN—ETHYLENE INSENSITIVE (ethylene signalling proteins); MPK9 and MPR12—MAP kinases; NPR—NONEXPRESSOR OF PATHOGENESIS-RELATED GENES 1 (SA receptor). Other designations as in Fig. 2
However, as stated above, the presence of a gene identical to animal NO synthase in higher plants has not been demonstrated. Therefore, it has been proposed to rename the previously discovered AtNOS1 gene in Arabidopsis as AtNOA1 [130]. AtNOA1 protein is a GTPase, yet it does modulate NO accumulation in plants. It was demonstrated that noa1 mutants exhibited minimal effects on NO synthesis and stomatal closure induced by exogenous SA in wild-type plants [130].
As previously stated, one of the primary pathways through which NO exerts its influence on the condition of stomata is linked to cGMP- and cADP-ribose-dependent alterations in calcium homeostasis and calcium-dependent protein kinases that regulate the activity of ion channels in guard cells (Fig. 4). In other words, changes in calcium homeostasis in the signaling pathway are thought to be downstream of NO. However, Hao et al. [129] employed an inhibitor method to demonstrate that cGMP and cADP-ribose play pivotal roles in both SA-induced stomatal closure in A. thaliana plants and NO accumulation in guard cells. Additionally, NO accumulation in guard cells was prevented by treatment of the epidermis with calcium-dependent protein kinase and calmodulin inhibitors [129]. It is probable that calcium can act both before and after NO in SA signaling pathways.
Recent studies have implicated the Ca2+-dependent protein kinases CPK3 and CPK6 in SA-induced stomatal closure. SA activation of SLAC1 required for stomatal closure was shown to be impaired in protoplasts of cpk3-2 and cpk6-1 guard cells [131]. Concurrently, the calcium-independent protein kinase OST1 is not a critical component of the SA signaling pathway in guard cells. Thus, the activation of SLAC1 required for stomatal closure under SA was not impaired in the protoplasts of ost1-3 mutants [131]. The authors proposed a model for the integration of SA and ABA signaling in Arabidopsis guard cells in which SA triggers extracellular peroxidase-dependent ROS signaling that activates calcium-dependent protein kinases, but not OST1 protein kinase. Calcium-dependent protein kinases then phosphorylate Ser59 and Ser120 in SLAC1 and activate the channel. On the other hand, the activity of both Ca2+-dependent protein kinases and OST1 is required for SLAC1 activation by ABA [131]. At the same time, the peroxidase-dependent generation of ROS was shown to be involved in the activation of calcium entry into the cytosol and the subsequent increase in the activity of calcium-dependent protein kinases.
Agurla et al. [132] corroborated the role of ROS generated by peroxidase in stomatal closure using methyl salicylate, but not by NADPH oxidase. Meanwhile, the significance of NO generated by the reductive pathway in the manifestation of the stomatal response induced by methyl salicylate was demonstrated using nia1 and nia2 mutants, and the nitrate reductase inhibitor tungstate. Furthermore, evidence has been obtained indicating that MAP kinases (MPK9 and MPR12) serve as the link between ROS and NO in the salicylate-induced signaling pathway (Fig. 4) [132].
In general, summarizing the results cited above, it can be assumed that NO is downstream of ROS but upstream of calcium in the signal transduction pathways induced by ABA, JA, and SA. At the same time, the effect of NO on ion channels, leading to a decrease in guard cell turgor, can be realized both by calcium-dependent protein kinases and calcium-independently, probably by post-translational modification of the corresponding proteins.
2.6 Involvement of NO in the Regulation of Stomata by Some Stress Metabolites
One of the important groups of multifunctional stress metabolites is polyamines, the levels of which can increase several times or even by orders of magnitude in plants under stress factors [133]. Polyamines are involved in the regulation of many plant functions, particularly cell division and differentiation, rhizogenesis, flowering, growth, and fruit development and ripening [134,135]. Their functions are believed to be similar to those of classical plant hormones; however, polyamines are present in plant cells and exhibit physiological activity at concentrations much higher than hormone concentrations. Polyamines perform both direct protective functions and those caused by activation of the signaling network. The direct stress-protective effects of polyamines are associated with their ability to stabilize the structures of proteins, nucleic acids, and membrane complexes. This is due to their cationic state under physiological conditions [136]. The stress-protective effects of polyamines may be mediated by the formation of signaling compounds upon oxidation by Cu amine oxidase (CuAO) [137] or FAD-binding polyamine oxidases [138]. Such reactions directly result in the formation of hydrogen peroxide, the ROS with the highest signaling potential [139]. It is also believed that NO may be formed during the oxidation of polyamines (Fig. 3). However, the mechanism underlying NO synthesis in plant cells under the influence of amine oxidases remains unknown. It has been suggested that CuAO does not directly generate NO but may indirectly affect NO levels through the formation of hydrogen peroxide [139].
Stomatal closure is one of the effects of exogenous polyamines on plant cells. The significance of hydrogen peroxide formation and NO synthesis in the mechanism of polyamine-mediated stomatal closure has been elucidated using inhibitor assays and molecular genetic techniques in Arabidopsis epidermal guard cells [140]. The authors discovered that putrescine, spermidine, and spermine induced stomatal closure, which was accompanied by an increase in NO and ROS levels in guard cells. The NO scavenger cPTIO and an inhibitor of animal NOS-like enzymes L-NAME were found to abolish the stomatal closure induced by polyamines.
However, this effect was not eliminated by the nitrate reductase inhibitor tungstate. Additionally, polyamines effectively closed stomata in a mutant defective in the nitrate reductase gene (nia1/2). This suggests a role for oxidatively generated NO in stomatal closure (Fig. 5). Furthermore, a notable reduction in polyamine-induced hydrogen peroxide accumulation and stomatal closure was observed following the pretreatment of cells with the NADPH-oxidase inhibitor diphenyleneiodonium and the di- and polyamine oxidase inhibitors 2-bromoethylamine and 1,12-diaminododecane. The involvement of these ROS-generating enzymes in the manifestation of the stomatal effects of polyamines was also substantiated through the utilization of mutants of the corresponding genes. In these plants, the capacity to close stomata in response to polyamine treatment was compromised. Furthermore, mutants of the diamine oxidase (cuao1-1) and polyamine oxidase (pao-4) genes had almost no effect on the increase in NO levels in guard cells under the action of polyamines. The authors suggested that ROS are upstream of NO in the signaling chain that causes stomatal closure (Fig. 5) [140].
Figure 5: Involvement of nitric oxide in stomatal closure under the influence of polyamines and melatonin. An increase in cellular polyamines causes activation of Cu-dependent diamine oxidase (CuAO) or polyamine oxidase (PAO) and NADPH oxidase (RBOH), leading to increased generation of reactive oxygen species (ROS) and nitric oxide (NO). NO activation causes an increase in cytosolic calcium (Ca2+cyt) concentration with the involvement of phospholipase C (PLC), inositol 1,4,5-trisphosphate (IP3), and possibly other mechanisms. An increase in Ca2+cyt leads to activation of calcium-dependent protein kinases (CDPKs) and modulation of ion channels, resulting in decreased guard cell turgor and stomatal closure. Under the influence of melatonin, RBOH activation occurs, leading to increased ROS generation and subsequent increased NO synthesis with the participation of nitrate reductase (NR). As a result, calcium homeostasis is altered and changes in the state of ion channels occur, leading to stomatal closure. Red and green arrows indicate effects related to polyamines and melatonin, respectively. Interrupted arrows indicate the presence of unspecified mediators. Dotted arrows indicate hypothetical pathways without direct experimental confirmation. Other abbreviations: PMTR1—melatonin receptor; NOS/NOA—nitric oxide synthase-like enzyme
The mechanisms by which NO functions as a signaling mediator in the action of polyamines on the ion homeostasis of guard cells remain poorly understood. This process appears to be calcium dependent. The nonspecific calcium channel blocker lanthanum chloride almost completely abolished the effects of putrescine and spermine on epidermal stomatal closure in pea leaves [141]. The effects of putrescine, but not of spermine, were partially inhibited in the presence of the extracellular calcium chelator EGTA. In addition, the effects of putrescine and spermine on stomatal aperture size were completely eliminated when the epidermis was treated with the PLC inhibitor neomycin [141]. Neomycin prevents the opening of intracellular calcium channels by inhibiting inositol 1,4,5-trisphosphate (IP3) synthesis. Thus, these results suggest that calcium entry into the cytosol of guard cells from intracellular compartments rather than from the apoplast may play a greater role in the effects of polyamines.
Adamipour et al. [142], using intact Rosa canina L. plants, showed a connection between drought-induced accumulation of putrescine, increased CuAO activity, and hydrogen peroxide and NO levels as signaling mediators, which is essential for the reduction of stomatal aperture.
Another multifunctional stress metabolite whose role in plants has been actively investigated in recent years is melatonin (N-acetyl-5-methoxytryptamine), the major plant indolamine [143]. An increasing body of experimental evidence indicates its involvement in signaling and hormonal network functions in plants [144]. New insights are emerging that melatonin effects may manifest at the level of gene expression, protein status, and by involvement in the signal transduction system of other hormones and mediators of non-hormonal nature [145]. An increase in endogenous melatonin content in response to stress factors has been demonstrated in plants of diverse species [146]. Additionally, there is a substantial body of evidence indicating that exogenous melatonin can enhance plant resistance to a range of abiotic stressors [146–148].
Melatonin appears to be one of several physiologically active substances that regulate the stomata. Recently, it was found in Arabidopsis that melatonin induces stomatal closure through phytomelatonin receptor 1 (PMTR1)-mediated activation of ROS and NO production [19]. The authors showed that exogenous melatonin-induced stomatal closure and NO accumulation in guard cells were abolished by the NO scavenger cPTIO. It was also found that lines mutant for two forms of nitrate reductase (nia1nia2) and an enzyme associated with NO synthesis (noa1) were incapable of melatonin-induced stomatal closure, indicating that melatonin-mediated stomatal closure is dependent on NO. Furthermore, melatonin-induced NO synthesis was shown to be impaired in rbohC and rbohD/F (NADPH oxidase homologs) mutant plants. Moreover, ROS levels in nia1nia2 and noa1 were not significantly different from those in the wild-type plants, indicating that NO is a downstream component of melatonin-induced ROS generation. It was also found that exogenous melatonin did not induce NO or ROS synthesis in guard cells of pmtr1 mutant lines. This suggests that NO levels increase after increased ROS generation during PMTR1-mediated stomatal closure in Arabidopsis [19].
3 Hydrogen Sulfide in Stomatal Regulation
3.1 Synthesis, Metabolism of Hydrogen Sulfide and Its Involvement in Cell Signaling
Hydrogen sulfide is a weak acid that dissociates in aqueous media to form hydrosulfide (HS–) and sulfide (S2–) anions. The term “hydrogen sulfide” is typically used to refer to both molecular and ionic forms [149]. However, only the molecular form, H2S, can be considered a GT in the strict sense, as it is the only form capable of diffusing freely across cell membranes, whereas HS– can move between different subcellular organelles or cells through specific ion channels [150].
Along with NO, hydrogen sulfide is considered to be one of the most important GTs in plants. At present, hydrogen sulfide is considered to be involved in the regulation of many functions of the plant organism, in particular, growth processes, fruit maturation and ripening, and adaptation to the action of stressors of diverse nature [149,151,152]. Data obtained by bioinformatics methods indicate that hydrogen sulfide as a mediator is involved in the signal transduction of almost all known plant hormones [153]. This allowed us to consider it a key component of the regulatory systems of plant cells and organisms in general.
In plants, hydrogen sulfide synthesis occurs predominantly in chloroplasts and, to a lesser extent, in other subcellular spaces such as the cytoplasm and mitochondria [154].
The generation of H2S by plants is a result of the action of several enzymes. The primary enzyme involved in the synthesis of hydrogen sulfide is believed to be L-cysteine desulfhydrase (DES) (Table 2). Evidence suggests that it is localized in the cytoplasm, plastids, and mitochondria [155]. Furthermore, the high concentration of cysteine in the cytosol provides an environment conducive to the rapid synthesis of hydrogen sulfide from cysteine via the action of DES, accompanied by the generation of by-products, such as ammonia and pyruvate [154]. The DES1 gene in Arabidopsis is expressed in various tissues at all stages of plant development, and its role has been extensively investigated in the context of stomatal regulation, including ABA-mediated regulation [149] (see below). Hydrogen sulfide can also be formed from D-cysteine through the action of D-cysteine desulfhydrase, the localization of which has been confirmed in the cytoplasm [156] (Table 2).
Hydrogen sulfide can be synthesized by sulfite reduction involving sulfite reductase in the chloroplasts when the photosynthetic sulfate assimilation pathway is active [154]. The formation of hydrogen sulfide is contingent upon the availability of reduced ferredoxin, which serves as the electron source for sulfur reduction (Table 2). In the presence of an alkaline reaction within the chloroplast stroma, plants exhibit spontaneous transport of hydrosulfide anion into the cytosol [151].
Additionally, a non-enzymatic pathway for hydrogen sulfide synthesis in plants through the reaction of thiols or thiol-containing compounds with other molecules has been documented [151]. For example, glutathione reduces inorganic polysulfides or hydrolyzed inorganic sulfide salts (Na2S) by reacting with water to form H2S [149].
In the mitochondria, hydrogen sulfide formation is possible during cyanide detoxification. This process occurs with the help of β-cyanoalanine synthase, an enzyme that catalyses the conversion of cyanide to β-cyanoalanine using cysteine (Table 2) [157]. Hydrogen sulfide metabolism also occurs in the mitochondria. The generated H2S is used by the mitochondrial isoform O-acetylserine(thiol)lyase to synthesize cysteine [154,158]. Thus, the hydrogen sulfide and cysteine cycle are maintained in the mitochondria.
Concurrently, the production of hydrogen sulfide by cells results in its release into the atmosphere. Additionally, hydrogen sulfide can be incorporated into a range of organic compounds, including cysteine and GSH [154,158].
Protein persulfidation as a type of oxidative PTM is now considered the main redox mechanism for the realization of H2S signaling effects [149]. Hydrogen sulfide reacts with oxidized rather than reduced thiols (R-SOH) or with protein nitrosothiols (R-SNO) to form protein persulfides:
protein-SH+ROS→protein-SOH (1)
protein-SOH+H2S→protein-SSH (2)
Persulfidation is recognized not only as a mechanism for regulating the functional activity of proteins, but also as a mechanism for protecting them from oxidative damage. Persulfidized residues can be converted into regular sulfhydryl groups using the thioredoxin system (Trx/TrxR) [159]:
protein-SSH+Trx/TrxR→protein-SH.
Data obtained using bioinformatics methods indicate that up to 5% of the plant cell proteome may undergo persulfidation [160]. As a result of the PTM, target proteins change their stability, biochemical activity, conformation, subcellular localization, and protein-protein interaction abilities [161].
Persulfidation is likely to be a component of the gene expression regulation toolbox. For example, in tomato, activation of 5349 genes and repression of 5536 genes were recorded after NaHS treatment of the roots [162].
Many physiological effects of hydrogen sulf ide are mediated by other components of the signaling network, such as ROS. NADPH oxidase, a key ROS-generating enzyme, is known to be activated by persulfidation by hydrogen sulfide [163]. Also, as noted above, the signaling effects of H2S may be related to its functional interaction with NO. Moreover, such interactions may be synergistic or antagonistic.
Another universal and crucial cell mediator, calcium, is also closely associated with the signaling potential of hydrogen sulfide. Thus, calcium entry into the cytosol can be a stimulus for hydrogen sulfide synthesis. For example, DES activity was increased in cultured tobacco cells under the influence of exogenous calcium and calmodulin, leading to enhanced formation of endogenous H2S [164]. Calcium also appears to be involved in H2S signaling, which induces the development of plant resistance to abiotic stresses. The possibility of persulfidation of calcium channels with increasing concentration of hydrogen sulfide in the submembrane space has been considered [165].
3.2 Main Mechanisms of H2S Influence on State of Stomata
Hydrogen sulfide can be synthesized directly in guard cells. The most studied phenomenon is the increased synthesis of hydrogen sulfide in guard cells associated with activation of gene DES expression under the action of ABA [149]. The direct effect of hydrogen sulfide donors on stomatal state and the effect of stressors on stomatal status due to stress-induced increases in H2S synthesis have also been studied. For example, it has been shown that under the action of osmotic stress induced by polyethylene glycol, stomatal aperture decreases in Arabidopsis Col-0 plants, whereas it is almost unchanged in des mutants defective in genes encoding enzymes involved in hydrogen sulfide synthesis, cysteine desulfhydrases [166]. However, the response of the stomatal apparatus to hydrogen sulfide donors in model experiments (using isolated epidermis or detached leaves) remains unclear. For example, exposure to 100 μM sodium hydrosulfide for 90 min caused a decrease in stomatal aperture in Arabidopsis plants [167]. Treatment of sweet potato leaf epidermis with 100 μM NaHS resulted in an increase in the relative number of fully closed stomata [168]. Concurrently, Lisjak et al. [169] demonstrated an increase in stomatal aperture in Arabidopsis and pepper plants following 2.5-h light treatments of the epidermis with 100 and 200 μM NaHS. Furthermore, this treatment prevented stomatal closure in the dark. An increase in the relative number of open stomata was also observed in rice plants treated with a 100 μM sodium hydrosulfide solution [170]. However, in experiments with the organic hydrogen sulfide donor GYY4137 (morpholin-4-ium-4-methoxyphenyl [morpholino] phosphinodithioate), treatment of detached Arabidopsis leaves resulted in stomatal closure after 90 min and, conversely, an increase in aperture after 120 min followed by a decrease after 180 min [63]. In other words, rhythmic changes in the stomatal aperture size were recorded. In our studies using whole rosette leaves of Arabidopsis (Col-0), we found that treatment with NaHS at a wide range of concentrations (5–250 μM) caused a decrease in stomatal aperture. The maximum effect on stomatal closure was observed 90 min after the start of H2S donor treatment and after 180 min of exposure; in contrast, the stomatal aperture in the NaHS-treated variants was significantly wider than that in the control [171]. Thus, the effects of hydrogen sulfide donors on stomata may have complex temporal dynamics.
It is also necessary to distinguish between the direct effects of stomatal closure connected with increase of hydrogen sulfide content in guard cells (for example, at treatment of epidermis or isolated leaves with hydrogen sulfide donors) and the long-term systemic effects of hydrogen sulfide donors on intact plants. In the first case, there is a more frequent closure of stomata. In the second case, the development of many adaptive reactions with participation of hydrogen sulfide in plants can develop the ability to keep stomata in the open state under the action of unfavorable factors. For example, it has been demonstrated that repeated treatment of rice plants with 10 μM NaHS resulted in an increase in the aperture of the stomata and their density on the leaf surface, as well as an increase in the photosynthetic rate and a decrease in the photorespiration rate [170]. Nevertheless, it is now well established that an increase in H2S content in guard cells plays a pivotal role in regulating stomatal closure in response to various environmental stresses and plant hormones, particularly ABA and JA [149].
Increased activity and gene expression of the main enzyme of hydrogen sulfide synthesis, DES, and the associated increase in cellular hydrogen sulfide content have been observed in plants of different species in response to the classical osmotic stresses of drought and salinity [172,173]. A causal relationship between increased hydrogen sulfide and stomatal closure has also been established by various methods (using des mutants or by applying scavengers or inhibitors of hydrogen sulfide synthesis). Furthermore, as mentioned above, numerous studies on plants of different species have shown stomatal closure in response to exogenous hydrogen sulfide.
The participants of the hydrogen sulfide signal transduction pathway that is necessary for the realization of the effect of stomatal closure remain largely unknown. However, the available experimental data allow for the discussion of potential models of the process of hydrogen sulfide-mediated control of the stomatal state (Fig. 6). It is postulated that the necessary components of the stomatal effect induced by hydrogen sulfide are the PTMs of several signaling system proteins. It should be noted that studies of these protein PTMs have been carried out in recent years, mainly in the context of the involvement of hydrogen sulfide in ABA signaling [174]. One of the first effects in the formation of such a signal is the persulfidation of Cys44 and Cys205 in one of the main enzymes in hydrogen sulfide synthesis, DES [175]. In other words, hydrogen sulfide acts as a self-enhancing factor for its own synthesis. Under the influence of ABA, DES1 expression also increased [163]. However, the mechanisms underlying these phenomena are not fully understood.
Figure 6: Hydrogen sulfide signaling pathway and its involvement in abscisic acid-induced stomatal closure. Abscisic acid (ABA) causes activation of desulfhydrase (DES1), which increases the level of hydrogen sulfide (H2S) in cells. DES1 activity can also be increased by persulfidation associated with the action of exogenous H2S and/or an increase in the concentration of endogenous H2S. Under the action of H2S, the protein kinase OST1 (Open Stomata 1) is also persulfidated, leading to modulation of the transcription factor ABA response element-binding factor 2 (ABF2), which is thought to affect calcium homeostasis. In addition, elevated levels of H2S cause persulfidation of the transcription factor ABI4 (ABSCISIC ACID INSENSITIVE 4), which leads to activation of the mitogen-activated protein kinase (MAPK) cascade, also affecting calcium homeostasis. Under the influence of H2S, persulfidation of phospholipase D (PLD) also occurs, resulting in the accumulation of phosphatidic acid (PA). PA activates NADPH oxidase (RBOH), resulting in increased generation of reactive oxygen species (ROS) and nitric oxide (NO). The increase in cytosolic calcium concentration (Ca2+cyt) with the participation of NO and other factors causes activation of calcium-dependent protein kinases (CDPKs) and modulation of ion channels. ROS and NO can also influence the state of ion channels and the turgor of guard cells. Blunt-ended arrows indicate inhibition. Interrupted arrows indicate the presence of unspecified mediators. Dotted arrows indicate hypothetical pathways without direct experimental confirmation
Persulfidation of OST1 at Cys131 and Cys137, which are adjacent to the catalytic loop of the kinase, and the major Ser175 phosphorylation site [176], is thought to be one of the major events that occur following an increase in hydrogen sulfide in guard cells. Persulfidation of OST1 alters its protein structure, bringing the Ser175 residue closer to the phosphate acceptor Asp140 and enhancing its catalytic activity [176]. As a result, the ability of OST1 to phosphorylate ABF2 is increased (Fig. 6). In vitro treatment of OST1 from wild-type Arabidopsis plants with hydrogen sulfide resulted in increased phosphorylation of ABF2, whereas mutation of both Cys sites in OST1 resulted in loss of ABF2 binding and phosphorylation activity [174]. The precise function of ABF2 remains unclear. However, evidence suggests that protein phosphorylation is associated with an increase in Ca2+ influx into the cytosol of guard cells [149].
Transcription factor ABI4 (ABSCISIC ACID INSENSITIVE 4) is another protein important for stomatal regulation that undergoes persulfidation. ABI4 is a versatile transcription factor that is thought to be a component of the ABA signaling pathway (Fig. 6). The abi4 mutants are characterized by a lack of sensitivity to ABA, including stomatal responses [177,178]. Persulfidation of ABI4 at Cys250 enhances its transactivation activity on one of the proteins that activates the MAP kinase cascade (MAPKKK18) [149,178]. In addition, persulfidation of ABI4 can enhance the expression of DES1, thus enabling the function of an amplified regulatory loop in H2S signaling in guard cells [149].
NADPH oxidase is also an important target for ABA- and hydrogen sulfide-induced persulfidation (Fig. 6). H2S accumulation causes persulfidation of residues Cys825 and Cys890 in the molecular form of RBOHD, which increases enzyme activity and promotes ROS accumulation in guard cells [179]. Accumulation of ROS in guard cells is thought to be one of the phenomena required for stomatal closure. In particular, enhancement of ROS generation by NADPH oxidase is known to increase calcium entry into guard cells [149]. However, persulfidation of Cys825 and Cys890 is not the only known mechanism of NADPH oxidase activation by hydrogen sulfide. Using a molecular genetic approach, phosphatidic acid has recently been shown to be involved in hydrogen sulfide-induced enhancement of ROS formation in Arabidopsis guard cells. Using a PLDδ-deficient mutant, impaired hydrogen peroxide formation in guard cells was shown to be a response to hydrogen sulfide treatment [172]. Plants of this genotype exhibited significantly higher water loss under drought conditions than wild-type plants. Exogenous exposure to phosphatidic acid and hydrogen sulfide donor alleviated the effects of leaf wilting and yellowing in the phosphatidic acid synthesis mutant pldδ, as well as in mutant with impaired hydrogen sulfide synthesis (lcd) and double mutant (pldδlcd) under osmotic stress. In addition, exogenous H2O2 treatment of pldδ, lcd, and pldδlcd plants induced stomatal closure under osmotic stress. Data were also obtained on the contribution of another isoform, phospholipase Dα1 (PLDα1), to the regulation of hydrogen sulfide formation and stomatal closure in Arabidopsis. It has been shown that the levels of LCD transcription and translation are lower in pldα1 than in wild-type plants [173]. These results suggest that hydrogen sulfide, phosphatidic acid, and hydrogen peroxide are required for stomatal closure under osmotic stress and that PLDα1, PLDδ, and LCD act upstream of H2O2 [172].
Thus, considering the functional relationship between hydrogen sulfide and ROS, we can state the existence of at least two mechanisms for the increase in NADPH oxidase activity under the action of hydrogen sulfide. One is related to the persulfidation of cysteine residues in the molecular form of RBOHD, and the other is related to the activation of the enzyme by phosphatidic acid (Fig. 6).
However, regulation of NADPH oxidase activity by hydrogen sulfide is not the only mechanism by which it affects ROS generation. Recently, the mitochondria-directed H2S donor AP39 was shown to induce stomatal closure in a dose-dependent manner [180]. Experiments using mitochondrial electron transfer chain inhibitors or cytochrome c (CYTc) insertion mutants have shown that mitochondrial CYTc and/or complex IV activity are required for AP39-dependent stomatal closure. It has also been reported that AP39 hyperpolarizes the mitochondrial inner potential, increases cytosolic ATP and hydrogen peroxide levels, and enhances oxidation of glutathione pool in guard cells. Thus, H2S donors targeting mitochondria modulate cytosolic energy and redox status, thereby inducing stomatal closure [180]. There are other mechanisms of hydrogen sulfide influence the energy status of guard cells. Recently, it has been shown that treatment of cells with hydrogen sulfide causes persulfidation of succinate dehydrogenase and increase in its activity, as well as enhances SDH1-1 gene expression [181]. The authors believe that succinate dehydrogenase may act as the main node to maintain the energy processes that ensure the movement of guard cells under the action of hydrogen sulfide.
Hydrogen sulfide-induced changes in ROS levels and phosphorylation processes, in turn, lead to changes in ion fluxes, reduction of guard cell turgor, and stomatal closure (Fig. 6). Thus, using Nicotiana tabacum as an example, it has been shown that submicromolar concentrations of hydrogen sulfide cause selective inactivation of K+in guard cell channels [163,182]. It is noteworthy that the authors were able to separate the effect of H2S from that of ABA using H2S antagonists, thereby demonstrating the unique signaling effect of H2S. Furthermore, it has been demonstrated that this mechanism of potassium channel regulation by hydrogen sulfide is not inhibited by calcium binding to EGTA [182]. Thus, inhibition of potassium entry into guard cells reduces turgor and promotes stomatal closure. Concurrently, H2S directly or indirectly enhances transport through the K+out channels of plasma membrane. The mechanism by which hydrogen sulfide exerts its effects on this class of potassium channels remains poorly understood. However, the possibility of direct persulfidation of ion channel proteins has been considered, similar to the effects observed in animal cells [149]. Using Arabidopsis mutants with different levels of endogenous hydrogen sulfide, it was shown that the intensity of K+ flux from guard cells was closely correlated with the H2S content of the plants [166].
Another mechanism by which hydrogen sulfide reduces osmotic pressure in guard cells is linked to the activation of anion release. Low concentrations of H2S have been demonstrated to facilitate SLAC1 phosphorylation in a calcium- and protein kinase OST1-dependent manner [4,183]. In more recent studies on the drought response of Chinese cabbage plants, chloride channel activation was identified as one of the main causes of stomatal closure in the presence of hydrogen sulfide [184].
Thus, in general, it seems that hydrogen sulfide can be considered not only as a mediator of ABA in the regulation of stomatal state, but also as an independent signal regulating many processes in guard cells (Fig. 6). In this case, however, hydrogen sulfide is involved in signaling processes involving ROS and calcium. The stomatal effects of hydrogen sulfide also include its functional interaction with NO. One of the most prominent examples of this interaction is the nitrosation of OST1 at Cys137, resulting in the inhibition of this key protein kinase for stomatal responses [185]. As mentioned above, hydrogen sulfide activates it by persulfidation at Cys131 and Cys137. Thus, it is suggested that NO can inhibit ABA-induced stomatal closure by antagonizing hydrogen sulfide [149]. Another potential mechanism for the negative regulation of ABA- and hydrogen sulfide-induced stomatal closure by NO may be the nitrosation of Cys 890 in the RBOHD subunit [65]. This results in a reduction in ROS generation and likely inhibition of ROS-mediated hydrogen sulfide signaling. Nevertheless, further investigation is required to elucidate the impact of NO-mediated modifications of RBOHD on ABA signaling in guard cells [149].
Furthermore, there are instances where NO and H2S engage in antagonistic interactions regarding stomatal responses, even in the absence of ABA. It was demonstrated that the co-treatment of Vicia faba epidermis with donors of two GTs, NaHS and SNP, in contrast to their separate application, did not induce stomatal closure [24]. Blocking of H2S-induced stomatal closure was also caused by another reactive nitrogen species, nitroxyl (HNO/NO–). The authors showed that a specific HNO donor (Angeli’s salt) eliminated stomatal closure induced by exogenous H2S [24]. Notably, the HNO donor itself, unlike the NO donor, did not cause the stomatal closure effect in V. faba, indicating significant differences in the biological effects of these RNS. However, the specific role of HNO/NO– and its interactions with other mediators in plant cells remains largely unexplored. It is anticipated that the recent emergence of new methods for in situ HNO/NO– analysis will contribute to such knowledge [48].
Despite the above-described mechanisms of possible antagonism between hydrogen sulfide and NO in the reactions of stomatal closure, experimental data suggesting the involvement of NO as a mediator in the realization of stomatal effects of hydrogen sulfide have also been obtained. Honda et al. [63] showed that the “slow” hydrogen sulfide donor GYY4137 induced NO formation in Arabidopsis guard cells, and that GYY4137-induced stomatal closure was reduced by NO scavengers and inhibitors of NO-producing enzymes. It has been demonstrated that GYY4137 treatment induces the synthesis of 8-nitro-cGMP and 8-mercapto-cGMP, and that this synthesis is mediated by NO. In addition, direct treatment with 8-mercapto-cGMP induced stomatal closure. Furthermore, inhibitor and genetic studies have shown that calcium, cADP-ribose, and SLAC1 act downstream of 8-mercapto-cGMP. Thus, this study demonstrated that 8-mercapto-cGMP mediates the H2S signaling cascade in guard cells. The manner in which the associated positive hydrogen sulfide-NO interaction-related pathway of stomatal regulation relates to the antagonistic effects described above remains unclear, as the data were obtained in different experimental models. Subtle compartmentalization may allow these processes to coexist in guard cells. Additionally, the nature of the effects may depend on the concentrations of NO and H2S in guard cells.
Furthermore, the sequence of mediators in the signaling chains that ensure stomatal closure is likely to vary depending on the nature of the stressor causing this effect. For example, a study of the effects of salt stress on A. thaliana demonstrated that exposure of epidermal strips to NaCl initially resulted in the generation of H2O2 and NO, subsequently leading to H2S synthesis, and ultimately to stomatal closure [186]. In particular, the authors demonstrated that H2O2 and NO modulators prevented salt stress-induced H2S synthesis and increased L- and D-cysteine desulfhydrases activity in leaves. However, H2S modulators did not inhibit salt stress-induced H2O2 production or NO synthesis.
3.3 Involvement of Hydrogen Sulfide in the Realization of Stomatal Effects of Plant Hormones
The close relationship between the effects of hydrogen sulfide and the signals induced by ABA, the main hormone responsible for stomatal closure, has been discussed above. However, there is evidence that hydrogen sulfide can mediate the induction of stomatal closure by other plant hormones such as ethylene, JA, and brassinosteroids (Fig. 7) [64,175,186]. Thus, exogenous ethylene was found to induce the expression of the D-cysteine desulfhydrase gene AtD-CDes and its enzymatic activity. At the same time, the Atd-cdes mutant was insensitive to ethylene and H2S synthesis inhibitors abolished ethylene-induced stomatal closure in Arabidopsis [187]. In the same study, it was demonstrated that the overexpression of AtD-CDes in transgenic plants resulted in increased sensitivity to ethylene. Additionally, hydrogen sulfide mediates the stress-protective effects of endogenous ethylene synthesized in rice in response to heat stress [188]. Experiments with inhibitors of ethylene and hydrogen sulfide synthesis have shown that their functional interaction are necessary for the regulation of stomatal status and the formation of non-stomatal adaptive responses.
Figure 7: Involvement of reactive oxygen species and hydrogen sulfide in the manifestation of stomatal responses induced by ethylene, jasmonic acid, and brassinosteroids. Jasmonic acid (JA) or methyl jasmonate (MeJA) causes activation of extracellular peroxidase (PO) and/or NADPH oxidase (RBOH), resulting in increased generation of reactive oxygen species (ROS). Under the influence of ROS, desulfhydrase (DES) is activated and hydrogen sulfide (H2S) levels are increased. 24-epibrassinolide (EBR) also causes an increase in H2S mediated by ROS generated by RBOH. Ethylene (ET) activates DES by an unknown mechanism. The increase in H2S under the influence of various plant hormones modifies the state of ion channels, leading to stomatal closure. Interrupted arrows indicate the presence of unspecified mediators. Dotted arrows indicate hypothetical pathways without direct experimental confirmation
Together with ABA and ethylene, JA is important for the response of the stomatal apparatus to stress factors, as mentioned above. Over a decade ago [189] investigated the functions of H2S and H2O2 in signaling in JA-mediated regulation of stomata in beans. Inhibitors of H2S synthesis (aminooxyacetic acid, hydroxylamine, and potassium pyruvate), H2O2 scavenger (ascorbic acid), and inhibitors of H2O2 synthesis (salicylhydroxamic acid and diphenyleneiodonium) reduced JA-induced stomatal closure. Direct assays showed that JA increased H2O2 and H2S levels and L- and D-cysteine desulfhydrase activity in leaves and specifically in guard cells. Application of the H2O2 scavenger mitigated the impact of JA-induced elevations in desulfhydrase activities and H2S content. Thus, hydrogen sulfide and hydrogen peroxide are involved in signal transduction during JA-induced stomatal closure, with H2S downstream of H2O2 in the signaling cascade (Fig. 7).
In addition to its role in regulating stomatal closure, H2S may participate in the development of the stomatal apparatus. It was demonstrated that the H2S scavenger hypotaurine abolished JA-induced repression of stomatal development in the epidermis of wild-type Arabidopsis. Concurrently, H2S-deficient mutant lcd plants exhibited augmented stomatal density and elevated stomatal index values [190]. These attributes in the mutant could be approximated to those of wild-type plants through sodium hydrosulfide treatment yet not by JA. Based on these findings, the authors propose that JA-mediated repression of stomatal development is contingent on H2S biosynthesis.
The functional interaction between hydrogen sulfide and JA may not be related only to the involvement of hydrogen sulfide in JA-induced signaling pathways. There is evidence of a possible role of hydrogen sulfide in the induction of JA synthesis in plants. In particular, data obtained by bioinformatic methods indicate that almost half of the proteins associated with jasmonate biosynthesis are persulfidized [191]. There is also experimental evidence of such a link between H2S and JA. For example, fumigation of millet plants with hydrogen sulfide increased their MeJA content [192]. Treatment of tomato plants with an H2S donor increased JA levels in the leaves under normal conditions and under salt stress [193].
Another mechanism linking hydrogen sulfide and JA may be the involvement of individual components of the jasmonate signaling pathway in the transduction of H2S signals into the genetic apparatus. For example, molecular genetic methods have been used to show that activation of the expression of the gene encoding the regulatory transcription factor MYC2/JIN1 is involved in the enhanced adaptation of Arabidopsis plants to hypoxia in the dark by the action of exogenous hydrogen sulfide [194]. This work also demonstrated the involvement of endogenous hydrogen sulfide as a signaling mediator in the activation of MYC2 expression. A comparative analysis of the impact of the hydrogen sulfide donor NaHS on wild-type Arabidopsis plants (Col-0) and mutants in the gene encoding the MYC2/JIN1 protein (jin1) revealed an enhancement in salt tolerance in wild-type plants, as evidenced by a reduction in oxidative damage, diminished water deficit, and maintenance of the photosynthetic pigment pool under salt stress [195]. Additionally, the treatment of wild-type plants with H2S donor prevented the stress-induced decrease in the activity of antioxidant enzymes. Conversely, exposure of jin1 plants to exogenous H2S did not result in enhanced salt tolerance or alterations in the status of stress-protective systems. These phenomena suggest the involvement of the transcription factor JIN1/MYC2 in the physiological effects of hydrogen sulfide [103]. However, direct experimental evidence for the involvement of JIN1/MYC2 in hydrogen sulfide signal transduction is still lacking. It is unclear whether the process of stomatal closure induced by hydrogen sulfide can depend on the function of the JIN1/MYC2 protein. The involvement of JIN1/MYC2 in the manifestation of stomatal effects of JA and ABA is well known [106]. However, the stomatal effects of NO, a GT that, as noted above, did not fully induce salt tolerance in jin1 mutants, manifested in this genotype almost as well as in wild-type plants. Thus, the mechanism underlying the possible effect of hydrogen sulfide signaling on the JIN1/MYC2 transcription factor remains unclear.
Brassinosteroids are another class of stress plant hormones that modulate stomatal state and can promote both stomatal opening and closure, depending on their concentration and interactions with other mediators. Ma et al. [196] showed the inhibition of 24-epibrassinolide (EBR)-induced stomatal closure in wild-type Arabidopsis by H2S scavengers and inhibitors of its synthesis. Stomatal closure was also inhibited by H2O2 antagonists. In addition, the authors showed that EBR failed to close stomata in mutants of the genes for hydrogen sulfide and ROS-generating enzymes Atl-cdes, Atd-cdes, AtrbohF, and AtrbohD/F. Based on the results of inhibitor analysis and experiments with ROS and H2S synthesis mutants, the authors concluded that EBR induces AtRBOHF-dependent H2O2 generation and subsequent H2S production, catalyzed by cysteine desulfhydrases, leading to stomatal closure in A. thaliana (Fig. 7).
Recently, convincing evidence for the involvement of hydrogen sulfide in the effect of melatonin on the state of stomata in Arabidopsis has also been obtained [197]. The authors showed that the increase in drought tolerance of plants by exogenous melatonin was accompanied by increased expression of cysteine desulfhydrase genes and increased hydrogen sulfide content in guard cells. Both melatonin and exogenous hydrogen sulfide increased the expression of genes encoding proteins of guard cell K+out channels (Fig. 7). In addition, persulfidation of these proteins increased under the influence of melatonin and hydrogen sulfide, thereby decreasing their degradation rate [197].
In general, ROS and hydrogen sulfide appear to be the main mediators of the stomatal effects of a number of plant hormones such as ABA, ethylene, jasmonates, and brassinosteroids. However, the functional interaction of hydrogen sulfide and nitric oxide during stomatal closure by these plant hormones remains unexplored.
4 Carbon Monoxide as a Possible Participant in Regulating Plant Stomatal Responses
Carbon monoxide (CO) is one of the least studied GTs in plant cells [198,199]. The mechanisms underlying the biological activity of CO differ significantly from that of NO and hydrogen sulfide. It is postulated that these effects are primarily related to the formation of coordination bonds between CO and metals in the active centers of proteins, particularly heme-containing proteins [200]. The molecular targets of CO action associated with its manifestation of certain physiological effects remain unclear [201]. Nevertheless, experimental evidence suggests that CO plays a role in the regulation of processes related to plant growth and development [202], and adaptation to abiotic stresses [23], including the control of stomatal movements [8].
The main enzyme providing CO synthesis in both animals and plants is considered to be heme oxygenase (HO). It catalyzes the conversion of heme to biliverdin IXα, with the release of Fe2+ and CO [203]. This reaction involves NADPH as a source of electrons and molecular oxygen [204]. In plant cells, the enzymatic HO-1 protein is found in chloroplasts (mainly in their stroma) mitochondria [203].
Plant hemoxygenases are a family of four genes. However, HO-1 is most intensively expressed in plants. This gene is induced by a variety of environmental factors, including osmotic stress [205], salinization [206], heavy metals [207], and UV-B [208].
The pathways of CO elimination in plant cells remain poorly understood. Binding of CO to hemoglobin iron is thought to be one of the major pathways for its inactivation [209].
The phenomenology of the effects of exogenous CO on the state of stomata is still rather narrow. In experimental settings, exogenous heme oxygenase inducers such as haematine and hemin are typically employed. Thus, the closure of stomata in beans under the action of haematine and aqueous CO solution was revealed [210]. Data indicating the involvement of the main signaling mediators (hydrogen peroxide and NO) in the manifestation of stomatal effects of exogenous CO were also obtained. Both an H2O2 scavenger (ascorbic acid) and an NADPH oxidase inhibitor (diphenyleneiodonium) abolished the increase in ROS content upon haematine action and prevented stomatal closure. The authors concluded that ROS are involved in CO signal transduction that causes stomatal closure in Vicia faba [210].
Using the same model (V. faba epidermis), it was shown that stomatal closure induced by haematine treatment was mediated by an increase in NO synthesis, as it was accompanied by an increase in NO content in guard cells and was abolished by NO synthesis inhibitors [211]. At the same time, stomatal closure induced by the NO donor sodium nitroprusside was not abolished by the heme oxygenase inhibitor Zn-protoporphyrin IX, indicating that NO is downstream of CO in the signaling chain that regulates stomatal closure and not vice versa. The involvement of cGMP as a downstream mediator after NO in the carbon monoxide-initiated signaling pathway leading to stomatal closure has also been demonstrated [212].
Carbon monoxide is likely to be functionally related to plant hormone system [213]. Thus, stomatal closure in beans under the action of ABA was accompanied by an increase in heme oxygenase activity and CO content in guard cells [214]. However, the specific role of CO in the stomatal effects of ABA has not been investigated.
5 Carbon Dioxide Signaling in Stomatal Regulation
Carbon dioxide is a product of plant metabolism, the main substrate for photosynthesis, and a gas that is constantly present in large amounts in the intercellular space and environment. Moreover, since the industrial revolution, its atmospheric content has increased by more than 1.5 times, causing planetary environmental problems [215]. None of this is the reason for considering CO2 as a classical GT. However, in recent years, some researchers have included it in the list of GTs that are important for plant life [29]. CO2 meets certain criteria by which gaseous compounds can be classified as GTs.
Carbon dioxide has the ability to penetrate cell membranes, including with the help of aquaporins [29]. It is involved in signaling processes that induce the synthesis of other mediators in cells, especially hydrogen peroxide and NO, which are related to classical GT [216]. Ultimately, the conversion of CO2 into a signaling intermediate, the HCO3– anion, is catalyzed by carbonic anhydrase. The concentration of CO2 in plant tissues is largely dependent on the activity of Rubisco [217]. Thus, despite the constant presence of this gas in the external and internal environments, its amount and biological activity can be regulated to some extent by the plant, which in turn gives reason to consider CO2 as another gaseous bioregulator, including a regulator of stomatal movements.
In recent years, ideas about the molecular mechanisms regulating the state of stomata under the influence of carbon dioxide have emerged. An increase in the concentration of CO2 in the environment contributes to the closure of stomatal pores in plants, and conversely, a decrease in [CO2] causes the opening of stomatal pores [28]. In addition, the long-term effect of increasing CO2 concentration in the environment surrounding the plant can be the suppression of stomatal development in the leaf epidermis [218], although some species do not show such a response [219]. Either way, changes in CO2 concentration can significantly affect stomatal conductance.
The information obtained thus far allows us to describe the signaling mechanism of stomatal closure caused by the action of an increased concentration of carbon dioxide. Intercellular CO2 can induce stomatal closure during drought, which has a protective value [29].
The entry of CO2 into guard cells in Arabidopsis is mediated by PIP2 and possibly other aquaporins [215]. Passive entry of carbon dioxide into cells is also possible. Carbonic anhydrases appear to be the next participants in the effect of CO2 on the state of stomata. Under the influence of carbonic anhydrase, CO2 is converted to HCO3– anions (Fig. 8). There is evidence that β-carbonic anhydrases play a specific role in this process, but the importance of α- and γ-carbonic anhydrases remains poorly understood [28]. In particular, molecular evidence has been obtained for the involvement of several forms of β-carbonic anhydrase (βCA1, βCA2, and βCA4) in stomatal closure under the influence of high carbon dioxide concentrations [215,220]. It is not yet known whether guard cells contain proteins that act as specific sensors of hydrocarbonate ions. However, data have been obtained regarding their ability to directly increase SLAC1 channel activity [221]. Bioinformatic methods were used to identify the SLAC1 regions that interact with HCO3–. The proposed models were partially experimentally validated. Gas exchange experiments with slac1-transformed plants showed that the SLAC1 residue R256 is required for carbon dioxide regulation of stomatal movements in planta, but not for ABA-induced stomatal closure. Thus, there is reason to believe that the SLAC1 anion channel is one of the physiologically important specific HCO3– sensors in guard cells [221]. Other models of SLAC1 regulation by hydrocarbonate action have also been proposed, for example involving the kinases MPK 4/12 and GHR1 (GUARD CELL HYDROGEN PEROXIDE RESISTANT1) [215]. The latter protein is considered a receptor-like (pseudo) kinase that is localized to the Arabidopsis plasma membrane [222]. It has been suggested that GHR1 regulates SLAC1 activity via phosphorylation [223]. However, no direct evidence for the kinase activity of GHR1 has been obtained, although a mutation in the gene encoding this protein impairs stomatal closure induced by high CO2 levels. Currently, a model has been proposed in which GHR1 acts as a structural protein between proteins that ensure SLAC1 activation (Fig. 8) [215].
Figure 8: Possible pathways of carbon dioxide (CO2) influence on the state of ion channels of stomatal guard cells. βCA—β-carbonic anhydrase; CDPKs—calcium-dependent protein kinases; GHR1—protein GUARD CELL HYDROGEN PEROXIDE RESISTANT1; SLAC1—slow-type anion channel 1; NR—nitrate reductase; MPK 4/12—mitogen-activated protein kinase; OST1—protein kinase Open Stomata 1; NO—nitric oxide; RBOH—NADPH oxidase; ROS—reactive oxygen species. Interrupted arrows indicate the presence of unspecified mediators. Blunt-ended arrows indicate inhibition. Dotted arrows indicate hypothetical pathways without direct experimental confirmation. Other explanations in the text
The relatively long-term effects of CO2-induced stomatal closure are accompanied not only by activation of SLAC1 anion channels present in guard cells but also by increased expression of SLAC1 genes. This effect, as well as carbon dioxide-induced stomatal closure, was disrupted by the suppression of OST1 activity, indicating that this protein kinase is involved in signaling not only ABA but also CO2 to induce stomatal closure [216].
However, the involvement of OST1 and the interaction between CO2 and ABA signals in stomatal closure remains unclear. Several experimental data indicate that carbon dioxide can affect the state of stomata without the involvement of ABA. It has been shown that mutants in ABA biosynthesis, nced3/nced5 and aba2-1, are sensitive to elevated CO2 levels and, like wild-type plants, show activation of S-type anion channels. In mutants of the ABA receptor PYR/RCAR, stomatal closure also occurred under the influence of CO2, but its dynamics were delayed [88]. The same study showed that, in contrast to ABA, elevated CO2 did not activate OST1 kinase in guard cells. Based on the results of these studies, the authors proposed a model in which rapid CO2 signaling leading to stomatal closure occurs through an ABA-independent pathway downstream of OST1 [88]. At the same time, other authors propose a model in which an increase in CO2 concentration can cause both ABA-independent and ABA-mediated stomatal closure [220]. The ambiguity of the experimental data on the relationships between the effects of CO2 and ABA and the function of OST1 may be related to different time phases of the responses of the stomatal apparatus to high CO2 concentrations. In other words, the contribution of different mediators to the dynamics of the stomatal response may change.
Several studies have indicated the importance of signaling mediators downstream of the OST1 kinase in the regulation of CO2 effects [216]. These include ROS, NO, and calcium ions. For example, a decrease in stomatal aperture caused by elevated ambient CO2 in tomatoes subjected to heat stress was accompanied by an increase in the amount of RBOH1 transcripts, apoplastic accumulation of H2O2, and a decrease in stomatal aperture [224]. In plants with suppressed expression of RBOH1 and SLAC1, there was no significant decrease in stomatal aperture under the influence of elevated CO2, either at normal temperature or under heat stress. At the same time, water use efficiency and resistance to heat stress decreased. At the same time, the treatment of plants with suppressed expression of the RBOH1 gene with hydrogen peroxide helped to normalize the water balance by closing the stomata. The authors concluded that RBOH1 activity-dependent H2O2 accumulation is involved in high CO2-induced stomatal closure and that this effect is important for maintaining optimal water balance under stress conditions [224].
Data have also shown that ROS generated by RBOH1 affect the state of stomata and are in a functional relationship with NO generated by NR. In tomato plants with reduced expression of the NR gene, the NO content in guard cells did not increase in response to CO2 treatment [216]. Additionally, increased expression of SLAC1 was not observed in these plants under elevated carbon dioxide levels. At the same time, the authors showed that changes in H2O2 and NO levels caused by elevated carbon dioxide concentrations were not dependent on ABA synthesis and were manifested in mutants with reduced levels of this hormone. At the same time, in plants with impaired synthesis of hydrogen peroxide and NO in response to treatment with high concentrations of CO2, as in normal plants, the expression of the OST1 protein kinase gene in guard cells increased. In this regard, the authors concluded that H2O2, synthesized by the participation of RBOH1, and NO, formed under the influence of NR, act as critical signaling molecules downstream of OST1 and regulate the expression of SLAC1, which is necessary for the reduction of the stomatal aperture (Fig. 8) [216].
The signaling cascade induced by elevated CO2 which leads to stomatal closure, does not only involve an increase in anion release from guard cells due to the opening of slow SLAC1 anion channels. At the same time, an increase in CO2 concentration inactivated K+ channels responsible for the entry of potassium into guard cells and activated K+out channels [220] (Fig. 8).
The regulation of potassium channels by the high CO2 signaling cascade appears to involve protein phosphorylation by calcium-dependent protein kinases. Thus, cpk3/5/6/11/23 mutants were defective in stomatal closure induced by high CO2, but the same plants were also unable to respond to a decrease in CO2 concentration by opening their stomata [225]. Thus, specific protein kinases can regulate different types of potassium channels involved in stomatal responses to the modulation of carbon dioxide levels (Fig. 8).
Thus, the effect of high CO2 concentrations on the stomatal state is associated with changes in the state of ion channels. Such effects can be achieved both by the direct action of hydrocarbonate, as shown for SLAC1 channels, and apparently by the activation of a signaling network that includes ROS, NO, and calcium ions. These mediators are likely involved in regulating the activity of protein kinases that activate the corresponding ion channels. How these phenomena relate to the action of ABA and the protein kinase OST1, which is important for the regulation of ion channels, has not been well studied.
Apparently, it can be assumed that when stomata close, stimulated by high CO2 concentrations, this gas functionally interacts with at least one classical GT, nitric oxide. No data are available on the involvement of other GTs in these processes.
The ability to rapidly adapt the stomatal apparatus to changes in the environment determines the ability of the plant to maintain water balance and the supply of inorganic carbon necessary for photosynthesis. The state of the stomata is regulated by a complex network of plant hormones and signaling mediators. This review focuses on the role of GTs in stomatal regulation. However, despite a significant expansion in the range of compounds that have been found to be involved in stomatal regulation, an increase in ABA content in guard cells is still considered to be the most potent and specialized hormonal signal for stomatal closure. ABA is mainly produced in vascular tissues. In this context, the manifestation of ABA effects depends on its transport across the plasma membrane into the protoplasts of the target cells. In recent years, considerable attention has been paid to investigating the mechanisms of ABA transport and the proteins responsible for the degradation of ABA transporters [226–228]. A relationship between stomatal status and the expression of AtABCG25, which encodes a protein that transports ABA to guard cells, has been shown [218]. It was also found that Arabidopsis snx2 mutants, which are defective in the gene encoding the protein responsible for the degradation of the ABCG25 transporter, are characterized by a more efficient release of ions from guard cells under the action of ABA and increased drought tolerance [227]. These phenomena can be considered strong evidence for the role of stomatal regulation in plant resistance at the organismal level. However, the stomatal effects of ABA depend not only on the proteins that regulate its transport and degradation but also on the mediators of intracellular ABA signaling.
During the last decade and a half, it has been established that the main signaling mediators responsible for the effect of ABA on stomatal state are ROS generated by separate molecular forms of NADPH oxidase, NO generated mainly by the reductive pathway, and cGMP and cADP-ribose as mediators binding NO and cytosolic calcium. An increase in the concentration of the latter activates the corresponding protein kinases, which stimulate anion channels while inhibiting the potassium entry into cells. These effects lead to a decrease in guard cell turgor and stomatal closure. In recent years, however, numerous findings have indicated that ROS and NO are involved in stomatal regulation in obligate cooperation with another GT, hydrogen sulfide. In particular, hydrogen sulfide is required for the activation of NADPH oxidase and the most important protein kinase involved in stomatal responses, OST1 [163].
It should be noted that a similar set of mediators is involved in the stomatal effects of other plant hormones such as JA, ethylene, brassinosteroids, and melatonin. However, the functional interaction between H2S and NO in the induction of stomatal closure by most hormones (with the exception of ABA) remains to be studied.
In general, it can be stated that hydrogen sulfide, NO, and ROS are involved in a complex functional interaction that can be both synergistic and antagonistic. The latter seems to provide extinction of signals that cause stomatal closure. However, as shown by numerous experimental data, the GTs NO and H2S not only mediate the hormonal regulation of guard cells but can also independently cause the effects of stomatal closure. A third known GT, carbon monoxide, has the same effect on stomata, although its physiological effects are much less studied. Finally, high concentrations of carbon dioxide also cause stomatal closure. Although CO2 is not yet considered a plant GT, data obtained in recent years show that it corresponds to the effects of GTs by many criteria and is closely involved in the signaling network that regulates the stomatal state. Carbon dioxide is associated with components of the ABA signaling pathway and with important mediators, such as ROS and GT NO. At the same time, elevated CO2 concentrations may not only contribute to the optimization of the water balance by regulating not only the state of stomata but also the development of the stomatal apparatus but may also activate other defense systems, in particular the antioxidant system [29]. In this context, the use of exogenous CO2 can be an additional tool to regulate not only the photosynthetic productivity of plants but also their stress resistance.
The most important mechanisms of action of most GT are the effects of protein PTMs, which appear to belong to the same universal mechanisms of regulation of protein functional activity as phosphorylation [163]. Since the main targets of NO and H2S action in protein molecules are thiol groups, GT-induced PTMs are closely related to another universal cellular regulatory process, redox regulation due to the conversion of thiol and sulfhydryl groups. All of these mechanisms are involved in the regulation of stomatal status. However, it should be recognized that despite the intensive accumulation of knowledge in this field, the understanding of the principle of operation of such an “orchestra” of regulatory mechanisms has not yet been achieved.
Thus, there is no doubt that three major GTs (NO, H2S, and CO), as well as CO2, are capable of influencing the stomatal state. However, most experimental data on the effects of these gaseous molecules have been obtained in short-term model experiments using isolated epidermis or cut leaves incubated in GT donor solutions. Such experiments likely provide evidence for the potential mechanisms of interaction between signaling mediators in the regulation of the state of the stomatal apparatus. Nevertheless, it is unlikely that these data can be extrapolated to the long-term effects of compounds involved in stomatal regulation in intact plants. It is sufficient to cite the phenomenon described in this review, namely, the change of sign in the effect of hydrogen sulfide on the condition of stomata depending on the time of incubation of the epidermis in the medium containing it [63,171]. In light of these considerations, it seems implausible that the state of stomata can be regulated by the direct treatment of intact plants with relevant plant hormones, GTs, or other signaling molecules. Although it is conceivable that GT donors and other exogenous compounds may be effective for certain practical tasks, such as preserving the freshness of cut flowers [229], which is highly dependent on the condition of the stomata [230], this remains to be seen.
Nevertheless, the positive (though largely empirical) experience of utilizing exogenous plant hormones and GTs to enhance plant resilience to drought and other abiotic stressors suggests their capacity to induce stress-protective responses, including long-term responses. Detailed examination of the mechanisms underlying these reactions is beyond the scope of this review. It can only be postulated that the efficacy of exogenous GTs may be attributed to their capacity to not only modulate the actions of plant hormones but also to stimulate the synthesis of diverse classes of plant hormones themselves [194,231,232]. Additionally, they are involved in a multitude of non-stomatal stress-protective responses. It is sufficient to mention the activation of antioxidant and osmoprotective systems under their influence, as demonstrated in experiments on whole plants under conditions approximating natural conditions [23].
Despite the aforementioned limitations, the study of the mechanisms through which GTs influence the state of stomata (links between intermediates) in model systems may prove to be a promising avenue of research. This is particularly the case for GTs for which insufficient information has been obtained thus far (e.g., carbon monoxide), as well as for those GTs whose effects on stomata, in general, remain unexplored even at the phenomenological level (methane and hydrogen). On the other hand, it is important to study the action of GTs on more complex systems, particularly their complex effects on the stomatal apparatus of intact plants under conditions as close as possible to natural ones.
Acknowledgement: None.
Funding Statement: This work was supported by Projects 14.00.02.06.P “Development of Methods of Seed Priming of Cereal Grains by the Action of Donors of Gasotransmitters and Compounds with Hormonal Activity” (state registration number of work 0124U000126) and III-2-23 “Genetic and Epigenetic Mechanisms and Factors of Protective and Adaptive Reactions of Plants” (state registration number of work 0123U101054).
Author Contributions: The authors confirm contribution to the paper as follows: conceptualization: Yuriy E. Kolupaev, Alexander P. Dmitriev; draft manuscript preparation: Yuriy E. Kolupaev, Tetiana O. Yastreb; illustration and editing: Tetiana O. Yastreb, Alexander P. Dmitriev. All authors reviewed the results and approved the final version of the manuscript.
Availability of Data and Materials: Data sharing does not apply to this article.
Ethics Approval: Not applicable.
Conflicts of Interest: The authors declare no conflicts of interest to report regarding the present study.
References
1. Scuffi D, Lamattina L, García-Mata C. Decoding the interaction between nitric oxide and hydrogen sulfide in stomatal movement. In: Lamattina L, García-Mata C, editors. Gasotransmitters in plants. Cham: Springer; 2016. p. 271–87. doi:10.1007/978-3-319-40713-5_13. [Google Scholar] [CrossRef]
2. Hasanuzzaman MD, Zhou M, Shabala S. How does stomatal density and residual transpiration contribute to osmotic stress tolerance? Plants. 2023;12(3):494. doi:10.3390/plants12030494. [Google Scholar] [PubMed] [CrossRef]
3. Kollist H, Zandalinas SI, Sengupta S, Nuhkat M, Kangasjarvi J, Mittler R. Rapid responses to abiotic stress: priming the landscape for the signal transduction network. Trends Plant Sci. 2019;24(1):25–37. doi:10.1016/j.tplants.2018.10.003. [Google Scholar] [PubMed] [CrossRef]
4. Liu H, Xue S. Interplay between hydrogen sulfide and other signaling molecules in the regulation of guard cell signaling and abiotic/biotic stress response. Plant Commun. 2021;2(3):100179. doi:10.1016/j.xplc.2021.100179. [Google Scholar] [PubMed] [CrossRef]
5. Wu J, Liu Y. Stomata-pathogen interactions: over a century of research. Trends Plant Sci. 2022;27(10):964–67. doi:10.1016/j.tplants.2022.07.004. [Google Scholar] [PubMed] [CrossRef]
6. Wang M, Ji Q, Liu P, Liu Y. Guarding and hijacking: stomata on the move. Trends Plant Sci. 2022;27(8):736–8. doi:10.1016/j.tplants.2022.05.004. [Google Scholar] [PubMed] [CrossRef]
7. Pantaleno R, Scuffi D, Garcia-Mata C. Hydrogen sulphide as a guard cell network regulator. New Phytol. 2021;230(2):451–6. doi:10.1111/nph.17113. [Google Scholar] [PubMed] [CrossRef]
8. Gahir S, Bharath P, Raghavendra S. The role of gasotransmitters in movement of stomata: mechanisms of action and importance for plant immunity. Biol Plant. 2020;64:623–32. doi:10.32615/bp.2020.071. [Google Scholar] [CrossRef]
9. Roelfsema MRG, Hedrich R. In the light of stomatal opening: new insights into ‘The Watergate’. New Phytol. 2005;167(3):665–91. doi:10.1111/j.1469-8137.2005.01460.x. [Google Scholar] [PubMed] [CrossRef]
10. Wani KI, Naeem M, Castroverde CDM, Kalaji HM, Albaqami M, Aftab T. Molecular mechanisms of nitric oxide (NO) signaling and reactive oxygen species (ROS) homeostasis during abiotic stresses in plants. Int J Mol Sci. 2021;22(17):9656. doi:10.3390/ijms22179656. [Google Scholar] [PubMed] [CrossRef]
11. Meddya S, Meshram S, Sarkar DSR, Datta R, Singh S, Avinash G, et al. Plant stomata: an unrealized possibility in plant defense against invading pathogens and stress tolerance. Plants. 2023;12(19):3380. doi:10.3390/plants12193380. [Google Scholar] [PubMed] [CrossRef]
12. Nguyen TBA, Lefoulon C, Nguyen TH, Blatt MR, Carroll W. Engineering stomata for enhanced carbon capture and water-use efficiency. Trends Plant Sci. 2023;28(11):1290–309. doi:10.1016/j.tplants.2023.06.002. [Google Scholar] [PubMed] [CrossRef]
13. Agurla S, Gayatri G, Raghavendra AS. Signal transduction components in guard cells during stomatal closure by plant hormones and microbial elicitors. In: Pandey GK, editor. Mechanism of plant hormone signaling under stress. New Jersey: Wiley; 2017. vol. 2, p. 353–87. doi:10.1002/9781118889022.ch30. [Google Scholar] [CrossRef]
14. Kashtoh H, Baek KH. Structural and functional insights into the role of guard cell ion channels in abiotic stress-induced stomatal closure. Plants. 2021;10(12):2774. doi:10.3390/plants10122774. [Google Scholar] [PubMed] [CrossRef]
15. Li Y, Ding Y, Qu L, Li X, Lai Q, Zhao P, et al. Structure of the Arabidopsis guard cell anion channel SLAC1 suggests activation mechanism by phosphorylation. Nature Commun. 2022;13(1):2511. doi:10.1038/s41467-022-30253-3. [Google Scholar] [PubMed] [CrossRef]
16. Askari-Khorsgani O, Flores FB, Pessarakli M. Plant signaling pathways involved in stomatal movement under drought stress conditions. Adv Plants Agric Res. 2018;8(3):290–7. doi:10.15406/apar.2018.08.00329. [Google Scholar] [CrossRef]
17. Kolbert Z, Feigl G, Freschi L, Poór P. Gasotransmitters in action: nitric oxide-ethylene crosstalk during plant growth and abiotic stress responses. Antioxidants. 2019;8(6):167. doi:10.3390/antiox8060167. [Google Scholar] [PubMed] [CrossRef]
18. Xu B, Feng X, Piechatzek A, Zhang S, Konrad KR, Kromdijk J, et al. The GABA shunt contributes to ROS homeostasis in guard cells of Arabidopsis. New Phytol. 2024;241(1):73–81. doi:10.1111/nph.19390. [Google Scholar] [PubMed] [CrossRef]
19. Wang Z, Li L, Khan D, Chen Y, Pu X, Wang X, et al. Nitric oxide acts downstream of reactive oxygen species in phytomelatonin receptor 1 (PMTR1)-mediated stomatal closure in Arabidopsis. J Plant Physiol. 2023;282(2):153917. doi:10.1016/j.jplph.2023.153917. [Google Scholar] [PubMed] [CrossRef]
20. Feng D, Liu W, Chen K, Ning S, Gao Q, Chen J, et al. Exogenous substances used to relieve plants from drought stress and their associated underlying mechanisms. Int J Mol Sci. 2024;25(17):9249. doi:10.3390/ijms25179249. [Google Scholar] [PubMed] [CrossRef]
21. Jensen NB, Ottosen CO, Fomsgaard IS, Zhou R. Elevated CO2 induce alterations in the hormonal regulation of stomata in drought stressed tomato seedlings. Plant Physiol Biochem. 2024;212:108762. doi:10.1016/j.plaphy.2024.108762. [Google Scholar] [PubMed] [CrossRef]
22. Dey P, Pattanaik D, Mohapatra D, Saha D, Dash D, Mishra A, et al. Gasotransmitters signaling and their crosstalk with other signaling molecules under diverse stress conditions in plants. South Afr J Bot. 2024;169(1):119–33. doi:10.1016/j.sajb.2024.03.041. [Google Scholar] [CrossRef]
23. Kolupaev YE, Karpets YV, Shkliarevskyi MA, Yastreb TO, Plohovska SH, Yemets АI, et al. Gasotransmitters in plants: mechanisms of participation in adaptive responses. Open Agricult J. 2022;16:e187433152207050. doi:10.2174/18743315-v16-e2207050. [Google Scholar] [CrossRef]
24. Scuffi D, Lamattina L, García-Mata C. Gasotransmitters and stomatal closure: is there redundancy, concerted action, or both? Front Plant Sci. 2016;7(104):277. doi:10.3389/fpls.2016.00277. [Google Scholar] [PubMed] [CrossRef]
25. Chen S, Jia H, Wang X, Shi C, Wang X, Ma P, et al. Hydrogen sulfide positively regulates abscisic acid signaling through persulfidation of SnRK2.6 in guard cells. Mol Plant. 2020;13(5):732–44. doi:10.1016/j.molp.2020.01.004. [Google Scholar] [PubMed] [CrossRef]
26. Wong A, Hu N, Tian X, Yang Y, Gehring C. Nitric oxide sensing revisited. Trends Plant Sci. 2021;26(9):885–97. doi:10.1016/j.tplants.2021.009. [Google Scholar] [CrossRef]
27. Raza A, Tabassum J, Mubarik MS, Anwar S, Zahra N, Sharif Y, et al. Hydrogen sulfide: an emerging component against abiotic stress in plants. Plant Biol. 2022;24(4):540–58. doi:10.1111/plb.13368. [Google Scholar] [PubMed] [CrossRef]
28. Engineer CB, Hashimoto-Sugimoto M, Negi J, Israelsson-Nordström M, Azoulay-Shemer T, Rappel WJ, et al. CO2 sensing and CO2 regulation of stomatal conductance: advances and open questions. Trends Plant Sci. 2016;21(1):16–30. doi:10.1016/j.tplants.2015.08.014. [Google Scholar] [PubMed] [CrossRef]
29. Verma N, Prasad SM. Gasotransmitters signaling in plants under abiotic stress: an overview. In: Fatma M, Sehar Z, Khan NA, editors. Gasotransmitters signaling in plant abiotic stress. Cham: Springer; 2023. p. 1–16. doi:10.1007/978-3-031-30858-1_1. [Google Scholar] [CrossRef]
30. Kabange NR, Mun BG, Lee SM, Kwon Y, Lee D, Lee GM, et al. Nitric oxide: a core signaling molecule under elevated GHGs (CO2, CH4, N2O, O3)-mediated abiotic stress in plants. Front Plant Sci. 2022;13:994149. doi:10.3389/fpls.2022.994149. [Google Scholar] [PubMed] [CrossRef]
31. Kumari R, Kapoor P, Mir BA, Singh M, Parrey ZA, Rakhra G, et al. Unlocking the versatility of nitric oxide in plants and insights into its molecular interplays under biotic and abiotic stress. Nitric Oxide. 2024;150(9):1–17. doi:10.1016/j.niox.2024.07.002. [Google Scholar] [PubMed] [CrossRef]
32. Khator K, Parihar S, Jasik J, Shekhawat GS. Nitric oxide in plants: an insight on redox activity and responses toward abiotic stress signaling. Plant Signal Behav. 2024;19(1):2298053. doi:10.1080/15592324.2023.22980. [Google Scholar] [CrossRef]
33. Verma N, Tiwari S, Singh VP, Mohan PS. Nitric oxide in plants: an ancient molecule with new tasks. Plant Growth Regul. 2020;90(1):1–13. doi:10.1007/s10725-019-00543-w. [Google Scholar] [CrossRef]
34. Hancock JT, Neill SJ. Nitric oxide: its generation and interactions with other reactive signaling compounds. Plants. 2019;8(2):41. doi:10.3390/plants8020041. [Google Scholar] [PubMed] [CrossRef]
35. Jeandroz S, Wipf D, Stuehr DJ, Lamattina L, Melkonian M, Tian Z, et al. Occurrence, structure, and evolution of nitric oxide synthase-like proteins in the plant kingdom. Sci Signal. 2016;9(417):re2. doi:10.1126/scisignal.aad4403. [Google Scholar] [PubMed] [CrossRef]
36. Gupta KJ, Kolbert Z, Durner J, Lindermayr C, Corpas FJ, Brouquisse R, et al. Regulating the regulator: nitric oxide control of post-translational modifications. New Phytol. 2020;227(5):1319–25. doi:10.1111/nph.16622. [Google Scholar] [PubMed] [CrossRef]
37. Mishra V, Singh P, Tripathi DK, Corpas FJ, Singh VP. Nitric oxide and hydrogen sulfide: an indispensable combination for plant functioning. Trends Plant Sci. 2021;26(12):1270–85. doi:10.1016/j.tplants.2021.07.016. [Google Scholar] [PubMed] [CrossRef]
38. Farnese FS, Menezes-Silva PE, Gusman GS, Oliveira JA. When bad guys become good ones: the key role of reactive oxygen species and nitric oxide in the plant responses to abiotic stress. Front Plant Sci. 2016;7(273):471. doi:10.3389/fpls.2016.00471. [Google Scholar] [PubMed] [CrossRef]
39. Saha J, Brauer EK, Sengupta A, Popescu SC, Gupta K, Gupta B. Polyamines as redox homeostasis regulators during salt stress in plants. Front Environ Sci. 2015;3(85):21. doi:10.3389/fenvs.2015.00021. [Google Scholar] [CrossRef]
40. Gupta KJ, Kaladhar VC, Fitzpatrick TB, Fernie AR, Møller IM, Loake GJ. Nitric oxide regulation of plant metabolism. Mol Plant. 2022;15(2):228–42. doi:10.1016/j.molp.2021.12.012. [Google Scholar] [PubMed] [CrossRef]
41. López-Gómez P, Buezo J, Urra M, Cornejo A, Esteban R, de Los Reyes JF, et al. A new oxidative pathway of nitric oxide production from oximes in plants. Mol Plant. 2024;17(1):178–98. doi:10.1016/j.molp.2023.12.009. [Google Scholar] [PubMed] [CrossRef]
42. Lindermayr C. Crosstalk between reactive oxygen species and nitric oxide in plants: key role of S-nitrosoglutathione reductase. Free Radical Biol Med. 2018;122:110–5. doi:10.1016/j.freeradbiomed.2017.11.027. [Google Scholar] [PubMed] [CrossRef]
43. Jahnová J, Luhová L, Petřivalský M. S-nitrosoglutathione reductase—the master regulator of protein S-nitrosation in plant NO signaling. Plants. 2019;8(2):48. doi:10.3390/plants8020048. [Google Scholar] [PubMed] [CrossRef]
44. Chaki M, Valderrama R, Fernández-Ocaña AM, Carreras A, Gómez-Rodríguez MV, Pedrajas JR, et al. Mechanical wounding induces a nitrosative stress by down-regulation of GSNO reductase and an increase in S-nitrosothiols in sunflower (Helianthus annuus) seedlings. J Exp Bot. 2011;62(6):1803–13. doi:10.1093/jxb/erq358. [Google Scholar] [PubMed] [CrossRef]
45. Lee U, Wie C, Fernandez BO, Feelisch M, Vierling E. Modulation of nitrosative stress by S-nitrosoglutathione reductase is critical for thermotolerance and plant growth in Arabidopsis. Plant Cell. 2008;20(3):786–802. doi:10.1105/tpc.107.052647. [Google Scholar] [PubMed] [CrossRef]
46. Montilla-Bascón G, Rubiales D, Hebelstrup KH, Mandon J, Harren FJM, Cristescu SM, et al. Reduced nitric oxide levels during drought stress promote drought tolerance in barley and is associated with elevated polyamine biosynthesis. Sci Rep. 2017;7(1):13311. doi:10.1038/s41598-017-13458-1. [Google Scholar] [PubMed] [CrossRef]
47. Bari SE, Olabe JA. New features of the NO/H2S cross talk: a chemical basis. In: Lamattina L, García-Mata C, editors. Gasotransmitters in plants. Cham: Springer; 2016. p. 289–327. doi:10.1007/978-3-319-40713-5_14. [Google Scholar] [CrossRef]
48. Luo F, Zhang Y, Zhang S, Ji Y, Yan D, Lai M, et al. Rational design of Near-Infrared fluorescent probe for monitoring HNO in plants. Spectrochim Acta A. 2024;321:124672. doi:10.1016/j.saa.2024.124672. [Google Scholar] [PubMed] [CrossRef]
49. Arora D, Jain P, Singh N, Kaur H, Bhatla SC. Mechanisms of nitric oxide crosstalk with reactive oxygen species scavenging enzymes during abiotic stress tolerance in plants. Free Radic Res. 2016;50(3):291–303. doi:10.3109/10715762.2015.1118473. [Google Scholar] [PubMed] [CrossRef]
50. Lamotte O, Bertoldo JB, Besson-Bard A, Rosnoblet C, Aimé S, Hichami S, et al. Protein S-nitrosylation: specificity and identification strategies in plants. Front Chem. 2015;2(137):114. doi:10.3389/fchem.2014.00114. [Google Scholar] [PubMed] [CrossRef]
51. Hu J, Huang X, Chen L, Sun X, Lu C, Zhang L, et al. Site-specific nitrosoproteomic identification of endogenously S-nitrosylated proteins in Arabidopsis. Plant Physiol. 2015;167(4):1731–46. doi:10.1104/pp.15.00026. [Google Scholar] [PubMed] [CrossRef]
52. Wei L, Zhang J, Wei S, Wang C, Deng Y, Hu D, et al. Nitric oxide alleviates salt stress through protein S-nitrosylation and transcriptional regulation in tomato seedlings. Planta. 2022;256(6):101. doi:10.1007/s00425-022-04015-w. [Google Scholar] [PubMed] [CrossRef]
53. Sánchez-Vicente I, Fernández-Espinosa MG, Lorenzo O. Nitric oxide molecular targets: reprogramming plant development upon stress. J Exp Bot. 2019;70(17):4441–60. doi:10.1093/jxb/erz339. [Google Scholar] [PubMed] [CrossRef]
54. Corpas FJ, Barroso JB. Nitro-oxidative stress vs oxidative or nitrosative stress in higher plants. New Phytol. 2013;199(3):633–5. doi:10.1111/nph.12380. [Google Scholar] [PubMed] [CrossRef]
55. Valderrama R, Begara-Morales JC, Chaki M, Mata-Pérez C, Padilla MN, Barroso JB. Hydrogen peroxide (H2O2)- and nitric oxide (NO)-derived posttranslational modifications. In: Gupta D, Palma J, Corpas F, editors. Nitric oxide and hydrogen peroxide signaling in higher plants. Cham: Springer; 2019. p. 37–67. doi:10.1007/978-3-030-11129-8_3. [Google Scholar] [CrossRef]
56. Xuan L, Li J, Wang X, Wang C. Crosstalk between hydrogen sulfide and other signal molecules regulates plant growth and development. Int J Mol Sci. 2020;21(13):4593. doi:10.3390/ijms21134593. [Google Scholar] [PubMed] [CrossRef]
57. Gautam H, Khan S, Nidhi, Sofo A, Khan NA. Appraisal of the role of gaseous signaling molecules in thermo-tolerance mechanisms in plants. Plants. 2024;13(6):791. doi:10.3390/plants13060791. [Google Scholar] [PubMed] [CrossRef]
58. Lamotte O, Courtois C, Dobrowolska G, Besson A, Pugin A, Wendehenne D. Mechanisms of nitric-oxide-induced increase of free cytosolic Ca2+ concentration in Nicotiana plumbaginifolia cells. Free Radic Biol Med. 2006;40(8):1369–76. doi:10.1016/j.freeradbiomed.2005.12.006. [Google Scholar] [PubMed] [CrossRef]
59. Lau S-E, Hamdan MF, Pua T-L, Saidi NB, Tan BC. Plant nitric oxide signaling under drought stress. Plants. 2021;10(2):360. doi:10.3390/plants10020360. [Google Scholar] [PubMed] [CrossRef]
60. Hancock JT, Veal D. Nitric oxide, other reactive signalling compounds, redox, and reductive stress. J Exp Bot. 2021;72(3):819–29. doi:10.1093/jxb/eraa331. [Google Scholar] [PubMed] [CrossRef]
61. Singh G, Patel A, Tiwari S, Gupta D, Prasad SM. Signaling molecules hydrogen sulfide (H2S) and nitric oxide (NOrole in microalgae under adverse environmental conditions. Acta Physiol Plant. 2022;44(7):68. doi:10.1007/s11738-022-03404-8. [Google Scholar] [CrossRef]
62. Mulaudzi T, Ludidi N, Ruzvidzo O, Morse M, Hendricks N, Iwuoha E, et al. Identification of a novel Arabidopsis thaliana nitric oxide-binding molecule with guanylate cyclase activity in vitro. FEBS Lett. 2022;585(17):2693–7. doi:10.1016/j.febslet.2011.07.023. [Google Scholar] [PubMed] [CrossRef]
63. Honda K, Yamada N, Yoshida R, Ihara H, Sawa T, Akaike T, et al. 8-mercapto-cyclic GMP mediates hydrogen sulfide-induced stomatal closure in Arabidopsis. Plant Cell Physiol. 2015;56(8):1481–89. doi:10.1093/pcp/pcv069. [Google Scholar] [PubMed] [CrossRef]
64. Liu Y, Zhang H. Reactive oxygen species and nitric oxide as mediators in plant hypersensitive response and stomatal closure. Plant Signal Behav. 2021;16(12):1985860. doi:10.1080/15592324.2021.1985860. [Google Scholar] [PubMed] [CrossRef]
65. Yun BW, Feechan A, Yin M, Saidi NB, Le Bihan T, Yu M, et al. S-nitrosylation of NADPH oxidase regulates cell death in plant immunity. Nature. 2011;478(7368):264–8. doi:10.1038/nature10427. [Google Scholar] [PubMed] [CrossRef]
66. Ortega-Galisteo AP, Rodríguez-Serrano M, Pazmiño DM, Gupta DK, Sandalio LM, Romero-Puertas MC. S-Nitrosylated proteins in pea (Pisum sativum L.) leaf peroxisomes: changes under abiotic stress. J Exp Bot. 2012;63(5):2089–103. doi:10.1093/jxb/err414. [Google Scholar] [PubMed] [CrossRef]
67. Begara-Morales JC, Sánchez-Calvo B, Chaki M, Valderrama R, Mata-Pérez C, LópezJaramillo J, et al. Dual regulation of cytosolic ascorbate peroxidase (APX) by tyrosine nitration and S-nitrosylation. J Exp Bot. 2014;65(2):527–38. doi:10.1093/jxb/ert396. [Google Scholar] [PubMed] [CrossRef]
68. Rodríguez-Ruiz M, González-Gordo S, Cañas A, Campos MJ, Paradela A, Corpas FJ, et al. Sweet pepper (Capsicum annuum L.) fruits contain an atypical peroxisomal catalase that is modulated by reactive oxygen and nitrogen species. Antioxidants. 2019;8(9):374. doi:10.3390/antiox8090374. [Google Scholar] [PubMed] [CrossRef]
69. Dubovskaya LV, Bakakina YS, Kolesneva EV, Sodel DL, McAinsh MR, Hetherington AM, et al. cGMP-dependent ABA-induced stomatal closure in the ABA-insensitive Arabidopsis mutant abi1-1. New Phytol. 2011;191(1):57–69. doi:10.1111/j.1469-8137.2011.03661.x. [Google Scholar] [PubMed] [CrossRef]
70. Corpas FJ, González-Gordo S, Palma JM. Nitric oxide and hydrogen sulfide modulate the NADPH-generating enzymatic system in higher plants. J Exp Bot. 2021;72(3):830–47. doi:10.1093/jxb/eraa440. [Google Scholar] [PubMed] [CrossRef]
71. Sun Y-Y, Wang J-Q, Xiang R-H, Li Z-G. Key role of reactive oxygen species-scavenging system in nitric oxide and hydrogen sulfide crosstalk-evoked thermotolerance in maize seedlings. Front Plant Sci. 2022;13:967968. doi:10.3389/fpls.2022.967968. [Google Scholar] [PubMed] [CrossRef]
72. Zhou H, Zhou Y, Zhang F, Guan W, Su Y, Yuan X, et al. Persulfidation of nitrate reductase 2 is involved in L-cysteine desulfhydrase-regulated rice drought tolerance. Int J Mol Sci. 2021;22(22):12119. doi:10.3390/ijms222212119. [Google Scholar] [PubMed] [CrossRef]
73. Wang Y, Li L, Cui W, Xu S, Shen W, Wang R. Hydrogen sulfide enhances alfalfa (Medicago sativa) tolerance against salinity during seed germination by nitric oxide pathway. Plant Soil. 2012;351(1–2):107–19. doi:10.1007/s11104-011-0936-2. [Google Scholar] [CrossRef]
74. Karpets YV, Kolupaev YE, Lugovaya AA, Shvidenko NV, Shkliarevskyi MA, Yastreb TO. Functional interaction of ROS and nitric oxide during induction of heat resistance of wheat seedlings by hydrogen sulfide donor. Russ J Plant Physiol. 2020;67(4):653–60. doi:10.1134/S1021443720030140. [Google Scholar] [CrossRef]
75. Desikan R, Griffiths R, Hancock J, Neill S. A new role for an old enzyme: nitrate reductase-mediated nitric oxide generation is required for abscisic acid induced stomatal closure in Arabidopsis thaliana. Proc Natl Acad Sci U S A. 2002;99(25):16314–18. doi:10.1073/pnas.252461999. [Google Scholar] [PubMed] [CrossRef]
76. Gayatri G, Agurla S, Raghavendra AS. Nitric oxide in guard cells as an important secondary messenger during stomatal closure. Front Plant Sci. 2013;4:425. doi:10.3389/fpls.2013.00425. [Google Scholar] [PubMed] [CrossRef]
77. Yastreb TO, Kolupaev YE, Kokorev AI, Horielova EI, Dmitriev AP. Methyl jasmonate and nitric oxide in regulation of the stomatal apparatus of Arabidopsis thaliana. Cytol Genet. 2018;52(6):400–5. doi:10.3103/S0095452718060129. [Google Scholar] [CrossRef]
78. Wilson ID, Neill SJ, Hancock JT. Nitric oxide synthesis and signalling in plants. Plant Cell Environ. 2008;31(5):622–31. doi:10.1111/j.1365-3040.2007.01761.x. [Google Scholar] [PubMed] [CrossRef]
79. Joudoi T, Shichiri Y, Kamizono N, Akaike T, Sawa T, Yoshitake J, et al. Nitrated cyclic GMP modulates guard cell signaling in Arabidopsis. Plant Cell. 2013;25(2):558–71. doi:10.1105/tpc.112.105049. [Google Scholar] [PubMed] [CrossRef]
80. Mukherjee S, Roy S, Corpas FJ. Aquaporins: a vital nexus in H2O2—gasotransmitter signaling. Trends Plant Sci. 2024;29(6):681–93. doi:10.1016/j.tplants.2023.11.021. [Google Scholar] [PubMed] [CrossRef]
81. Mishra V, Singh P, Kushwaha BK, Tripathi KD, Corpas FJ, Singh VP. HPCA1 and HSL3: two plasma membrane proteins that probably cooperate to modulate H2O2 signalling under drought conditions. Plant Growth Regul. 2022;98(1):1–3. doi:10.1007/s10725-022-00829-6. [Google Scholar] [CrossRef]
82. Xia H, Liu X, Wang Y, Lin Z, Deng H, Wang J, et al. 24-epibrassinolide and nitric oxide combined to improve the drought tolerance in kiwifruit seedlings by proline pathway and nitrogen metabolism. Sci Horticult. 2022;297:110929. doi:10.1016/j.scienta.2022.110929. [Google Scholar] [CrossRef]
83. Lawrence SR2nd, Gaitens M, Guan Q, Dufresne C, Chen S. S-nitroso-proteome revealed in stomatal guard cell response to Flg22. Int J Mol Sci. 2020;21(5):1688. doi:10.3390/ijms21051688. [Google Scholar] [PubMed] [CrossRef]
84. Bharath P, Gahir S, Raghavendra AS. Abscisic acid-induced stomatal closure: an important component of plant defense against abiotic and biotic stress. Front Plant Sci. 2021;12:615114. doi:10.3389/fpls.2021.615114. [Google Scholar] [PubMed] [CrossRef]
85. Niu M, Xie J, Chen C, Cao H, Sun J, Kong Q, et al. An early ABA-induced stomatal closure, Na+ sequestration in leaf vein and K+ retention in mesophyll confer salt tissue tolerance in Cucurbita species. J Exp Bot. 2018;69(20):4945–60. doi:10.1093/jxb/ery251. [Google Scholar] [PubMed] [CrossRef]
86. Maheshwari P, Assmann SM, Albert R. A guard cell abscisic acid (ABA) network model that captures the stomatal resting state. Front Physiol. 2020;11:927. doi:10.3389/fphys.2020.00927. [Google Scholar] [PubMed] [CrossRef]
87. Xu J, Lu X, Liu Y, Lan W, Wei Z, Yu W, et al. Interaction between ABA and NO in plants under abiotic stresses and its regulatory mechanisms. Front Plant Sci. 2024;15:1330948. doi:10.3389/fpls.2024.1330948. [Google Scholar] [PubMed] [CrossRef]
88. Hsu PK, Takahashi Y, Munemasa S, Merilo E, Laanemets K, Waadt R, et al. Abscisic acid-independent stomatal CO2 signal transduction pathway and convergence of CO2 and ABA signaling downstream of OST1 kinase. Proc Natl Acad Sci U S A. 2018;115(42):E9971–80. doi:10.1073/pnas.1809204115. [Google Scholar] [PubMed] [CrossRef]
89. Sirichandra C, Gu D, Hu HC, Davanture M, Lee S, Djaoui M, et al. Phosphorylation of the Arabidopsis AtrbohF NADPH oxidase by OST1 protein kinase. FEBS Lett. 2009;583(18):2982–6. doi:10.1016/j.febslet.2009.08.033. [Google Scholar] [PubMed] [CrossRef]
90. Chen ZH, Wang Y, Wang JW, Babla M, Zhao C, García-Mata C, et al. Nitrate reductase mutation alters potassium nutrition as well as nitric oxide-mediated control of guard cell ion channels in Arabidopsis. New Phytol. 2016;209(4):1456–69. doi:10.1111/nph.13714. [Google Scholar] [PubMed] [CrossRef]
91. Deng YN, Kashtoh H, Wang Q, Zhen GX, Li QY, Tang LH, et al. Structure and activity of SLAC1 channels for stomatal signaling in leaves. Proc Natl Acad Sci U S A. 2021;118(18):e2015151118. doi:10.1073/pnas.2015151118. [Google Scholar] [PubMed] [CrossRef]
92. Agurla S, Gahir S, Munemasa S, Murata Y, Raghavendra AS. Mechanism of stomatal closure in plants exposed to drought and cold stress. Adv Exp Med Biol. 2018;1081(1):215–32. doi:10.1007/978-981-13-1244-1_12. [Google Scholar] [PubMed] [CrossRef]
93. Castillo MC, Lozano-Juste J, González-Guzmán M, Rodriguez L, Rodriguez PL, León J. Inactivation of PYR/PYL/RCAR ABA receptors by tyrosine nitration may enable rapid inhibition of ABA signaling by nitric oxide in plants. Sci Signal. 2015;8(392):ra89. doi:10.1126/scisignal.aaa7981. [Google Scholar] [PubMed] [CrossRef]
94. Corpas FJ. NO and H2S contribute to crop resilience against atmospheric stressors. Int J Mol Sci. 2024;25(6):3509. doi:10.3390/ijms25063509. [Google Scholar] [PubMed] [CrossRef]
95. Wang P, Du Y, Hou Y-J, Zhao Y, Hsu CC, Yuan F, et al. Nitric oxide negatively regulates abscisic acid signaling in guard cells by S-nitrosylation of OST1. Proc Natl Acad Sci U S A. 2015;112(2):613–8. doi:10.1073/pnas.1423481112. [Google Scholar] [PubMed] [CrossRef]
96. Van Meeteren U, Kaiser E, Malcolm Matamoros P, Verdonk JC, Aliniaeifard S. Is nitric oxide a critical key factor in ABA-induced stomatal closure? J Exp Bot. 2020;71(1):399–410. doi:10.1093/jxb/erz437. [Google Scholar] [PubMed] [CrossRef]
97. Sehar Z, Masood A, Khan NA. Nitric oxide reverses glucosemediated photosynthetic repression in wheat (Triticum aestivum L.) under salt stress. Environ Exp Bot. 2019;161(6957):277–89. doi:10.1016/j.envexpbot.2019.01.010. [Google Scholar] [CrossRef]
98. Laxalt AM, García-Mata C, Lamattina L. The dual role of nitric oxide in guard cells: promoting and attenuating the aba and phospholipid-derived signals leading to the stomatal closure. Front Plant Sci. 2016;7(ra89):476. doi:10.3389/fpls.2016.00476. [Google Scholar] [PubMed] [CrossRef]
99. Ali MS, Baek K-H. Jasmonic acid signaling pathway in response to abiotic stresses in plants. Int J Mol Sci. 2020;21(2):621. doi:10.3390/ijms21020621. [Google Scholar] [PubMed] [CrossRef]
100. Jang G, Yoon Y, Choi YD. Crosstalk with jasmonic acid integrates multiple responses in plant development. Int J Mol Sci. 2020;21(1):305. doi:10.3390/ijms21010305. [Google Scholar] [PubMed] [CrossRef]
101. Wang X, Li Q, Xie J, Huang M, Cai J, Zhou Q, et al. Abscisic acid and jasmonic acid are involved in drought priming-induced tolerance to drought in wheat. Crop J. 2021;9(1):120–32. doi:10.1016/j.cj.2020.06.002. [Google Scholar] [CrossRef]
102. Ghorbel M, Brini F, Sharma A, Landi M. Role of jasmonic acid in plants: the molecular point of view. Plant Cell Rep. 2021;40(8):1471–94. doi:10.1007/s00299-021-02687-4. [Google Scholar] [PubMed] [CrossRef]
103. Kolupaev YE, Yastreb TO, Dmitriev AP. Signal mediators in the implementation of jasmonic acid’s protective effect on plants under abiotic stresses. Plants. 2023;12(14):2631. doi:10.3390/plants12142631. [Google Scholar] [PubMed] [CrossRef]
104. Montillet JL, Leonhardt N, Mondy S, Tranchimand S, Rumeau D, Boudsocq M, et al. An abscisic acid-independent oxylipin pathway controls stomatal closure and immune defense in Arabidopsis. PLoS Biol. 2013;11(3):e1001513. doi:10.1371/journal.pbio.1001513. [Google Scholar] [PubMed] [CrossRef]
105. Munemasa S, Mori IC, Murata Y. Methyl jasmonate signaling and signal crosstalk between methyl jasmonate and abscisic acid in guard cells. Plant Signal Behav. 2011;6(7):939–41. doi:10.4161/psb.6.7.15439. [Google Scholar] [PubMed] [CrossRef]
106. Yin Y, Adachi Y, Nakamura Y, Munemasa S, Mori IC, Murata Y. Involvement of OST1 protein kinase and PYR/PYL/RCAR receptors in methyl jasmonate-induced stomatal closure in Arabidopsis guard cells. Plant Cell Physiol. 2016;57(8):1779–90. doi:10.1093/pcp/pcw102. [Google Scholar] [PubMed] [CrossRef]
107. Munemasa S, Oda K, Watanabe-Sugimoto M, Nakamura Y, Shimoishi Y, Murata Y. The coronatine-insensitive 1 mutation reveals the hormonal signaling interaction between abscisic acid and methyl jasmonate in Arabidopsis guard cells. Specific impairment of ion channel activation and second messenger production. Plant Physiol. 2007;143(3):1398–407. doi:10.1104/pp.106.091298. [Google Scholar] [PubMed] [CrossRef]
108. Ye W, Hossain MA, Munemasa S, Nakamura Y, Mori IC, Murata Y. Endogenous abscisic acid is involved in methyl jasmonate-induced reactive oxygen species and nitric oxide production but not in cytosolic alkalization in Arabidopsis guard cells. J Plant Physiol. 2013;170(13):1212–15. doi:10.1016/j.jplph.2013.03.011. [Google Scholar] [PubMed] [CrossRef]
109. Yastreb TO, Kolupaev YE, Lugovaya AA, Dmitriev AP. Formation of adaptive reactions in Arabidopsis thaliana wild-type and mutant jin1 plants under action of abscisic acid and salt stress. Cytol Genet. 2017;51(5):325–30. doi:10.3103/S0095452717050115. [Google Scholar] [CrossRef]
110. Yastreb TO, Kolupaev YE, Karpets YV, Dmitriev AP. Response of stomatal apparatus of Arabidopsis plants defective in jasmonate signaling to abscisic acid and methyl jasmonate action. Visn Hark Nac Agrar Univ Ser Biol. 2017;3(42):72–80. doi:10.35550/vbio2017.03.072. [Google Scholar] [CrossRef]
111. Palmieri MC, Sell S, Huang X, Scherf M, Werner T, Durner J, et al. Nitric oxide-responsive genes and promoters in Arabidopsis thaliana: a bioinformatics approach. J Exp Bot. 2008;59(2):177–86. doi:10.1093/jxb/erm345. [Google Scholar] [PubMed] [CrossRef]
112. Yastreb TO, Kolupaev YE, Shkliarevskyi MA, Dmitriev AP. Participation of jasmonate signaling components in the development of Arabidopsis thaliana’s salt resistance induced by H2S and NO donors. Russ J Plant Physiol. 2020;67(5):827–34. doi:10.1134/S1021443720050192. [Google Scholar] [CrossRef]
113. Samanta S, Roychoudhury A. Molecular crosstalk of jasmonate with major phytohormones and plant growth regulators during diverse stress responses. J Plant Growth Regul. 2024;89(9):1. doi:10.1007/s00344-024-11412-w. [Google Scholar] [CrossRef]
114. Liu X, Shi W, Zhang S, Lou C. Nitric oxide involved in signal transduction of jasmonic acid-induced stomatal closure of Vicia faba L. Chin Sci Bull. 2005;50(6):520–5. doi:10.1360/982004-794. [Google Scholar] [CrossRef]
115. Nazareno AL, Hernandez BS. A mathematical model of the interaction of abscisic acid, ethylene and methyl jasmonate on stomatal closure in plants. PLoS One. 2017;12(2):e0171065. doi:10.1371/journal.pone.0171065. [Google Scholar] [PubMed] [CrossRef]
116. Sangwan S, Shameem N, Yashveer S, Tanwar H, Parray JA, Jatav HS, et al. Role of salicylic acid in combating heat stress in plants: insights into modulation of vital processes. Front Biosci. 2022;27(11):310. doi:10.31083/j.fbl2711310. [Google Scholar] [PubMed] [CrossRef]
117. Gutierrez-Larruscain D, Krüger M, Abeyawardana OAJ, Belz C, Dobrev PI, Vanková R, et al. The high concentrations of abscisic, jasmonic, and salicylic acids produced under long days do not accelerate flowering in Chenopodium ficifolium 459. Plant Sci. 2022;320(15):111279. doi:10.1016/j.plantsci.2022.111279. [Google Scholar] [PubMed] [CrossRef]
118. Sedláková V, Zeljkovic S, Štefelová N, Smtfytfkal P, Hanácek P. Phenylpropanoid content of chickpea seed coats in relation to seed dormancy. Plants. 2023;12(14):2687. doi:10.3390/plants12142687. [Google Scholar] [PubMed] [CrossRef]
119. Song W, Shao H, Zheng A, Zhao L, Xu Y. Advances in roles of salicylic acid in plant tolerance responses to biotic and abiotic stresses. Plants. 2023;12(19):3475. doi:10.3390/plants12193475. [Google Scholar] [PubMed] [CrossRef]
120. Hasanuzzaman M, Nahar K, Bhuiyan TF, Anee TI, Inafuku M, Oku H, et al. Salicylic acid: An all-rounder in regulating abiotic stress responses in plants. In: El-Esawi M, editor. Phytohormones–signaling mechanisms and crosstalk in plant development and stress responses. InTech; 2017. p. 31–75. doi:10.5772/intechopen.68213. [Google Scholar] [CrossRef]
121. Emamverdian A, Ding Y, Mokhberdoran F. The role of salicylic acid and gibberellin signaling in plant responses to abiotic stress with an emphasis on heavy metals. Plant Signal Behav. 2020;15(7):1777372. doi:10.1080/15592324.2020.1777372. [Google Scholar] [PubMed] [CrossRef]
122. Hoque TS, Sohag AAM, Burritt DJ, Hossain MA. Salicylic acid-mediated salt stress tolerance in plants. In: Lone R, Shuab R, Kamili A, editors. Plant phenolics in sustainable agriculture. Singapore: Springer; 2020. p. 1–38. doi:10.1007/978-981-15-4890-1_1. [Google Scholar] [CrossRef]
123. Khan MSS, Islam F, Ye Y, Ashline M, Wang D, Zhao B, et al. The interplay between hydrogen sulfide and phytohormone signaling pathways under challenging environments. Int J Mol Sci. 2022;23(8):4272. doi:10.3390/ijms23084272. [Google Scholar] [PubMed] [CrossRef]
124. Koo YM, Heo AY, Choi HW. Salicylic acid as a safe plant protector and growth regulator. Plant Pathol J. 2020;36(1):1–10. doi:10.5423/PPJ.RW.12.2019.0295. [Google Scholar] [PubMed] [CrossRef]
125. Miura K, Tada Y. Regulation of water, salinity, and cold stress responses by salicylic acid. Front Plant Sci. 2014;5:4. doi:10.3389/fpls.2014.00004. [Google Scholar] [PubMed] [CrossRef]
126. Melotto M, Underwood W, Koczan J, Nomura K, He SY. Plant stomata function in innate immunity against bacterial invasion. Cell. 2006;126(5):969–80. doi:10.1016/j.cell.2006.06.054. [Google Scholar] [PubMed] [CrossRef]
127. Miura K, Okamoto H, Okuma E, Shiba H, Kamada H, Hasegawa PM, et al. SIZ1 deficiency causes reduced stomatal aperture and enhanced drought tolerance via controlling salicylic acid-induced accumulation of reactive oxygen species in Arabidopsis. Plant J. 2013;73(1):91–104. doi:10.1111/tpj.12014. [Google Scholar] [PubMed] [CrossRef]
128. Wang HQ, Sun LP, Wang LX, Fang XW, Li ZQ, Zhang FF, et al. Ethylene mediates salicylic-acid-induced stomatal closure by controlling reactive oxygen species and nitric oxide production in Arabidopsis. Plant Sci. 2020;294:110464. doi:10.1016/j.plantsci.2020.110464. [Google Scholar] [PubMed] [CrossRef]
129. Hao F, Zhao S, Dong H, Zhang H, Sun L, Miao C. Nia1 and Nia2 are involved in exogenous salicylic acid-induced nitric oxide generation and stomatal closure in Arabidopsis. J Integr Plant Biol. 2010;52(3):298–307. doi:10.1111/j.1744-7909.2010.00920.x. [Google Scholar] [PubMed] [CrossRef]
130. Sun LR, Hao FS, Lu BS, Ma LY. AtNOA1 modulates nitric oxide accumulation and stomatal closure induced by salicylic acid in Arabidopsis. Plant Signal Behav. 2010;5(8):1022–4. doi:10.4161/psb.5.8.12293. [Google Scholar] [PubMed] [CrossRef]
131. Prodhan MY, Munemasa S, Nahar MNEN, Nakamura Y, Murata Y. Guard cell salicylic acid signaling is integrated into abscisic acid signaling via the Ca2+/CPK-dependent pathway. Plant Physiol. 2018;178(1):441–50. doi:10.1104/pp.18.00321. [Google Scholar] [PubMed] [CrossRef]
132. Agurla S, Sunitha V, Raghavendra AS. Methyl salicylate is the most effective natural salicylic acid ester to close stomata while raising reactive oxygen species and nitric oxide in Arabidopsis guard cells. Plant Physiol Biochem. 2020;157:276–83. doi:10.1016/j.plaphy.2020.10.026. [Google Scholar] [PubMed] [CrossRef]
133. Chen D, Shao Q, Yin L, Younis A, Zheng B. Polyamine function in plants: metabolism, regulation on development, and roles in abiotic stress responses. Front Plant Sci. 2019;9:1945. doi:10.3389/fpls.2018.01945. [Google Scholar] [PubMed] [CrossRef]
134. Khan AS, Singh Z, Abbasi NA, Swinny EE. Pre-or post-harvest application of putrescine and low temperature storage affect fruit ripening and quality of ‘Angelino’ plum. J Sci Food Agric. 2008;88(10):1686–95. doi:10.1002/jsfa.3265. [Google Scholar] [CrossRef]
135. Abbasi NA, Ali I, Hafiz IA, Khan AS. Application of polyamines in horticulture: a review. Int J Biosci. 2017;10(5):319–42. doi:10.12692/ijb/10.5.319-342. [Google Scholar] [CrossRef]
136. Shao J, Huang K, Batool M, Idrees F, Afzal R, Haroon M, et al. Versatile roles of polyamines in improving abiotic stress tolerance of plants. Front Plant Sci. 2022;13:1003155. doi:10.3389/fpls.2022.1003155. [Google Scholar] [PubMed] [CrossRef]
137. Wimalasekera R, Villar C, Begum T, Scherer GFE. COPPER AMINE OXIDASE 1 (CuAO1) of Arabidopsis thaliana contributes to abscisic acid- and polyamine-induced nitric oxide biosynthesis and abscisic acid signal transduction. Mol Plant. 2011;4(4):663–78. doi:10.1093/mp/ssr023. [Google Scholar] [PubMed] [CrossRef]
138. Wimalasekera R, Tebartz F, Scherer GF. Polyamines, polyamine oxidases and nitric oxide in development, abiotic and biotic stresses. Plant Sci. 2011;181(5):593–603. doi:10.1016/j.plantsci.2011.04.002. [Google Scholar] [PubMed] [CrossRef]
139. Fraudentali I, Rodrigues-Pousada RA, Angelini R, Ghuge SA, Cona A. Plant copper amine oxidases: key players in hormone signaling leading to stress-induced phenotypic plasticity. Int J Mol Sci. 2021;22(10):5136. doi:10.3390/ijms22105136. [Google Scholar] [PubMed] [CrossRef]
140. Agurla S, Gayatri G, Raghavendra AS. Polyamines increase nitric oxide and reactive oxygen species in guard cells of Arabidopsis thaliana during stomatal closure. Protoplasma. 2018;255(1):153–62. doi:10.1007/s00709-017-1139-3. [Google Scholar] [PubMed] [CrossRef]
141. Kokorev AI, Kolupaev YE, Yastreb TO, Horielova EI, Dmitriev AP. Realization of polyamines’ effect on the state of pea stomata with the involvement of calcium and components of lipid signaling. Cytol Genet. 2021;55(2):117–24. doi:10.3103/S0095452721020079. [Google Scholar] [CrossRef]
142. Adamipour N, Khosh-Khui M, Salehi H, Razi H, Karami A, Moghadam A. Regulation of stomatal aperture in response to drought stress mediating with polyamines, nitric oxide synthase and hydrogen peroxide in Rosa canina L. Plant Signal Behav. 2020;15(9):1790844. doi:10.1080/15592324.2020.1790844. [Google Scholar] [PubMed] [CrossRef]
143. Arnao MB, Cano A, Hernández-Ruiz J. Phytomelatonin: an unexpected molecule with amazing performances in plants. J Exp Bot. 2022;73(17):5779–800. doi:10.1093/jxb/erac009. [Google Scholar] [PubMed] [CrossRef]
144. Nawaz K, Chaudhary R, Sarwar A, Ahmad B, Gul A, Hano C, et al. Melatonin as master regulator in plant growth, development and stress alleviator for sustainable agricultural production: current status and future perspectives. Sustainability. 2021;13(1):294. doi:10.3390/su13010294. [Google Scholar] [CrossRef]
145. Zeng W, Mostafa S, Lu Z, Jin B. Melatonin-mediated abiotic stress tolerance in plants. Front Plant Sci. 2022;13:847175. doi:10.3389/fpls.2022.847175. [Google Scholar] [PubMed] [CrossRef]
146. Altaf MA, Shahid R, Ren MX, Mora-Poblete F, Arnao MB, Naz S, et al. Phytomelatonin: an overview of the importance and mediating functions of melatonin against environmental stresses. Physiol Plant. 2021;172(2):820–46. doi:10.1111/ppl.13262. [Google Scholar] [PubMed] [CrossRef]
147. Yang X, Ren J, Li J, Lin X, Xia X, Yan W, et al. Meta-analysis of the effect of melatonin application on abiotic stress tolerance in plants. Plant Biotechnol Rep. 2023;17(2):39–52. doi:10.1007/s11816-022-00770-0. [Google Scholar] [CrossRef]
148. Colombage R, Singh MB, Bhalla PL. Melatonin and abiotic stress tolerance in crop plants. Int J Mol Sci. 2023;24(8):7447. doi:10.3390/ijms24087447. [Google Scholar] [PubMed] [CrossRef]
149. Huang J, Xie Y. Hydrogen sulfide signaling in plants. Antioxidants Redox Signal. 2023;39(1–3):40–58. doi:10.1089/ars.2023.0267. [Google Scholar] [PubMed] [CrossRef]
150. Kabil O, Banerjee R. Redox biochemistry of hydrogen sulfide. J Biol Chem. 2010;285(29):21903–7. doi:10.1074/jbc.R110.128363. [Google Scholar] [PubMed] [CrossRef]
151. Yang Z, Wang X, Feng J, Zhu S. Biological functions of hydrogen sulfide in plants. Int J Mol Sci. 2022;23(23):15107. doi:10.3390/ijms232315107. [Google Scholar] [PubMed] [CrossRef]
152. Dai J, Wen D, Li H, Yang J, Rao X, Yang Y, et al. Effect of hydrogen sulfide (H2S) on the growth and development of tobacco seedlings in absence of stress. BMC Plant Biol. 2024;24(1):162. doi:10.1186/s12870-024-04819-w. [Google Scholar] [PubMed] [CrossRef]
153. Li H, Li M, Wei X, Zhang X, Xue R, Zhao Y, et al. Transcriptome analysis of drought-responsive genes regulated by hydrogen sulfide in wheat (Triticum aestivum L.) leaves. Mol Genet Genom. 2017;292(5):1091–110. doi:10.1007/s00438-017-1330-4. [Google Scholar] [PubMed] [CrossRef]
154. Pandey AK, Borokotoky S, Tripathi K, Gautam A. Interplay of hydrogen sulfide and plant metabolites under environmental stress. In: Modolo LV, Da-Silva CJ, editors. H2S in plants. Elsevier Inc.; 2024. p. 297–317. doi:10.1016/B978-0-323-99035-6.00004-X. [Google Scholar] [CrossRef]
155. Guo H, Xiao T, Zhou H, Xie Y, Shen W. Hydrogen sulfide: a versatile regulator of environmental stress in plants. Acta Physiol Plant. 2016;38(1):16. doi:10.1007/s11738-015-2038-x. [Google Scholar] [CrossRef]
156. Riemenschneider A, Wegele R, Schmidt A, Papenbrock J. Isolation and characterization of a D-cysteine desulfhydrase protein from Arabidopsis thaliana. FEBS J. 2005;272(5):1291–304. doi:10.1111/j.1742-4658.2005.04567.x. [Google Scholar] [PubMed] [CrossRef]
157. Feng Y, Li CZ, Lin YJ, Yu XZ. Involvement of β-cyanoalanine synthase (β-CAS) and sulfurtransferase (ST) in cyanide (CN−) assimilation in rice seedlings. Chemosphere. 2022;294:133789. doi:10.1016/j.chemosphere.2022.133789. [Google Scholar] [PubMed] [CrossRef]
158. Gupta SK, Marwa N, Pandey AK, Zhang YB, Zhang JL. Hydrogen sulfide homeostasis in plants: an overview. In: Singh S, Singh VP, Tripathi DK, Prasad SM, Chauhan DK, Dubey NK, editors. Hydrogen sulfide in plant biology. New York: Academic Press; 2021. p. 341–63. doi:10.1016/B978-0-323-85862-5.00017-8. [Google Scholar] [CrossRef]
159. Zivanovic J, Kouroussis E, Kohl JB, Adhikari B, Bursac B, Schott-Roux S, et al. Selective persulfide detection reveals evolutionarily conserved antiaging effects of S-sulfhydration. Cell Metab. 2019;30(6):1152–70.e13. doi:10.1016/j.cmet.2019.10.007. [Google Scholar] [PubMed] [CrossRef]
160. Cuevasanta E, Lange M, Bonanata J, Coitiño EL, Ferrer-Sueta G, Filipovic MR, et al. Reaction of hydrogen sulfide with disulfide and sulfenic acid to form the strongly nucleophilic persulfide. J Biol Chem. 2015;290(45):26866–80. doi:10.1074/jbc.M115.672816. [Google Scholar] [PubMed] [CrossRef]
161. Khan NM, Siddiqui ZH, Naeem M, Abbas ZK, Ansari MW. Nitric oxide and hydrogen sulfide interactions in plants under adverse environmental conditions. In: Aftab T, Naeem M, editors. Emerging plant growth regulators in agriculture: roles in stress tolerance. New York: Academic Press; 2022. p. 215–44. doi:10.1016/B978-0-323-91005-7.00015-1. [Google Scholar] [CrossRef]
162. Guo Z, Liang Y, Yan J, Yang E, Li K, Xu H. Physiological response and transcription profiling analysis reveals the role of H2S in alleviating excess nitrate stress tolerance in tomato roots. Plant Physiol Biochem. 2018;124:59–69. doi:10.1016/j.plaphy.2018.01.006. [Google Scholar] [PubMed] [CrossRef]
163. Aroca A, Zhang J, Xie Y, Romero LC, Gotor C. Hydrogen sulfide signaling in plant adaptations to adverse conditions: molecular mechanisms. J Exp Bot. 2021;72(16):5893–904. doi:10.1093/jxb/erab239. [Google Scholar] [PubMed] [CrossRef]
164. Li ZG, Long WB, Yang SZ, Wang YC, Tang JH, Wen L, et al. Endogenous hydrogen sulfide regulated by calcium is involved in thermotolerance in tobacco Nicotiana tabacum L. suspension cell cultures. Acta Physiol Plant. 2015;37(10):219. doi:10.1007/s11738-015-1971-z. [Google Scholar] [CrossRef]
165. Li Z-G, Fang J-R, Bai S-J. Hydrogen sulfide signaling in plant response to temperature stress. Front Plant Sci. 2024;15:1337250. doi:10.3389/fpls.2024.1337250. [Google Scholar] [PubMed] [CrossRef]
166. Jin Z, Wang Z, Ma Q, Sun L, Zhang L, Liu Z, et al. Hydrogen sulfide mediates ion fluxes inducing stomatal closure in response to drought stress in Arabidopsis thaliana. Plant Soil. 2017;419(1–2):141–52. doi:10.1007/s11104-017-3335-5. [Google Scholar] [CrossRef]
167. Scuffi D, Nietzel T, Di Fino LM, Meyer AJ, Lamattina L, Schwarzländer M, et al. Hydrogen sulfide increases production of NADPH oxidase-dependent hydrogen peroxide and phospholipase D-derived phosphatidic acid in guard cell signaling. Plant Physiol. 2018;176(3):2532–42. doi:10.1104/pp.17.01636. [Google Scholar] [PubMed] [CrossRef]
168. Hu KD, Tang J, Zhao DL, Hu LY, Li YH, Liu YS, et al. Stomatal closure in sweet potato leaves induced by sulfur dioxide involves H2S and NO signaling pathways. Biol Plant. 2014;58(4):676–80. doi:10.1007/s10535-014-0440-7. [Google Scholar] [CrossRef]
169. Lisjak M, Teklić T, Wilson ID, Wood M, Whiteman M, Hancock JT. Hydrogen sulfide effects on stomatal apertures. Plant Signal Behav. 2011;6(10):1444–6. doi:10.4161/psb.6.10.17104. [Google Scholar] [PubMed] [CrossRef]
170. Duan B, Ma Y, Jiang M, Yang F, Ni L, Lu W. Improvement of photosynthesis in rice (Oryza sativa L.) as a result of an increase in stomatal aperture and density by exogenous hydrogen sulfide treatment. Plant Growth Regul. 2015;75(1):33–44. doi:10.1007/s10725-014-9929-5. [Google Scholar] [CrossRef]
171. Yastreb TO, Kolupaev YE, Havva EN, Shkliarevskyi MA, Dmitriev AP. Calcium and components of lipid signaling in implementation of hydrogen sulfide influence on the state of stomata in Arabidopsis thaliana. Cytol Genet. 2019;53(2):99–105. doi:10.3103/S0095452719020099. [Google Scholar] [CrossRef]
172. Liu Q, Liu R, Zhou Y, Wang W, Wu G, Yang N. Phospholipase Dδ and H2S increase the production of NADPH oxidase-dependent H2O2 to respond to osmotic stress-induced stomatal closure in Arabidopsis thaliana. J Plant Physiol. 2022;270(3):153617. doi:10.1016/j.jplph.2022.153617. [Google Scholar] [PubMed] [CrossRef]
173. Wang S, Zhang C, Chen R, Cheng K, Ma L, Wang W, et al. H2S is involved in drought-mediated stomatal closure through PLDα1 in Arabidopsis. Planta. 2024;259(6):142. doi:10.1007/s00425-024-04421-2. [Google Scholar] [PubMed] [CrossRef]
174. Siodmak A, Hirt H. Stomatal regulation: role of H2S-induced persulfidation in ABA signaling. Mol Plant. 2021;14(6):858–60. doi:10.1016/j.molp.2021.04.004. [Google Scholar] [PubMed] [CrossRef]
175. Huang D, Huo J, Liao W. Hydrogen sulfide: roles in plant abiotic stress response and crosstalk with other signals. Plant Sci. 2021;302:110733. doi:10.1016/j.plantsci.2020.110733. [Google Scholar] [PubMed] [CrossRef]
176. Chen S, Wang X, Jia H, Li F, Ma Y, Liesche J, et al. Persulfidation-induced structural change in SnRK2.6 establishes intramolecular interaction between phosphorylation and persulfidation. Mol Plant. 2021;14(11):1814–30. doi:10.1016/j.molp.2021.07.002. [Google Scholar] [PubMed] [CrossRef]
177. Chandrasekaran U, Luo X, Zhou W, Shu K. Multifaceted signaling networks mediated by Abscisic Acid Insensitive 4. Plant Commun. 2020;1(3):100040. doi:10.1016/j.xplc.2020.100040. [Google Scholar] [PubMed] [CrossRef]
178. Zhou M, Zhang J, Shen J, Zhou H, Zhao D, Gotor C, et al. Hydrogen sulfide-linked persulfidation of ABI4 controls ABA responses through the transactivation of MAPKKK18 in Arabidopsis. Mol Plant. 2021;14(6):921–36. doi:10.1016/j.molp.2021.03.007. [Google Scholar] [PubMed] [CrossRef]
179. Shen J, Zhang J, Zhou M, Zhou H, Cui B, Gotor C, et al. Persulfidationbased modification of cysteine desulfhydrase and the NADPH oxidase RBOHD controls guard cell abscisic acid signaling. Plant Cell. 2020;32(4):1000–17. doi:10.1105/tpc.19.00826. [Google Scholar] [PubMed] [CrossRef]
180. Pantaleno R, Scuffi D, Costa A, Welchen E, Torregrossa R, Whiteman M, et al. Mitochondrial H2S donor AP39 induces stomatal closure by modulating guard cell mitochondrial activity. Plant Physiol. 2023;191(3):2001–11. doi:10.1093/plphys/kiac591. [Google Scholar] [PubMed] [CrossRef]
181. Zhang J, Zhang L, Zhang W, Bo S, Pan J, Pei Y, et al. H2S activates succinate dehydrogenase through persulfidation to induce stomatal closure in Arabidopsis. Plant Soil. 2024;234(17):70. doi:10.1007/s11104-024-06837-x. [Google Scholar] [CrossRef]
182. Papanatsiou M, Scuffi D, Blatt MR, García-Mata C. Hydrogen sulfide regulates inward-rectifying K+ channels in conjunction with stomatal closure. Plant Physiol. 2015;168(1):29–35. doi:10.1104/pp.114.256057. [Google Scholar] [PubMed] [CrossRef]
183. Wang L, Wan R, Shi Y, Xue S. Hydrogen sulfide activates S-type anion channel via OST1 and Ca2+ modules. Mol Plant. 2016;9(3):489–91. doi:10.1016/j.molp.2015.11.010. [Google Scholar] [PubMed] [CrossRef]
184. Samantaray S, Kumari K. Hydrogen sulfide: an evolving gasotransmitter regulating salinity and drought stress response in plants. In: Fatma M, Sehar Z, Khan NA, editors. Gasotransmitters signaling in plant abiotic stress. Signaling and communication in plants. Cham: Springer; 2023. p. 71–91. doi:10.1007/978-3-031-30858-1_5. [Google Scholar] [CrossRef]
185. Zhang W, Wang L, Zhang L, Kong X, Zhang J, Wang X, et al. H2S-mediated balance regulation of stomatal and non-stomatal factors responding to drought stress in Chinese cabbage. Hortic Res. 2022;10(3):uhac284. doi:10.1093/hr/uhac284. [Google Scholar] [PubMed] [CrossRef]
186. Ma Y, Liang S, Huang L, Shao L. Hydrogen sulfide induces hydrogen peroxide and nitric oxide mediation of salt stress-caused stomatal closure in Arabidopsis thaliana (L.) Heynh. Ind J Exp Biol. 2024;62(3):157–68. doi:10.56042/ijeb.v62i03.4170. [Google Scholar] [CrossRef]
187. Hou L, Zhu D, Ma Q, Zhang D, Liu X. H2S synthetase AtD-CDes involves in ethylene and drought regulated stomatal movement. Sci Bull. 2016;61(15):1171–5. doi:10.1007/s11434-016-1128-5. [Google Scholar] [CrossRef]
188. Alvi AF, Khan S, Khan NA. Hydrogen sulfide and ethylene regulate sulfur-mediated stomatal and photosynthetic responses and heat stress acclimation in rice. Plant Physiol Biochem. 2024;207:108437. doi:10.1016/j.plaphy.2024.108437. [Google Scholar] [PubMed] [CrossRef]
189. Hou Z, Liu J, Hou L, Li X, Liu X. H2S may function downstream of H2O2 in jasmonic acid-induced stomatal closure in Vicia faba. Chin Bull Bot. 2011;46(4):396–406 (in Chinese). doi:10.3724/SP.J.1259.2011.00396. [Google Scholar] [CrossRef]
190. Deng G, Zhou L, Wang Y, Zhang G, Chen X. Hydrogen sulfide acts downstream of jasmonic acid to inhibit stomatal development in Arabidopsis. Planta. 2020;251(2):42. doi:10.1007/s00425-019-03334-9. [Google Scholar] [PubMed] [CrossRef]
191. Filipovic MR, Jovanovic VM. More than just an intermediate: hydrogen sulfide signalling in plants. J Exp Bot. 2017;68(17):4733–6. doi:10.1093/jxb/erx352. [Google Scholar] [PubMed] [CrossRef]
192. Tian B, Zhang Y, Jin Z, Liu Z, Pei Y. Role of hydrogen sulfide in the methyl jasmonate response to cadmium stress in foxtail millet. Front Biosci (Landmark Ed). 2017;22(3):530–9. doi:10.2741/4500. [Google Scholar] [PubMed] [CrossRef]
193. Yildirim E, Ekinci M, Turan M, Ors S, Dursun A. Physiological, morphological and biochemical responses of exogenous hydrogen sulfide in salt-stressed tomato seedlings. Sustainability. 2023;15(2):1098. doi:10.3390/su15021098. [Google Scholar] [CrossRef]
194. Yang T, Yuan G, Zhang Q, Xuan L, Li J, Zhou L, et al. Transcriptome and metabolome analyses reveal the pivotal role of hydrogen sulfide in promoting submergence tolerance in Arabidopsis. Environ Exp Bot. 2021;183:104365. doi:10.1016/j.envexpbot.2020.104365. [Google Scholar] [CrossRef]
195. Yastreb TO, Kolupaev YE, Havva EN, Horielova EI, Dmitriev AP. Involvement of the JIN1/MYC2 transcription factor in inducing salt resistance in Arabidopsis plants by exogenous hydrogen sulfide. Cytol Genet. 2020;54(2):96–102. doi:10.3103/S0095452720020127. [Google Scholar] [CrossRef]
196. Ma Y, Shao L, Zhang W, Zheng F. Hydrogen sulfide induced by hydrogen peroxide mediates brassinosteroid-induced stomatal closure of Arabidopsis thaliana. Funct Plant Biol. 2021;48(2):195–205. doi:10.1071/FP20205. [Google Scholar] [PubMed] [CrossRef]
197. Wang Z, Mu Y, Zhang L, Liu Z, Liu D, Jin Z, et al. Hydrogen sulfide mediated the melatonin induced stoma closure by regulating the K+ channel in Arabidopsis thaliana. Environ Exp Bot. 2023;205(2):105125. doi:10.1016/j.envexpbot.2022.105125. [Google Scholar] [CrossRef]
198. Chakraborty S, Roychoudhury A. Carbon compounds as gasotransmitters in plants under challenging environment. In: Aftab T, Corpas FJ, editors. Gasotransmitters signaling in plants under challenging environment. Cham: Springer; 2023. p. 299–314. doi:10.1007/978-3-031-43029-9_14. [Google Scholar] [CrossRef]
199. Hong K, Radani Y, Ahmad W, Li P, Luo Y. Carbon monoxide modulates auxin transport and nitric oxide signaling in plants under iron deficiency stress. Phyton-Int J Exp Bot. 2024;93(1):45–61. doi:10.32604/phyton.2023.046389. [Google Scholar] [CrossRef]
200. Feelisch M, Olson KR. Embracing sulfide and CO to understand nitric oxide biology. Nitric Oxide. 2013;35:2. doi:10.1016/j.niox.2013.06.004. [Google Scholar] [PubMed] [CrossRef]
201. Pal P, Sarangi PK, Singh AK, Husen A. Crosstalk of hydrogen sulfide and carbon monoxide with other plant growth regulators in plant defense, growth, and development. In: Husen A, Zhang W, editors. Hormonal cross-talk, plant defense and development. New York: Academic Press; 2023. p. 225–48. doi:10.1016/B978-0-323-95375-7.00008-2. [Google Scholar] [CrossRef]
202. Huang J, Han B, Xu S, Zhou M, Shen W. Heme oxygenase-1 is involved in the cytokinin-induced alleviation of senescence in detached wheat leaves during dark incubation. J Plant Physiol. 2011;168(8):768–75. doi:10.1016/j.jplph.2010.10.010. [Google Scholar] [PubMed] [CrossRef]
203. Shekhawat GS, Verma K. Haem oxygenase (HOan overlooked enzyme of plant metabolism and defence. J Exp Bot. 2010;61(9):2255–70. doi:10.1093/jxb/erq074. [Google Scholar] [PubMed] [CrossRef]
204. Bilban M, Haschemi A, Wegiel B, Chin BY, Wagner O, Otterbein LE. Heme oxygenase and carbon monoxide initiate homeostatic signaling. J Mol Med. 2008;86(3):267–79. doi:10.1007/s00109-007-0276-0. [Google Scholar] [PubMed] [CrossRef]
205. Liu Y, Xu S, Ling T, Xu L, Shen W. Heme oxygenase/carbon monoxide system participates in regulating wheat seed germination under osmotic stress involving the nitric oxide pathway. J Plant Physiol. 2010;167(16):1371–9. doi:10.1016/j.jplph.2010.05.021. [Google Scholar] [PubMed] [CrossRef]
206. Xie Y, Ling T, Han Y, Liu K, Zheng Q, Huang L, et al. Carbon monoxide enhances salt tolerance by nitric oxide-mediated maintenance of ion homeostasis and up-regulation of antioxidant defence in wheat seedling roots. Plant Cell Environ. 2008;31(12):1864–81. doi:10.1111/j.1365-3040.2008.01888.x. [Google Scholar] [PubMed] [CrossRef]
207. Han Y, Zhang J, Chen X, Gao Z, Xuan W, Xu S, et al. Carbon monoxide alleviates cadmium-induced oxidative damage by modulating glutathione metabolism in the roots of Medicago sativa. New Phytol. 2008;177(1):155–66. doi:10.1111/j.1469-8137.2007.02251.x. [Google Scholar] [PubMed] [CrossRef]
208. Yannarelli GG, Noriega GO, Batlle A, Tomaro ML. Heme oxygenase up-regulation in ultraviolet-B irradiated soybean plants involves reactive oxygen species. Planta. 2006;224(5):1154–62. doi:10.1007/s00425-006-0297-x. [Google Scholar] [PubMed] [CrossRef]
209. Jin Q, Cui W, Xie Y, Shen W. Carbon monoxide: A ubiquitous gaseous signaling molecule in plants. In: Lamattina L, Garcia-Mata C, editors. Gasotransmitters in plants signaling and communication in plants. Cham: Springer; 2016. p. 3–19. doi:10.1007/978-3-319-40713-5_1. [Google Scholar] [CrossRef]
210. She XP, Song XG. Carbon monoxide-induced stomatal closure involves generation of hydrogen peroxide in Vicia faba guard cells. J Integr Plant Biol. 2008;50(12):1539–48. doi:10.1111/j.1744-7909.2008.00716.x. [Google Scholar] [PubMed] [CrossRef]
211. Song XG, She XP, Zhang B. Carbon monoxide-induced stomatal closure in Vicia faba is dependent on nitric oxide synthesis. Physiol Plant. 2008;132(4):514–25. doi:10.1111/j.1399-3054.2007.01026.x. [Google Scholar] [PubMed] [CrossRef]
212. Cao Z, Huang B, Wang Q, Xuan W, Ling T, Zhang B, et al. Involvement of carbon monoxide produced by heme oxygenase in ABA-induced stomatal closure in Vicia faba and its proposed signal transduction pathway. Chin Sci Bull. 2007;52(17):2365–73. doi:10.1007/s11434-007-0358-y. [Google Scholar] [CrossRef]
213. Feng L, Wei L, Liu Y, Ren J, Liao W. Carbon monoxide/heme oxygenase system in plant: roles in abiotic stress response and crosstalk with other signals molecules. Nitric Oxide. 2023;138–139(2):51–63. doi:10.1016/j.niox.2023.06.005. [Google Scholar] [PubMed] [CrossRef]
214. Wang M, Liao W. Carbon monoxide as a signaling molecule in plants. Front Plant Sci. 2016;7(1665):572. doi:10.3389/fpls.2016.00572. [Google Scholar] [PubMed] [CrossRef]
215. Dubeaux G, Hsu PK, Ceciliato PHO, Swink KJ, Rappel WJ, Schroeder JI. Deep dive into CO2-dependent molecular mechanisms driving stomatal responses in plants. Plant Physiol. 2021;187(4):2032–42. doi:10.1093/plphys/kiab342. [Google Scholar] [PubMed] [CrossRef]
216. Shi K, Li X, Zhang H, Zhang G, Liu Y, Zhou Y, et al. Guard cell hydrogen peroxide and nitric oxide mediate elevated CO2-induced stomatal movement in tomato. New Phytol. 2015;208(2):342–53. doi:10.1111/nph.13621. [Google Scholar] [PubMed] [CrossRef]
217. Xu Z, Jiang Y, Zhou G. Response and adaptation of photosynthesis, respiration, and antioxidant systems to elevated CO2 with environmental stress in plants. Front Plant Sci. 2015;6:701. doi:10.3389/fpls.2015.00701. [Google Scholar] [PubMed] [CrossRef]
218. Woodward FI. Stomatal numbers are sensitive to increases in CO2 from pre-industrial levels. Nature. 1987;327(6123):617–8. doi:10.1038/327617a0. [Google Scholar] [CrossRef]
219. Wei Z, Abdelhakim LOA, Fang L, Peng X, Liu J, Liu F. Elevated CO2 effect on the response of stomatal control and water use efficiency in amaranth and maize plants to progressive drought stress. Agricult Water Manag. 2022;266(8):107609. doi:10.1016/j.agwat.2022.107609. [Google Scholar] [CrossRef]
220. Driesen E, Van den Ende W, De Proft M, Saeys W. Influence of environmental factors light, CO2, temperature, and relative humidity on stomatal opening and development: a review. Agronomy. 2020;10(12):1975. doi:10.3390/agronomy10121975. [Google Scholar] [CrossRef]
221. Zhang J, Wang N, Miao Y, Hauser F, McCammon JA, Rappel WJ, et al. Identification of SLAC1 anion channel residues required for CO2/bicarbonate sensing and regulation of stomatal movements. Proc Natl Acad Sci U S A. 2018;115(44):11129–37. doi:10.1073/pnas.1807624115. [Google Scholar] [PubMed] [CrossRef]
222. Sierla M, Hõrak H, Overmyer K, Waszczak C, Yarmolinsky D, Maierhofer T, et al. The receptor-like pseudokinase GHR1 is required for stomatal closure. Plant Cell. 2018;30(11):2813–37. doi:10.1105/tpc.18.00441. [Google Scholar] [PubMed] [CrossRef]
223. Hua D, Wang C, He J, Liao H, Duan Y, Zhu Z, et al. A plasma membrane receptor kinase, GHR1, mediates abscisic acid- and hydrogen peroxide-regulated stomatal movement in Arabidopsis. Plant Cell. 2012;24:2546–61. doi:10.1105/tpc.112.100107. [Google Scholar] [PubMed] [CrossRef]
224. Zhang H, Pan C, Gu S, Ma Q, Zhang Y, Li X, et al. Stomatal movements are involved in elevated CO2-mitigated high temperature stress in tomato. Physiol Plant. 2019;165(3):569–83. doi:10.1111/ppl.12752. [Google Scholar] [PubMed] [CrossRef]
225. Schulze S, Dubeaux G, Ceciliato PHO, Munemasa S, Nuhkat M, Yarmolinsky D, et al. A role for calcium-dependent protein kinases in differential CO2- and ABA-controlled stomatal closing and low CO2-induced stomatal opening in Arabidopsis. New Phytol. 2021;229(5):2765–79. doi:10.1111/nph.17079. [Google Scholar] [PubMed] [CrossRef]
226. Ying W, Liao L, Wei H, Gao Y, Liu X, Sun L. Structural basis for abscisic acid efflux mediated by ABCG25 in Arabidopsis thaliana. Nat Plants. 2023;9(10):1697–708. doi:10.1038/s41477-023-01510-0. [Google Scholar] [PubMed] [CrossRef]
227. Liang C, Li C, Wu J, Zhao M, Chen D, Liu C, et al. SORTING NEXIN2 proteins mediate stomatal movement and the response to drought stress by modulating trafficking and protein levels of the ABA exporter ABCG25. Plant J. 2022;110(6):1603–18. doi:10.1111/tpj.15758. [Google Scholar] [PubMed] [CrossRef]
228. Kuromori T, Miyaji T, Yabuuchi H, Shimizu H, Sugimoto E, Kamiya A, et al. ABC transporter AtABCG25 is involved in abscisic acid transport and responses. Proct Natl Acad Sci U S A. 2010;107(5):2361–6. doi:10.1073/pnas.0912516107. [Google Scholar] [PubMed] [CrossRef]
229. Zhang H, Hu S-L, Zhang Z-J, Hu L-Y, Jiang C-X, Wei Z-J, et al. Hydrogen sulfide acts as a regulator of flower senescence in plants. Postharvest Biol Technol. 2011;60(3):251–7. doi:10.1016/j.postharvbio.2011.01.006. [Google Scholar] [CrossRef]
230. Liu S, Wu Y, Li H, Cai D, Liang H, Ye C, et al. Physiological dynamics and transcriptomic analysis of cut roses ‘carola’ treated with KNO3. Phyton-Int J Exp Bot. 2023;92(12):3267–90. doi:10.32604/phyton.2023.045453. [Google Scholar] [CrossRef]
231. Cheng T, Hu L, Wang P, Yang X, Peng Y, Lu Y, et al. Carbon monoxide potentiates high temperature-induced nicotine biosynthesis in tobacco. Int J Mol Sci. 2018;19(1):188. doi:10.3390/ijms19010188. [Google Scholar] [PubMed] [CrossRef]
232. He M, He CQ, Ding NZ. Abiotic stresses: general defenses of land plants and chances for engineering multistress tolerance. Front Plant Sci. 2018;9:1771. doi:10.3389/fpls.2018.01771. [Google Scholar] [PubMed] [CrossRef]
Cite This Article
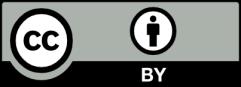
This work is licensed under a Creative Commons Attribution 4.0 International License , which permits unrestricted use, distribution, and reproduction in any medium, provided the original work is properly cited.