Open Access
ARTICLE
Comparative Effects of Compost and Arbuscular Mycorrhizal Fungi Versus NPK on Agro-Physiological, Biochemical and Tolerance Responses of Tomatoes to Drought
1 Multidisciplinary Faculty of Nador, Mohammed Premier University, Nador, 62700, Morocco
2 Centre d’Agrobiotechnologie et Bioingénierie, Unité de Recherche labellisée CNRST (Centre AgroBio-tech-URL-CNRST-05), Université Cadi Ayyad, Marrakech, 40000, Morocco
3 Laboratory of Agro-Food, Biotechnologies and Valorization of Plant Bioresources (AGROBIOVAL), Department of Biology, Faculty of Science Semlalia, Cadi Ayyad University (UCA), Marrakesh, 40000, Morocco
4 Phytopathology Unit, Department of Plant Protection, École Nationale d’Agriculture de Meknès, Km10, Rte Haj Kaddour, Meknès, 50001, Morocco
5 African Sustainable Agriculture Research Institute (ASARI), Mohammed VI Polytechnic University (UM6P), Laayoune, 70000, Morocco
* Corresponding Authors: Abderrahim Boutasknit. Email: ; Rachid Lahlali. Email:
Phyton-International Journal of Experimental Botany 2024, 93(12), 3589-3616. https://doi.org/10.32604/phyton.2024.057881
Received 29 August 2024; Accepted 07 November 2024; Issue published 31 December 2024
Abstract
Drought stress (DS) and overuse of chemical fertilizers cause considerable losses in the agro-physiological as well as biochemical performance of plants. In this context, considerable effort will be required to replace chemical fertilizers (NPK) with biostimulants as an important approach to enhance the productivity and sustainability of agriculture. Here, we evaluated the effect of separating and/or combining arbuscular mycorrhizal fungi (AMF) with compost (C) in comparison to the use of NPK on the growth, physiological and biochemical of tomatoes under DS. The findings showed that DS significantly reduced the growth and physiological attributes of tomatoes. Furthermore, the treatment of AMF and C showed better results in agro-physiological and fruit quality compared to the NPK and control under DS. The combination of AMF and C (C+AMF) increased leaf water potential by 18.8%, stomatal conductance by 14.1%, fresh fruit weight by 25.0%, shoot dry matter by 104% and root dry matter by 56.1% compared to the control under DS. The study revealed that C+AMF caused a significant increase in sugar, protein and activity of polyphenoloxidase and peroxidase in leaves and fruits, and an opposite trend was observed in the case of malonaldehyde and hydrogen peroxide compared to NPK and control under DS. In conclusion, it is recommended to utilize the combination of AMF with compost to enhance the growth, yield, osmotic adjustment, and antioxidant capacity of tomato plants. This approach can boost their resilience to water stress and improve overall fruit quality.Keywords
Tomatoes are the most widely cultivated and consumed vegetables worldwide, second only to potatoes [1]. Tomatoes are an essential food crop for all sectors of the economy, regardless of whether they are in developed or underdeveloped countries, and they are consumed by a large number of people [2]. In Morocco, the importance of the vegetable crop is demonstrated by the large area under cultivation, which is about 14 781 ha and yields 1.4 million tons in 2020 (FOASTAT, 2022). The horticultural applicability of tomato’s fleshy vegetable nature has reawakened interest in its wide range of uses in food and feed ingredients [2]. They serve as a valuable nutritional supplement, widely incorporated into salads and a variety of dishes, including pasta, pizza, and other baked goods. Their versatility enhances both the flavor and nutritional profile of numerous culinary creations. Lycopene, flavonoids, beta-carotene, and lutein, as well as vitamins C and E are all health-promoting components in tomatoes [3].
In addition, tomato production and yield are negatively impacted by poor soil, drought, salinity, temperature, and environmental changes. However, the impact of drought continues to be important in the arid as well as semi-arid regions and is considered the main factor in crop loss. This stress negatively affects soil nutrient availability and transport to plant and crop productivity worldwide [4], which has led to soil degradation and reduced agricultural productivity [5]. For this reason, farmers are forced to look for solutions such as the use of conventional chemical fertilizers to ensure a large agricultural production and cover the needs of a growing population. Indeed, the excessive use of these chemical fertilizers results in the alteration of soil structure and fertility and the reduction of soil microbial activity [6]. In addition, drought and chemical fertilizer application are important factors increasing soil degradation and limiting plant growth and productivity [7].
In this context, it is crucial to develop and implement management strategies and practices that enhance plant resilience to abiotic stresses, with the aim of boosting crop yields and improving soil fertility. In light of this critical situation, it is imperative to promote continuity in agricultural development and protect natural resources by striking a balance between environmental quality and economic viability. This can be accomplished through the development of organic farming systems that emphasize nutrient recycling and optimize soil water availability. Given this pressing challenge, advancing agricultural sustainability and natural resource management is essential. This involves fostering support for practices that harmonize environmental health with economic viability, through the development of biologically integrated agro-ecosystems that emphasize nutrient cycling and efficient soil water management.
Biological agriculture and sustainable agroecology are crucial in ensuring the resilience and sustainability of agricultural production amid the challenges posed by climate change. These approaches foster environmentally responsible practices and enhance the adaptability of farming systems to evolving climatic conditions. These approaches involve using biostimulants as an eco-friendly alternative to chemical fertilizers, combined with the adoption of cost-effective and reproducible biological and bio-organic practices. These practices include the application of compost and AMF, which enhance soil health and promote sustainable agricultural methods. These biostimulants can enhance soil structure and aeration, improve plant growth, regulate water balance, boost microbiological activity, and provide protection against abiotic stresses [8,9].
Currently, the biostimulants such as AMF and composts represent a promising technological advancement for soil restoration. These methods enhance soil health, improve carbon sequestration, increase energy efficiency, and elevate agricultural productivity [10]. Moreover, compost application enhances the retention of available nutrients for plant growth by improving water retention, porosity, surface area, cation exchange capacity, and beneficial microorganisms in the soil [11]. Additionally, compost application boosts soil nitrogen and organic carbon levels, which support microbial communities, thereby enhancing soil health and increasing crop yields [12]. The application of compost markedly improves plant resilience to drought stress by boosting photosynthetic activity and optimizing mineral nutrition [13–16].
The inoculation of plants with native beneficial microorganisms, such as AMF, further enhances drought tolerance by facilitating more efficient water uptake and nutrient assimilation [17]. AMF have been proven to markedly boost plant growth and nutrient uptake [18]. They enhance water use efficiency by finely tuning stomatal regulation and modulating aquaporin activity in plants [19]. Furthermore, AMF play a crucial role in alleviating oxidative stress and minimizing yield loss under adverse conditions, thereby fortifying overall plant resilience and productivity [20]. They foster the formation of resilient and stable soil aggregates, significantly improving soil structure and water-holding capacity. Additionally, they contribute to the efficient transport of water—accounting for up to 20% of the total water absorbed by roots—and enhance root hydraulic conductivity, particularly under stress conditions, thereby supporting overall soil health and plant resilience [20,21]. In addition, AMF can improve plant stress tolerance by triggering rapid defense mechanisms and offering protection against oxidative damage induced by abiotic stress [22,23]. AMF also influence root morphology, primarily by stimulating lateral root growth [24]. Their effects on physiology can include alterations in leaf water relations [12,25,26], signaling between roots and shoots and hormone production [27], enhanced secondary metabolites biosynthesis such as strigolactones—which facilitate the establishment and maintenance of AMF symbiosis with the host plant—and the regulation of antioxidative enzymes [28–31].
The impacts of AMF colonization of tomato plants alone or in combination with compost under varying water availability are investigated in this study, with an emphasis on plant growth, physiological features, and plant water status. For this purpose, we tested the effects of biostimulants (AMF (Rhizophagus irregularis strain) alone and or in combination with compost) and conventional chemical fertilizers on tomato plants under drought stress. Given the beneficial effects of combining natural biostimulants on tomato plants under drought stress, we conducted a study with the following objectives: (i) to assess the growth, physiological and biochemical responses of tomatoes treated with natural biostimulants compared to conventional fertilizers, (ii) to evaluate the effectiveness of natural biostimulants vs. conventional fertilizers in enhancing tomato tolerance to drought stress, and (iii) to identify sustainable alternatives for increasing tomato productivity while reducing reliance on conventional fertilizers under drought conditions.
We hypothesize that utilizing natural biostimulants, either individually or in combination, as a sustainable agricultural practice will enhance physiological and biochemical indicators and significantly improve the productivity of tomato plants under water stress, surpassing the effects of conventional fertilizers.
2.1 Experimental Design and Biofertilizer Materials
Cherry tomato seeds (Lycopersicon esculantum Mill.) were sourced from the National Institute for Agricultural Research in Marrakesh, Morocco. Lycopersicon esculentum, commonly known as the tomato, is classified within the domain Eukaryota, the kingdom Plantae, the phylum Angiosperms, the class Eudicots, the order Solanales, and the family Solanaceae. Its genus is Lycopersicon and its species is Lycopersicon esculentum. The tomato seeds were first disinfected by dipping in a 10% solution of sodium hypochlorite for 10 min, then rinsed five times with distilled water. Germination was carried out in plastic Petri dishes lined with sterile filter paper discs and incubated in the dark at 26°C for 5 days, after which the germinated seeds were transferred into plastic trays filled with autoclaved peat. When the seedlings reached the two-leaf stage, they were transferred directly to plastic pots with 5 kg of soil. For a period of two months, the tomato plants were irrigated to maintain soil moisture at 75% of field capacity (FC). Soil moisture levels in all pots were carefully monitored twice daily, in the morning and evening, using a Time-Domain Reflectometry meter to ensure consistent conditions for optimal plant growth.
The experiment comprised four treatments: (i) Control: tomatoes receiving no biofertilizer, (ii) Compost (C): tomatoes receiving 5% of compost (w/w), (iii) Chemical fertilizer (NPK): tomatoes receiving the optimal dose of ammonium nitrate (134 kg N/ha), superphosphate (127 kg P2O5/ha) and potassium sulfate (332 kg K2O/ha) [32]), (iv) AMF: tomatoes receiving a pure arbuscular mycorrhizal fungus strain (Rhizoglomus irregulare DAOM 197198), and (v) C+AMF: tomatoes receiving a combination of compost and AMF. The tomatoes were organized according to a completely randomized block design, with each treatment replicated five times biologically to ensure statistical validity.
The used soil in the experiment was categorized by a composition of 51% sand, 19% clay, and 30% loam. It had an organic matter content of 1% and a total organic carbon percentage of 0.58%. The soil contained 11 ppm of available phosphorus and had a nitrogen concentration of 0.84 mg/g. Its pH level was 8.6, and the electrical conductivity (EC) was 0.19 mS/cm. These soil properties were carefully measured to ensure they were suitable for evaluating plant growth and nutrient absorption under the experimental conditions.
The fungal strain R. irregulare used in the experiment was DAOM 197198, a pure arbuscular mycorrhizal fungus (AMF) strain supplied by the Plant Biotechnology Institute in Montreal, Canada. R. irregularis is a fungus belonging to the domain Eukaryota, the kingdom Fungi, the phylum Glomeromycota, the class Glomeromycetes, the order Glomerales, the family Glomeraceae, and the genus Rhizophagus. Cocultivation with maize significantly increased the number of propagules of R. irregulare. Corn roots, which contained hyphae, vesicles, and spores, were collected, chopped into small fragments, and used as inoculum. For inoculating the tomato plants, 30 g of mycorrhizal spores were mixed with 2 g of mycorrhizal root fragments, which exhibited a high AMF infection frequency of 96% and an intensity of 60%. This inoculum was characterized by having 748 AMF spores per 100 g of soil. In the control treatments, non-mycorrhizal corn roots were added in equivalent amounts to ensure consistent levels of organic matter in the pots. Additionally, filtered inoculum was utilized to reintroduce other beneficial soil microorganisms not associated with AMF, enhancing overall soil health.
The compost employed in this study was made from grass waste, following the method outlined by Meddich et al. [33]. The compost physicochemical properties were as follows: pH of 6.9, Olsen available phosphorus (P) of 2.5 mg/g, potassium (K) at 800 mg/kg, calcium (Ca) at 1900 mg/kg, organic carbon (C) at 306.5 g/kg, organic matter at 551.7 g/kg, nitrogen (N) at 21.9 g/kg, a C/N ratio of 14, and ash content of 490 g/kg. During the transplantation process, the compost was incorporated into the soil at a rate of 5% (w/w). This compost provided essential nutrients and organic matter to support plant growth and enhance soil fertility.
2.2 Fungal Colonization and Growth
Root samples were meticulously cleaned and then subjected to a 10% potassium hydroxide (KOH) solution at 90°C for 30 min to clear the tissue. Following this, the samples were acidified in 5% lactic acid for 15 min to neutralize the KOH. The roots were then stained with a 0.05% (w/v) solution of Trypan blue at 90°C for 20 min to visualize mycorrhizal colonization [34]. The extent of mycorrhizal colonization was quantified using the gridline-intersect method, assessing both colonization frequency (F) and intensity (I) with a minimum of 100 intersects per sample to ensure accuracy and reliability in the measurements [35]. The frequency (F) of fungal structures within the root system and the intensity (I) of mycorrhizal colonization were determined by analyzing 20 randomly selected root fragments, each 1 cm in length, per microscope slide. This procedure was repeated five times for each sample, resulting in a total of 100 root fragments. F and I were then calculated based on these 100 fragments as follows:
F (%)= (Infected root segmentstotal root segments)× 100 (1)
I (%)=(95n5+70n4+30n3+5n2+n1)Total root segments (2)
where n denotes fragments classified by infection rates as follows: n5 represents fragments with infection rates above 90% but less than 100%; n4 corresponds to infection rates between 50% and 90%; n3 indicates infection rates between 10% and 50%; n2 refers to infection rates between 1% and 10%; and n1 encompasses infection rates between 0% and 1%.
At the end of the light period, fully mature leaves from tomato plants subjected to biofertilizers and/or water stress were collected, rapidly snap-frozen in liquid nitrogen, and then ground into a fine powder using a pestle as well as mortar. This preparation was done for analyses conducted after 4 months. To assess growth performance, we measured various parameters including root length (RL), shoot height (SH), and both root (RDW) and shoot (SDW) dry weights. The samples were dried at 80°C until they reached a stable weight, at which point their dry weights were recorded, ensuring precise measurements of biomass accumulation and plant development under the experimental conditions.
2.3 Leaf Water Potential (ΨLeaf) and Relative Water (RWC) Content
Leaf water potential (ΨLeaf) was measured before sunrise, between 06:00 and 08:00 h, using a 600-EXP Super Pressure Chamber (PMS Instrument, Albany, OR, USA). Measurements were taken on fully mature leaves at the top of the stems from five different plants to ensure consistency. To determine the RWC, we followed the procedure described by Barrs et al. [35]. Circular discs with a 1 cm2 area, taken from the apex of the third fully developed leaf, were first weighed to obtain their fresh weight (FW). These discs were then placed in demineralized water for 24 h at 4°C in the dark to achieve full turgor, after which their turgor weight (TW) was measured. The discs were then dried at 70°C until a constant weight was achieved to determine the dry matter (DM). The relative water content (RWC) was calculated using the standard formula to evaluate the leaf water status.
RWC(%)=(FW−DM)(TW−DM)×100 (3)
2.4 Photosynthetic Pigments, Stomatal Conductance, and Chlorophyll Fluorescence
The carotenoids, chlorophyll a, and chlorophyll b concentrations were assessed using Arnon’s method [36]. Absorbance readings of the supernatant were recorded using a UV–visible spectrophotometer (UV-3100PC, VWR) at wavelengths of 480, 645, and 663 nm to quantify pigment concentrations.
Stomatal conductance (gs) was taken on mature leaves with a portable steady-state diffusion porometer (Leaf Porometer LP1989, Decagon Devices, Inc., Washington, DC, USA). Five measurements were taken on the abaxial side of each plant between 09:30 and 11:00 a.m. for each treatment to ensure accurate readings.
Chlorophyll fluorescence was analyzed using a portable fluorometer (Hudson, NY, USA). Fully developed leaves were dark-adapted for 30 min using clips before measurements. The quantum yield of photosystem II (Fv/Fm) was evaluated using the formula Fv/Fm = (Fm − F0)/Fm, where Fm and F0 represent the maximum and initial fluorescence values, respectively, to evaluate the photosynthetic efficiency of the dark-adapted leaves.
2.5 Quantification of Total Soluble Sugars and Proteins
To measure total soluble sugars (TSS), 0.1 g of frozen leaf samples were homogenized in 4 mL of 80% ethanol. After centrifugation, 0.25 mL of a 5% phenol solution and 1.25 mL of concentrated sulfuric acid were added to the supernatant. Following the procedure outlined by Dubois et al. [37], the absorbance was measured at 485 nm using a UV-3100PC spectrophotometer, and TSS content was quantified based on a standard glucose curve.
For protein content and enzyme activity analysis, 0.25 g of frozen leaf tissue was homogenized in 1 M phosphate buffer (pH 7) containing 5% polyvinylpolypyrrolidone (PVPP) to prevent phenolic interference. The homogenate was centrifuged at 18,000× g for 15 min at 4°C, and the supernatant was used to determine protein levels and antioxidant enzyme activities [38]. Enzyme activities were assessed using specific assays to gauge the functionality of key antioxidant enzymes.
2.6 Quantification of Malondialdehyde and Hydrogen Peroxide Content
Malondialdehyde (MDA) levels were measured using the procedure described by Savicka et al. [39]. This method provides insight into the levels of lipid peroxidation and oxidative stress within the tissues. MDA content was calculated as follows:
[MDA] = 6.45 × (A5324A600) - 0.565A450
Hydrogen peroxide (H2O2) levels in leaf and fruit tissues were assessed using the method described by Velikova et al. [40]. Frozen tissue samples were homogenized in 5 mL of 10% (w/v) trichloroacetic acid (TCA), then centrifuged at 15,000× g for 15 min at 4°C. From the resulting supernatant, 0.5 mL was homogenized with 0.5 mL of 10 mM potassium phosphate buffer (pH 7.0) and 1 mL of 1 M potassium iodide solution. The mixture was incubated in the dark at room temperature for 1 h. The H2O2 was assessed by determining the absorbance at 390 nm using a UV-Vis spectrophotometer, with readings compared against a standard H2O2 calibration curve. This procedure helps evaluate oxidative stress by quantifying hydrogen peroxide levels in the tissues.
2.7 Antioxidant Enzymes Contents
Fresh leaf tissue (0.1 g) was pulverized in liquid nitrogen, then homogenized with 4 mL of 1 M phosphate buffer (pH 7.0) and 5% polyvinylpolypyrrolidone (PVPP) using a cold mortar. The homogenate was centrifuged at 18,000× g for 15 min at 4°C, and the supernatant was used for measuring antioxidant enzyme activities. Peroxidase (POX) activity was assessed over a three-minute period following the method of Tejera García et al. [38]. The reaction mixture included 2 mL of K2HPO4/KH2PO4 buffer (100 mM; pH 6.5), 1 mL of guaiacol (40 mM), 0.5 mL of H2O2 (10 mM), and 0.1 mL of enzyme extract. Results were reported in units per mg of protein.
Polyphenoloxidase (PPO) activity was measured by evaluating the catechol oxidation at 410 nm, as described by [41]. The assay used 2 mL of K2HPO4/KH2PO4 buffer (pH 6; 100 mM) along with 2 mL catechol (50 mM) and 0.1 mL of enzyme extract. PPO activity was quantified and reported in units per mg of protein. This comprehensive approach allowed for the detailed assessment of antioxidant enzyme activities in the leaf tissues.
All data were analyzed using a multifactorial analysis of variance (MANOVA) by SPSS version 23.0 software (IBM, Armonk, NY, USA) for the experienced factors (AMF; A, NPK; B, Compost; C and drought; D) and their interactions. For mean comparison and to determine significant differences between treatment groups, Tukey’s honest significant difference (HSD) test was applied at a significance level of 5% (p ≤ 0.05). This approach allowed for a comprehensive evaluation of treatment effects and ensured robust statistical validation of the results.
Principal component analysis (PCA) was made using XLSTAT-software v. 2016, and Heatmap was achieved by the software GraphPad® Prism v9.0 to classify growth, physiological, and biochemical traits of tomatoes under WW, and DS that elucidate the distinction between the different treatments applied as well as the studied variables.
3.1 Mycorrhizal Status and Tomato Growth
Drought stress (DS) significantly reduced both the frequency (F) (p < 0.001) and intensity (I) (p < 0.01) of mycorrhization, as detailed in Tables 1 and 2. Non-inoculated plants exhibited no signs of root colonization by AMF. The presence of AMF notably enhanced significantly (p < 0.001) both the F and I of mycorrhization in tomato roots. Under WW conditions, plants treated with AMF alone or with a combination of AMF and compost (C+AMF) displayed significantly higher mycorrhizal F (F > 72%) and I (I > 51%) compared to those grown under drought stress conditions. Specifically, under WW conditions, the intensity of mycorrhizal infection was approximately doubled in plants treated with both compost and AMF (C+AMF) compared to those under DS and those inoculated with AMF alone. Moreover, when compared to AMF-only treatments, the mycorrhizal F and I were increased by 35% and 17%, respectively, in plants treated with C+AMF under drought stress conditions. The effect of interactions among all factors (AMF x compost x drought) was significant (p < 0.001) for intensity of mycorrhization (Table 1).
The addition of conventional chemical fertilizers (NPK) and AMF alone and/or combined with compost enhanced growth parameters in tomato plants under favorable irrigation conditions (WW). The DS application significantly affected tomato plant growth by reducing shoot height (SH) (p < 0.001), root length (RL) (p < 0.01), leaves number (LN) (p < 0.01), fruits number (FN) (p < 0.01), fresh fruit weight (FFW) (p < 0.01), and shoot dry weight (SDW) (p < 0.001) and root dry weight (RDW) (p < 0.001) accumulation (Tables 1 and 3). These parameters recorded a significant decrease especially in tomato plants not inoculated and not amended (NPK and control) under unfavorable irrigation conditions. DS showed a greater reduction in SH by 24.2% and 17.7%, in RL by 13.3% and 12.6%, in LN by 25.9% and 22.1%, in FN by 34.5% and 43.6%, in FFW by 15.8% and 18.8%, in SDW by 13.1% and 17.5%, and in RDW by 46.6% and 41% respectively in NPK and control compared to the control plants under WW conditions. Furthermore, the addition of compost alone and/or in combination with AMF (C+AMF) increased respectively, SH by 12.1% and 17.9%, RL by 5.5% and 7.6 %, LN by 21.% and 22.6%, FN by 29% and 35.5%, FFW by 21.3% and 25%, SDW by 10.7% and 10.4%, and RDW by 24.1% and 56.1% compared to the control plants under DS conditions (Table 3). The combined application of AMF and compost yielded superior results and more effectively alleviated the effects of water stress on growth parameters compared to the use of AMF or compost alone. Conversely, under well-watered (WW) conditions, conventional chemical fertilizers significantly enhanced biomass and yield relative to plants treated with AMF, compost, or left untreated. Specifically, the application of NPK fertilizers resulted in a 27% increase in SH, a 35% increase in NL, a 25% increase in SDW, a 33% increase in FN, and a 38% increase in FFW in tomato plants compared to the control. The interactions between AMF, compost, and drought had a significant effect on SDW, with p < 0.001 (Table 1).
3.2 Leaf Water Potential and Relative Water Content
Leaf water potential (ΨLeaf) (Fig. 1a) and relative water content (RWC) (Fig. 1b) were significantly (p < 0.001) reduced under water stress conditions (Table 1). Neither compost alone, AMF, nor NPK treatments had a notable impact on ΨLeaf compared to the control plants under WW conditions. However, under DS, the application of compost, either alone or in combination with AMF (C+AMF), had a significant effect on ΨLeaf compared to NPK-treated and untreated plants. Specifically, compost alone increased ΨLeaf by 19.8% compared to the control plants under DS conditions. In contrast, NPK application resulted in an 8.8% reduction in ΨLeaf relative to the control plants under DS conditions.
Figure 1: Effects of compost (C), chemical fertilizer (NPK), and arbuscular mycorrhizal fungi (AMF) on leaf water potential (a) and relative water content (b) in tomatoes under well-watered (WW) and drought stress (DS) conditions. Values marked with distinct letters indicate significant differences between treatments, with a significance level set at p ≤ 0.05, as determined by the Tukey test for each harvest period
Under DS conditions, the RWC decreased by 20.1% in plants treated with NPK and by 19.4% in control plants compared to plants under WW conditions. Conversely, plants treated with compost alone or with the combined treatment of compost and AMF (C+AMF) exhibited the greatest increases in RWC, with increases of 9.7% and 8.2%, respectively, compared to the untreated plants under DS conditions. This indicates that compost and AMF treatments were more effective in enhancing water retention compared to other treatments during drought stress. The interactions among NPK and drought, on the leaf water potential were significant at p < 0.05 (Table 1).
3.3 Stomatal Conductance, Chlorophyll Fluorescence and Photosynthetic Pigments
DS reduced stomatal conductance (gs) (Fig. 2a) and photosystem II quantum efficiency (Fv/Fm) (Fig. 2b) significantly (p < 0.001), with the decreases being especially pronounced in the absence of biostimulants (compost and AMF) (Table 1). When compared to control and NPK treatments, C+AMF enhanced the gs by 14.5% and 15.7%, respectively, under DS conditions. Furthermore, the combined application of compost with AMF (C+AMF) increased Fv/Fm by 3.6% and 3.4% respectively compared to NPK-treated and control plants under water stress. In contrast, the addition of NPK to tomato plants recorded the highest value under favorable irrigation conditions compared to the plants treated with biostimulants. The interactions among NPK and drought, on the stomatal conductance were significant at p < 0.001 (Table 1).
Figure 2: Impactof arbuscular mycorrhizal fungi (AMF), compost (C), and chemical fertilizer (NPK) on stomatal conductance (a) and chlorophyll fluorescence (Fv/Fm) (b) in tomato plants grown under well-watered (WW) and drought stress (DS) conditions
Following the application of DS, photosynthetic pigment concentrations significantly decreased (p < 0.001) across all treatments (Table 1), including those with biostimulants and NPK (Fig. 3). DS conditions caused considerable reductions in chlorophyll a (Chl a) (Fig. 3a), chlorophyll b (Chl b) (Fig. 3b), and carotenoids (Fig. 3c). Specifically, reductions in Chl a, Chl b, and carotenoids were more pronounced in tomato plants treated with NPK, showing decreases of 7.8%, 11.7%, and 20.1%, respectively, and in control plants with decreases of 8.9%, 18.3%, and 34.5%, compared to the control plants under WW conditions. In contrast, the application of compost combined with AMF (C+AMF) significantly mitigated these reductions, leading to increases in Chl a, Chl b, and carotenoids by 7.7%, 21.9%, and 29.9%, respectively, in comparison with control plants under DS conditions. Under WW conditions, the use of NPK was associated with higher concentrations of chlorophyll a and b, as well as carotenoids, compared to both biostimulant treatments (either alone or in combination) and untreated control plants. This indicates that while DS negatively impacts photosynthetic pigments, the combined use of compost and AMF can partially counteract these effects, and NPK treatment enhances pigment concentrations under optimal water conditions. The interactions among NPK and drought, on the chlorophyll a, chlorophyll b, and carotenoids were significant at p < 0.001 (Table 1).
Figure 3: Effects of compost (C) and a chemical fertilizer (NPK), and arbuscular mycorrhizal fungi (AMF) on chlorophyll a (a), chlorophyll b (b) and carotenoid (c) in tomatoes under well-watered (WW) and drought stress (DS) conditions
3.4 Quantification of Total Soluble Sugars and Proteins
Under drought stress (DS) conditions, the levels of total soluble sugars (TSS) and protein in tomato leaves and fruits decreased significantly (p < 0.001) (Fig. 4 and Table 1). Specifically, TSS levels dropped by approximately 13.6% and 10.9% in leaves (Fig. 4a) and 12.9% and 10.9% in fruits (Fig. 4b) for control and NPK-treated plants, respectively, compared to the WW controls. Similarly, protein content decreased by about 17.6% and 17.3% in leaves (Fig. 4c) and 17.7% and 16.9% in fruits (Fig. 4d) for control and NPK-treated plants, respectively, under DS conditions relative to WW conditions. Conversely, the application of compost alone (C) and in combination with AMF (C+AMF) significantly improved TSS content, with increases of 20.1% and 18.7% in leaves and 9.8% and 11.4% in fruits, respectively, compared to the control plants under DS conditions. Similarly, protein content was enhanced by 15.8% and 17.6% in leaves and 23.2% and 5.1% in fruits for compost and C+AMF treatments, respectively, under DS conditions. Under WW conditions, NPK treatment also led to increased TSS and protein content, with rises of 20.5% and 18.9% in leaves and 9.0% and 7.3% in fruits, respectively, compared to the control. The interactions among NPK and drought, on the total soluble sugars and protein content in tomato leaves and fruits were significant at p < 0.001 (Table 1).
Figure 4: Effects of arbuscular mycorrhizal fungi (AMF), compost (C) and a chemical fertilizer (NPK) on leaf sugar (a), fruit sugar (b), leaf protein (c) and fruit protein content (d) in tomatoes under well-watered (WW) and drought stress (DS) conditions
3.5 Quantification of Hydrogen Peroxide (H2O2) and Malondialdehyde (MDA) Content
H2O2 and MDA levels serve as key indicators of oxidative stress in plant tissues, as depicted in Fig. 5. Under DS conditions, both H2O2 and MDA concentrations increased significantly (p < 0.001) in leaves and fruits compared to WW conditions (Table 1). Specifically, H2O2 levels rose by 93.4% and 70.3% in leaves (Fig. 5a) and by 15.6% and 8.9% in fruits (Fig. 5b) for control and NPK-treated plants under DS circumstances, respectively. Similarly, MDA content surged by 133.4% and 124.7% in leaves (Fig. 5c) and by 7.0% and 9.7% in fruits (Fig. 5d) for control and NPK-plants, respectively, under DS. In contrast, the application of AMF and/or compost led to reductions in H2O2 and MDA levels in leaves under DS conditions. Notably, the combination of compost and AMF (C+AMF) was particularly effective, reducing H2O2 content by 47.6% and 39.8% and MDA content by 9.8% and 22.0% in leaves and fruits, respectively, compared to the control under DS. The lowest oxidative stress values were consistently observed in plants treated with compost, AMF, or both, compared to the control, regardless of the water regime applied. The interactions between AMF, compost and drought, on the hydrogen peroxide and malondialdehyde content were significant at p < 0.001 and p < 0.01 in leaves and fruits, respectively (Table 1).
Figure 5: Effects of arbuscular mycorrhizal fungi (AMF), compost (C) and a chemical fertilizer (NPK) on hydrogen peroxide (H2O2) content in leaf (a) and in fruit (b) and malondialdehyde (MDA) content in leaf (c) and in fruit (d) in tomatoes under well-watered (WW) and under drought stress (DS) conditions
3.6 Antioxidant Enzymes Contents
Tomato plants exposed to drought stress (DS) showed a significant increase (p < 0.01) in the activity of antioxidant enzymes, including polyphenol oxidase (PPO) and peroxidase (POX), in both leaves and fruits compared to those under well-watered (WW) conditions (Tables 1 and 4). Specifically, PPO activity saw the highest increase of 35.5% in leaves and 91.3% in fruits in AMF-inoculated plants under DS conditions compared to the control plants. Similarly, POX activity was significantly elevated by 43.5% in leaves and 52.3% in fruits for plants treated with compost alone under DS environments relative to the control plants. Under WW conditions, the most notable improvements in PPO and POX activities in both leaves and fruits were observed with the supplementation of compost and AMF, either separately or in combination. These improvements were generally greater than those achieved with NPK treatment. This suggests that compost and AMF treatments are particularly effective at enhancing antioxidant enzyme activity, thereby providing better protection against oxidative stress under both water stress and optimal conditions. The interactions between AMF, compost, and drought significantly affected peroxidase content in both leaves and fruits (p < 0.05) (Table 1).
3.7 Correlation between Agro-Physiological and Biochemical Traits
Fig. 6 illustrates the Pearson correlations among various physiological, biochemical, and growth traits. A significant positive correlation was detected between growth parameters—including root dry weight (RDW), shoot dry weight (SDW), number of leaves (LN), shoot height (SH), root length (RL), and fruit fresh weight (FFW) and physiological parameters such as stomatal conductance (gs) and the chlorophyll fluorescence (Fv/Fm). In contrast, stress markers, namely hydrogen peroxide (H2O2) and malondialdehyde (MDA), along with antioxidant enzyme activities (peroxidase (POX), and polyphenol oxidase (PPO)) in both leaves and fruits, displayed a significant negative correlation with growth parameters. Additionally, stomatal conductance (gs) was strongly positively correlated with leaf water potential (ψLeaf). Conversely, the sugar (TSS) content in leaves and fruits has shown a stronger negative correlation with stress markers and growth parameters. These relationships highlight how physiological and biochemical responses to stress influence plant growth and overall health.
Figure 6: Pearson correlation analysis of the evaluated parameters in tomato plants grown in WW (well-watered) and DS (drought stress) and treated or not (control) with arbuscular mycorrhizal fungi (AMF), compost (C), combination of compost with AMF (C+AMF) and chemical fertilizer (NPK). RL: root length; SH: shoot height; SDW: shoot dry weight; RDW: root dry weight; Gs: stomatal conductance; Fv/Fm: chlorophyll fluorescence; RWC: relative water content; LWP: leaf water potential; I: mycorrhizal intensity; F: mycorrhizal frequency; Caro: carotenoid; Chl a: chlorophyll a; Chl b: chlorophyll b; Protein (Lv): protein in leaves; Protein(Fr): protein in fruits; TSS (Lv): total soluble sugar in leaves; TSS (Fr): total soluble sugar in fruits; H2O2 (Lv): hydrogen peroxide in leaves; H2O2 (Fr): hydrogen peroxide in fruits; MDA (Lv): malondialdehyde in leaves; MDA (Fr): malondialdehyde in fruits; POX (Lv): peroxidase activity in leaves; POX (Fr): peroxidase activity in fruits; PPO (Lv): polyphenol oxidase activity in leaves; PPO (Fr): polyphenol oxidase activity in fruits
3.8 Principal Component Analysis and Heat Mapping for Evaluated Traits
Principal Component Analysis (PCA) was conducted to explore the distribution of agro-physiological as well as biochemical traits based on the treatments applied under two water regimes: well-watered (WW), and drought stress (DS) conditions (Fig. 7a). The analysis utilized two principal components, which collectively accounted for 76.29% of the total variability, with PC1 explaining 63.72% and PC2 accounting for 12.67%. PC1 effectively distinguished between the treatments based on the intensity of water stress, positioning the DS treatments on the left side and the WW treatments on the right side of the biplot. Under WW conditions, the PCA indicated a strong positive correlation between the C+AMF treatment and several growth parameters, including root length, shoot height, root dry weight, and shoot dry weight. Additionally, this treatment was positively associated with physiological metrics such as chlorophyll fluorescence, and stomatal conductance. Furthermore, PCA revealed significant positive correlations between C+AMF and water-related indicators, such as leaf water potential and relative water content, as well as with photosynthetic pigments (carotenoids, chlorophyll a, and chlorophyll b) and osmolytes content (protein and sugar) in both leaves and fruits. Conversely, the biplot analysis highlighted a positive correlation between the control and NPK treatments under DS conditions with elevated levels of stress markers, including hydrogen peroxide as well as malondialdehyde in leaves and fruits. The PCA also demonstrated a strong positive correlation between the antioxidant enzyme activities (polyphenol oxidase and peroxidase) in leaves and fruits and the application of AMF, either alone or in combination with compost (C+AMF). This suggests that AMF treatment, especially when combined with compost, plays a significant role in mitigating oxidative stress and enhancing plant resilience under DS.
Figure 7: Principal component (a) and Hierarchical grouping and heat map analysis (b) illustrating the associations between the treatments applied (a and b, scale on right) under the two water regimes WW and DS and the grouping of the different features studied (I, II, III, IV and V, scale at top). The different colors and intensities have been adjusted according to the treatment-treatment relationships. Dark blue indicates lower values (drought-sensitive), while dark green indicates higher values (drought-tolerant). RL: root length; SH: shoot height; SDW: shoot dry weight; RDW: root dry weight; gs: stomatal conductance; Fv/Fm: chlorophyll fluorescence; RWC: relative water content; LWP: leaf water potential; F: mycorrhizal frequency; I: mycorrhizal intensity; Caro: carotenoid; Chl a: chlorophyll a; Chl b: chlorophyll b; Protein (Lv): protein in leaves; Protein (Fr): protein in fruits; TSS (Fr): total soluble sugar in fruits; TSS (Lv): total soluble sugar in leaves; H2O2 (Lv): hydrogen peroxide in leaves; H2O2 (Fr): hydrogen peroxide in fruits; MDA (Lv): malondialdehyde in leaves; MDA (Fr): malondialdehyde in fruits; POX (Lv): peroxidase activity in leaves; POX(Fr): peroxidase activity in fruits; PPO (Lv): polyphenol oxidase activity in leaves; PPO (Fr): polyphenol oxidase activity in fruits
To further investigate the variability among the different treatments, hierarchical clustering analysis (HCA) was conducted (Fig. 7b). This analysis revealed two principal clusters, designated as Group A and Group B. Group A is subdivided into two main subgroups: i) DS Control and DS NPK; ii) WW Control, DS C, DS AMF, and DS C+AMF. Group B is also divided into two subgroups: iii) WW AMF, WW C+AMF, and WW C; iv) WW NPK. Under DS conditions, treatments primarily clustered into subgroups i and ii, with the exception of WW Control. Conversely, under WW conditions, the treatments formed distinct clusters in subgroups iii and iv. The HCA demonstrated that all applied treatments, compared to the conditions, enhanced various morphological, physiological as well as biochemical traits of the tomato plants, irrespective of whether the plants were under WW or DS conditions. The HCA identified two main groups of interest: Group I and II, which are associated with antioxidant enzyme activities (PPO and POX) and stress markers (MDA and H2O2). These groups encompass significant morphological, physiological, and growth parameters. Meanwhile, Groups III, IV, and V focus on key growth metrics (SH, RL, RDW, and SDW), physiological indicators (gs, ΨLeaf, RWC, Fv/Fm, and chlorophyll content, and biochemical parameters (protein and sugar contents). Under drought stress (DS) conditions, plants treated with AMF and/or compost demonstrated moderate growth, physiological, and biochemical responses compared to control plants under well-watered conditions. Conversely, plants treated with NPK displayed elevated levels of MDA and H2O2 in both leaves and fruits, resembling those of control plants under DS conditions. This indicates that while AMF and compost treatments provide some resilience under stress, NPK treatment exacerbates oxidative stress, affecting the overall plant health.
Drought remains a major limitation to crop growth, physiology, and yield worldwide. Furthermore, the overreliance on conventional chemical fertilizers has negatively impacted the physicochemical and biological properties of soils, causing significant disturbances in plant agro-physiological and biochemical processes, especially under drought stress. Addressing this issue requires the development of innovative strategies that enhance plant resilience to drought while ensuring agricultural productivity and sustainability to support global food security. Natural biostimulants, such as AMF and compost, are emerging as viable and eco-friendly alternatives to chemical fertilizers. These biostimulants are increasingly valued for their potential to improve crop tolerance and productivity in drought conditions. This study aimed to evaluate the effects of AMF and compost, both individually and in combination with chemical fertilizers, on the agro-physio-biochemical characteristics of tomato leaves and fruits under drought stress. This investigation is notable as it provides a comprehensive comparison of the impacts of AMF alone and its combination with compost against conventional chemical fertilizers on tomato plant growth, physiological responses, and biochemical attributes under drought stress.
4.1 Mycorrhizal Status and Tomato Growth
Drought significantly impairs the growth of tomato plants, particularly when they are not treated with AMF or compost. Numerous studies have documented that drought stress adversely affects tomato plants by reducing growth, physiological functions, biomass, and yield [42,43]. Specifically, drought can lead to decreases in shoot height, leaf number and area, chlorophyll content, photosynthetic efficiency, stomatal conductance, leaf water potential, plant quantum yield, and fruit yield [15,44–47]. Omena-Garcia et al. [48] observed that drought stress resulted in substantial reductions in shoot dry weight, root dry weight, total leaf area, and specific leaf area in tomatoes compared to well-watered plants. The findings of this study indicate that applying compost, either alone or combined with R. irregularis, significantly improved plant height, root length, shoot and root biomass, as well as the number and fresh weight of tomato fruits, compared to the control under both drought stress (DS) and well-watered (WW) conditions. Tomato plant growth was notably enhanced in nutrient-rich soils treated with chemical fertilizers (NPK), showing performance comparable to that achieved with natural biostimulants (AMF and compost), especially under well-watered conditions. Additionally, Abdelhady et al. [49] found that conventional NPK fertilizers resulted in the highest growth parameters for tomato plants under WW, whereas the lowest values were observed under drought stress. Ahanger et al. [50] demonstrated that both compost alone and its combination with AMF significantly enhanced tomato growth and biomass accumulation compared to the control plants under DS. Tahiri et al. [51] also found that the combination of AMF and compost led to a significant enhancement in tomato shoot biomass, achieving an impressive increase of 156% compared to the control plants subjected to drought stress (DS). Fuertes-Mendizábal et al. [52] observed that plants treated with lower concentrations of compost exhibited higher leaf dry weights compared to those fertilized with NPK. Singh et al. [53] also reported that water stress considerably diminished total shoot dry weights, with inorganic fertilizers resulting in 10%–30% greater reductions in plant biomass compared to organic fertilizers.
The observed improvements in plant growth and yield with compost and AMF applications are attributed to enhanced nutrient and water uptake, facilitated by the extra-radical hyphae of AMF. These hyphae can access phosphorus from non-rhizosphere soil, bypassing the slow diffusion process typically encountered by plant roots. The increased availability of nutrients from compost and the enhanced nutrient and water absorption through AMF likely contributed to the observed growth benefits under drought stress [10,54]. Plants can effectively access phosphorus from organic pools in compost once it has undergone mineralization [55–58]. Additionally, AMF play a fundamental role in the solubilization of key nutrients such as potassium, phosphorus, and iron [58]. They also contribute to the biodegradation and mineralization of soil organic matter, enhance phytohormones biosynthesis, and improve soil structure and aggregation [59]. The presence of AMF in the rhizosphere can stimulate the synthesis of aquaporins, thereby enhancing water uptake and maintaining ion homeostasis under drought stress conditions [19,60,61]. In contrast, chemical fertilizers can potentially alter soil water potential, which may impair root efficiency in water and nutrient absorption, especially under water stress [62,63]. AMF species, particularly R. irregularis, are known for their high root infection rates, effective nutrient cycling, and efficient phosphorus supply to plant roots [64]. The incorporation of compost-derived materials into the soil also provides a crucial energy source for beneficial microorganisms, including AMF, particularly in soils with low organic content [65]. Compost not only supplies essential nutrients and supports plant and microbial growth but also positively impacts soil structure, physicochemical properties, and aeration, offering several benefits over conventional NPK fertilizers [66].
The enhancement of soil structure and porosity may be attributed to the humification effects of the green waste-based compost used in this study. Roldán et al. [67] suggested that combining compost with mycorrhizal inoculation positively influences the stability of soil aggregates, thanks to the role of polysaccharides, which can lead to improved root development under DS. The interaction between compost and AMF can also enhance nutrient utilization efficiency by promoting cell division and growth performance [58]. Benaffari et al. [46] found that the application of various compost types combined with AMF increased quinoa yield more effectively than compost alone under water stress conditions. It is noteworthy that the application of compost had a synergistic effect on both plant growth and the colonization of roots by AMF under drought stress conditions. However, drought conditions significantly reduce root colonization and hyphal growth, highlighting the challenge of maintaining effective mycorrhizal associations during periods of water scarcity [68–70].
Environmental factors such as soil moisture, temperature, and nutrient availability have a direct impact on root colonization by AMF [71,72]. Our study demonstrates that the application of green waste compost significantly boosted AMF spore production, leading to an increased presence of infectious propagules in tomato plants. This enhancement may be due to the effective sporulation capabilities of AMF or the beneficial interactions facilitated by the organic matter available in the soil. These observations are consistent with findings from Anli et al. [73], who reported that compost application positively affects AMF growth, especially under drought stress conditions. Several studies have shown that water stress adversely affects spore germination, AMF survival, and hyphal growth within host roots [68,74,75]. The presence of AMF in arid environments can upregulate genes involved in nutrient transport, which improves nutrient absorption and movement to the host plant [76]. These mechanisms are essential for promoting root development and overall plant growth in difficult conditions [77,78].The incorporation of humic acid-rich organic matter has been shown to stimulate both root colonization and the growth of extra-radical hyphae in the soil [67,79]. Our results indicate that compost application maintained adequate levels of phosphorus, nitrogen, potassium, and soil moisture. This not only supported effective root colonization when combined with AMF but also improved the water status of tomato plants under drought stress conditions [67].
4.2 Leaf Water Potential and Relative Water Content
In our research, tomato plants that received AMF inoculation and/or were supplemented with compostdemonstrated significantly improved leaf water status under DS compared to plants that were either not amended or not inoculated, including those receiving only NPK fertilizer. This indicates the critical role of biostimulants, whether applied individually or in combination, in enhancing water absorption and transport from the soil to the plants during stressful conditions. The study highlights that AMF hyphae can significantly boost soil water use efficiency, enhancing both water and nutrient absorption in plants experiencing water stress [12,25,26,47,80]. The addition of organic amendments improves soil water retention and infiltration rates, as well as soil structure and fertility [81,82]. Notably, plants treated with AMF alone or in alongside with compost exhibited superior leaf water status compared to those treated with NPK fertilizers or left untreated, particularly under drought stress. This suggests that natural biostimulants are more effective than chemical fertilizers in improving the water status of tomato plants under stress conditions. The enhanced water uptake observed in AMF-treated plants may be attributed to the increased nutrient availability from soil organic amendments and the extensive hyphal networks that extend beyond the root zone [25]. These resultsare consistent with studies by Boutasknit et al. [15], who also reported that combining AMF with compost improves plant water status under drought conditions. Conversely, while chemical fertilizers enhance soil nutrient content, they do not significantly improve soil water retention or the efficiency of water and nutrient absorption under dry conditions [83]. Hashem et al. [25] found that organic amendments, whether applied alone or in combination with AMF, enhance water uptake by facilitating water movement to the plant’s evaporative surfaces and increasing stomatal opening under drought condition.
4.3 Stomatal Conductance, Chlorophyll Fluorescence, and Photosynthetic Pigments
Under DS, plants treated with AMF and/or supplemented with organic materials demonstrate a significantly improved ability to regulate water uptake, manage stomatal function, and optimize water distribution within their tissues [84]. The opening and closing of stomata is a crucial strategy employed by plants to reduce water loss and adapt to drought stress [85]. Our study revealed that stomatal conductance was notably reduced in DS-plants compared to those under optimal irrigation. However, plants treated with AMF, either alone or in combination with compost, showed a marked improvement in stomatal conductance. This enhancement is likely due to several factors, including increased hydraulic conductance, better osmotic adjustment, a larger root absorptive surface area, and improved mycorrhizal colonization [58,85,86]. The results indicate that plants with AMF inoculation and/or compost amendment exhibited higher mycorrhizal colonization, which appears to positively influence their water status, as evidenced by less negative leaf water potential (ΨLeaf) values under drought conditions.
Generally, plants treated with AMF and/or compost maintained better stomatal conductance compared to those treated with chemical fertilizers (NPK) or left untreated. Improved water status and enhanced stomatal opening in these plants contribute to better photosynthetic efficiency and quantum yield of photosystem II (PSII) under water stress. Specifically, the combined application of AMF and compost resulted in higher Fv/Fm values in tomato plants under DS compared to the controls and those treated with NPK fertilizers. These findings align with other research showing that AMF inoculation can enhance PSII quantum yield under drought conditions [44,47,87]. Under DS, Gong et al. [88] reported that the inoculation of Sophora davidii plants with AMF led to a significant enhancement in the quantum yield of photosystem II (Fv/Fm) compared to non-inoculated plants. This enhancement is attributed to improved efficiency in capturing xcitation energy and the minimized damage to the reaction centers of photosystem II, especially in conditions of drought stress [89,90].
Previous studies have shown that water deficit often results in oxidative stress, leading to the degradation of photosynthetic pigments such as chlorophyll and carotenoids due to photooxidation [91,92]. Our findings indicate that chlorophyll a, chlorophyll b, and carotenoid levels significantly decreased under DS compared to WW plants, which contrasts with some studies that reported increased carotenoid content under similar conditions [93]. Research has demonstrated that compost application can notably improve the chlorophyll and carotenoid content in plants subjected to DS [12,13,94,95]. Consistent with these findings, our study showed that AMF inoculation, either alone or in combination with compost, preserved higher levels of chlorophyll and carotenoids compared to plants treated with NPK fertilizers or those left untreated. Fallah et al. [96] observed that organic amendments, including compost, enhance soil nitrogen availability, which supports chlorophyll production and improves photosynthetic efficiency under water stress. The ability of compost to boost chlorophyll content is likely due to its role in enhancing root colonization by AMF and improving the plant’s water retention capabilities [96,97]. In addition, the compost-amended soil promoted a high water potential that allowed stomata to open, resulting in increased carbon assimilation and chlorophyll content under abiotic stress. Furthermore, the addition of NPK also boosts the soil’s nitrogen content, contributing to improved chlorophyll and carotenoid content, but is still less performing than the application of biostimulants, especially under water stress conditions.
4.4 Quantification of Organic Osmolytes (Total Soluble Sugars and Proteins)
The advantages of applying compost and/or AMF extend beyond improvements in water and mineral uptake and chlorophyll content. These benefits also include increased levels of sugars and proteins [47,60]. Consequently, plants that are treated with compost and AMF AMF may exhibit greater physiological resilience to water stress and more effective osmotic regulation compared to those treated with NPK fertilizers or left untreated. Hammad et al. [62] reported that the combination of compost and AMF significantly increased sugar and protein concentrations in plants under DS. The elevated sugar as well as protein levels observed in tomato plants treated with compost and/or AMF suggest that these treatments improve the plant’s metabolic activity and stress resilience compared to those receiving chemical fertilizers or no treatment. Indeed, the accumulation of sugar and protein contents in the leaves and fruits of plants under water stress situations reduced osmotic potential in host cells. The sugar and protein levels in the leaves and fruits of tomato plants treated with compost and/or AMF were elevated, indicating that the natural metabolism of untreated and uninoculated plants (controls and those receiving NPK) was less active under water stress conditions [51].
4.5 Quantification of Hydrogen Peroxide (H2O2) and Malondialdehyde (MDA) Content
The elevated protein levels in tomato plants subjected to AMF and compost treatments may contribute to the reinforcement of the non-enzymatic antioxidant protection system and the reduction of harmful effects linked with the buildup of reactive oxygen species (ROS) [98]. The reduction in H2O2 concentration in the leaves and fruits of plants treated with AMF as well as compost, either separately or in combination, may be attributed to the upregulation of antioxidant enzyme genes under water stress conditions. Additionally, membrane lipids are highly vulnerable to oxidative damage due to elevated ROS production when plants are subjected to environmental stresses [99,100]. Accumulation of ROS levels at the cellular level in plants under water stress generally leads to oxidation of membrane lipids, producing high levels of MDA, and decreasing the membrane stability of plant cells [12]. Our results confirmed that the application of DS increased the MDA content in relation to the high production of hydrogen peroxide (H2O2) especially in non-inoculated and non-amended plants (control and NPK). While AMF-inoculated tomato plants showed low production of MDA and H2O2 content under water stress compared to plants under favorable conditions. Similar findings indicated a positive correlation between reduced H2O2 and MDA levels and improved DS tolerance in plants that were treated with compost and AMF [101].
4.6 Antioxidant Enzymes Contents
The AMF application, whether used alone or combined with compost, can mitigate elevated H2O2 levels and play a crucial role in shielding membranes from oxidative damage under DS. Indeed, He et al. [102] showed that AMF have the capacity to stimulate the expression of peroxidase (POX) genes that facilitate H2O2 removal. In addition, AMF showed an ability to modulate aquaporin gene expression to transfer H2O and H2O2 in plants under water stress [103,104]. Plants defend against oxidative stress-induced damage through ROS detoxification mechanisms, which may include enzymatic processes. He et al. [102] observed similar findings, noting increased antioxidant enzyme activity in plants under DS. This enhancement was more significant in the leaves and fruits of plants treated with compost and/or AMF compared to those treated with NPK fertilizer or left untreated. Anli et al. [44] demonstrated that the application of compost alone or in combination with AMF mitigated the effects of DS by elevating levels of polyphenol oxidase and peroxidase while reducing malondialdehyde and hydrogen peroxide compared to the control plants. Various research have indicated that enhanced plant tolerance to water stress is achieved by improving cell membrane stability and elasticity—thereby minimizing membrane damage—through the activation of the antioxidant defense coordination [12,105–107].
In summary, drought stress (DS) considerably affects the growth and biomass production of tomato plants. Nonetheless, the application of natural biostimulants like AMF and green waste compost, has shown superior performance compared to traditional NPK fertilizers and untreated plants. Plants inoculated with R. irregularis and amended with 5% green waste compost—either individually or in combination—demonstrated enhanced tolerance to DS.
This improvement can be attributed to superior osmotic adjustment, characterized by elevated sugar and protein levels, and increased antioxidant activity, marked by higher peroxidase and polyphenol oxidase activities. The synergistic application of AMF as well as compost significantly boosted osmolyte accumulation, which in turn improved water relations, facilitated stomatal opening, and reduced lipid peroxidation, as evidenced by lower malondialdehyde levels.
Consequently, these treatments led to improved aerial and root growth, along with enhanced quantum yield of photosystem II under drought stress conditions. This suggests that the synergistic effect of combining AMF with compost can be a viable strategy for enhancing growth, yield, and drought resilience in tomato crops while reducing reliance on conventional chemical fertilizers and promoting sustainable organic agriculture.
Looking ahead, we recommend further research to evaluate the effectiveness of this biostimulant combination under field conditions. Additionally, investigating the molecular mechanisms underlying drought stress alleviation facilitated by AMF and compost could provide deeper insights into optimizing these practices for improved crop resilience and productivity.
Acknowledgement: None.
Funding Statement: The authors did not receive any specific financial support for this study.
Author Contributions: Abderrahim Boutasknit, Wissal Benaffari, Mohamed Anli, Abdoussadeq Ouamnina and Amine Assouguem conducted the experiments, contributed to the design and development of the research, performed the analyses, interpreted the data, and authored the manuscript. Abdelilah Meddich was responsible for designing and supervising the research. Rachid Lahlali and Abdelilah Meddich revised and finalized the manuscript. All authors reviewed the results and approved the final version of the manuscript.
Availability of Data and Materials: All data generated or analyzed during this study are included in this published article.
Ethics Approval: This article does not include any research involving human or animal subjects.
Conflicts of Interest: The authors declare no conflicts of interest to report regarding the present study.
References
1. Tumanyan A, Gertrude K, Nadezhda Z, Bayat M. Impact of agrochemicals on the productivity of various tomato cultivars. Res Crops. 2020;21(2):301–5. doi:10.31830/2348-7542.2020.052. [Google Scholar] [CrossRef]
2. Berni R, Romi M, Cantini C, Hausman JF, Guerriero G, Cai G. Functional molecules in locally-adapted crops: the case study of tomatoes, onions, and sweet cherry fruits from tuscany in Italy. Front Plant Sci. 2019;9:1–8. doi:10.3389/fpls.2018.01983. [Google Scholar] [PubMed] [CrossRef]
3. Gürbüz ÇN, Eken NT, Ülger M, Frary A, Doğanlar S. Mapping of quantitative trait loci for antioxidant molecules in tomato fruit: carotenoids, vitamins C and E, glutathione and phenolic acids. Plant Sci. 2020;292(2016):110393. doi:10.1016/j.plantsci.2019.110393. [Google Scholar] [PubMed] [CrossRef]
4. Nadeem M, Li J, Yahya M, Sher A, Ma C, Wang X, et al. Research progress and perspective on drought stress in legumes: a review. Int J Mol Sci. 2019;20(10):2541. doi:10.3390/ijms20102541. [Google Scholar] [PubMed] [CrossRef]
5. Lorenz K, Lal R, Ehlers K. Soil organic carbon stock as an indicator for monitoring land and soil degradation in relation to United Nations’ Sustainable Development Goals. Land Degradation Dev. 2019;30(7):824–38. doi:10.1002/ldr.3270. [Google Scholar] [CrossRef]
6. Han J, Dong Y, Zhang M. Chemical fertilizer reduction with organic fertilizer effectively improve soil fertility and microbial community from newly cultivated land in the Loess Plateau of China. Appl Soil Ecol. 2021;165(2):103966. doi:10.1016/j.apsoil.2021.103966. [Google Scholar] [CrossRef]
7. Chukwudi UP, Kutu FR, Mavengahama S. Heat stress effect on the grain yield of three drought-tolerant maize varieties under varying growth conditions. Plants. 2021;10(8):1–15. doi:10.3390/plants10081532. [Google Scholar] [PubMed] [CrossRef]
8. Liu X, Wei Z, Ma Y, Liu J, Liu F. Effects of biochar amendment and reduced irrigation on growth, physiology, water-use efficiency and nutrients uptake of tobacco (Nicotiana tabacum L.) on two different soil types. Sci Total Environ. 2021;770(3):144769. doi:10.1016/j.scitotenv.2020.144769. [Google Scholar] [PubMed] [CrossRef]
9. Wei Z, Wang JJ, Fultz LM, White P, Jeong C. Application of biochar in estrogen hormone-contaminated and manure-affected soils: impact on soil respiration, microbial community and enzyme activity. Chemosphere. 2021;270(1):128625. doi:10.1016/j.chemosphere.2020.128625. [Google Scholar] [PubMed] [CrossRef]
10. Armada E, Portela G, Roldán A, Azcón R. Combined use of beneficial soil microorganism and agrowaste residue to cope with plant water limitation under semiarid conditions. Geoderma. 2014;232(4):640–8. doi:10.1016/j.geoderma.2014.06.025. [Google Scholar] [CrossRef]
11. Zhang L, Sun X. Influence of bulking agents on physical, chemical, and microbiological properties during the two-stage composting of green waste. Waste Manag. 2016;48:115–26. doi:10.1016/j.wasman.2015.11.032. [Google Scholar] [PubMed] [CrossRef]
12. Boutasknit A, Ait-Rahou Y, Anli M, Ait-El-Mokhtar M, Ben-Laouane R, Meddich A. Improvement of garlic growth, physiology, biochemical traits, and soil fertility by Rhizophagus irregularis and compost. Gesunde Pflanzen. 2021;73(2):149–60. doi:10.1007/s10343-020-00533-3. [Google Scholar] [CrossRef]
13. Abd El-Mageed TA, El-Sherif AMA, Abd El-Mageed SA, Abdou NM. A novel compost alleviate drought stress for sugar beet production grown in Cd-contaminated saline soil. Agric Water Manag. 2019;226(1):105831. doi:10.1016/j.agwat.2019.105831. [Google Scholar] [CrossRef]
14. Purwanto BH, Wulandari P, Sulistyaningsih E, Utami SNH, Handayani S. Improved corn yields when humic acid extracted from composted manure is applied to acid soils with phosphorus fertilizer. Appl Environ Soil Sci. 2021;2021:1–12. doi:10.1155/2021/8838420. [Google Scholar] [CrossRef]
15. Boutasknit A, Baslam M, Anli M, Ait-El-Mokhtar M, Ben-Laouane R, Ait-Rahou Y, et al. Impact of arbuscular mycorrhizal fungi and compost on the growth, water status, and photosynthesis of carob (Ceratonia siliqua) under drought stress and recovery. Plant Biosyst. 2022;156(4):1010. doi:10.1080/11263504.2021.1985006. [Google Scholar] [CrossRef]
16. Duri LG, El-Nakhel C, Caporale AG, Ciriello M, Graziani G, Pannico A, et al. Mars regolith simulant ameliorated by compost as in situ cultivation substrate improves lettuce growth and nutritional aspects. Plants. 2020;9(5):1–17. doi:10.3390/plants9050628. [Google Scholar] [PubMed] [CrossRef]
17. Jabborova D, Annapurna K, Al-Sadi AM, Alharbi SA, Datta R, Zuan ATK. Biochar and Arbuscular mycorrhizal fungi mediated enhanced drought tolerance in Okra (Abelmoschus esculentus) plant growth, root morphological traits and physiological properties. Saudi J Biol Sci. 2021;2(10):5490–9. doi:10.1016/j.sjbs.2021.08.016. [Google Scholar] [PubMed] [CrossRef]
18. Kaur S, Suseela V. Unraveling arbuscular mycorrhiza-induced changes in plant primary and secondary metabolome. Metabolites. 2020;10(8):335. doi:10.3390/metabo10080335. [Google Scholar] [PubMed] [CrossRef]
19. Quiroga G, Erice G, Aroca R, Chaumont F, Ruiz-Lozano JM. Contribution of the arbuscular mycorrhizal symbiosis to the regulation of radial root water transport in maize plants under water deficit. Environ Exp Bot. 2019;167:103821. doi:10.1016/j.envexpbot.2019.103821. [Google Scholar] [CrossRef]
20. Al-Arjani AF, Hashem A, Abd_Allah EF. Arbuscular mycorrhizal fungi modulates dynamics tolerance expression to mitigate drought stress in Ephedra foliata Boiss. Saudi J Biol Sci. 2020;27(1):380–94. doi:10.1016/j.sjbs.2019.10.008. [Google Scholar] [PubMed] [CrossRef]
21. Begum N, Ahanger MA, Zhang L. AMF inoculation and phosphorus supplementation alleviates drought induced growth and photosynthetic decline in Nicotiana tabacum by up-regulating antioxidant metabolism and osmolyte accumulation. Environ Exp Bot. 2020;176(9):104088. doi:10.1016/j.envexpbot.2020.104088. [Google Scholar] [CrossRef]
22. Yang Y, Tang M, Sulpice R, Chen H, Tian S, Ban Y. Arbuscular mycorrhizal fungi alter fractal dimension characteristics of Robinia pseudoacacia L. seedlings through regulating plant growth, leaf water status, photosynthesis, and nutrient concentration under drought stress. J Plant Growth Regul. 2014;33:612–25. doi:10.1007/s00344-013-9410-0. [Google Scholar] [CrossRef]
23. Zou YN, Wu QS, Kuča K. Unravelling the role of arbuscular mycorrhizal fungi in mitigating the oxidative burst of plants under drought stress. Plant Biol. 2021;23(S1):50–7. doi:10.1111/plb.13161. [Google Scholar] [PubMed] [CrossRef]
24. Chen W, Meng P, Feng H, Wang C. Effects of arbuscular mycorrhizal fungi on growth and physiological performance of Catalpa bungei C.A. Mey. under drought stress. Forests. 2020;11(10):1–29. doi:10.3390/f11101117. [Google Scholar] [CrossRef]
25. Hashem A, Kumar A, Al-Dbass AM, Alqarawi AA, Al-Arjani AF, Singh G, et al. Arbuscular mycorrhizal fungi and biochar improves drought tolerance in chickpea. Saudi J Biol Sci. 2019;26(3):614–24. doi:10.1016/j.sjbs.2018.11.005. [Google Scholar] [PubMed] [CrossRef]
26. Azizi S, Tabari Kouchaksaraei M, Hadian J, Fallah Nosrat Abad AR, Modarres Sanavi SAM, Ammer C, et al. Dual inoculations of arbuscular mycorrhizal fungi and plant growth-promoting rhizobacteria boost drought resistance and essential oil yield of common myrtle. For Ecol Manag. 2021;497:119478. doi:10.1016/j.foreco.2021.119478. [Google Scholar] [CrossRef]
27. López-Bucio J, Campos-Cuevas JC, Hernández-Calderón E, Velásquez-Becerra C, Farías-Rodríguez R, Macías-Rodríguez LI, et al. Bacillus megaterium rhizobacteria promote growth and alter root-system architecture through an auxin- and ethylene-independent signaling mechanism in Arabidopsis thaliana. Mol Plant Microbe Interact. 2007;20(2):207–17. doi:10.1094/MPMI-20-2-0207. [Google Scholar] [PubMed] [CrossRef]
28. Lanfranco L, Fiorilli V, Venice F, Bonfante P. Strigolactones cross the kingdoms: plants, fungi, and bacteria in the arbuscular mycorrhizal symbiosis. J Exp Bot. 2018;69(9):2175–88. doi:10.1093/jxb/erx432. [Google Scholar] [PubMed] [CrossRef]
29. Evelin H, Devi TS, Gupta S, Kapoor R. Mitigation of salinity stress in plants by arbuscular mycorrhizal symbiosis: current understanding and new challenges. Front Plant Sci. 2019;10:470. doi:10.3389/fpls.2019.00470. [Google Scholar] [PubMed] [CrossRef]
30. Caravaca F, Alguacil MM, Azcón R, Parladé J, Torres P, Roldán A. Establishment of two ectomycorrhizal shrub species in a semiarid site after in situ amendment with sugar beet, rock phosphate, and Aspergillus niger. Microb Ecol. 2005;49(1):73–82. doi:10.1007/s00248-003-0131-y. [Google Scholar] [PubMed] [CrossRef]
31. Rivero J, Álvarez D, Flors V, Azcón-Aguilar C, Pozo MJ. Root metabolic plasticity underlies functional diversity in mycorrhiza-enhanced stress tolerance in tomato. New Phytol. 2018;220(4):1322–36. doi:10.1111/nph.15295. [Google Scholar] [PubMed] [CrossRef]
32. Elalaoui AC. Fertilisation minérale des cultures: les élèments fertilisants majeurs (N, P, K). Transfert de Technologie en Agriculture. 2007;155:4. [Google Scholar]
33. Meddich A, Elouaqoudi F, Khadra A, Bourzik W. Valorization of green and industrial waste by composting process. Revue des Composites et des Matériaux Avancés. 2016;26(3–4):451–69. doi:10.3166/RCMA.26.451-469. [Google Scholar] [CrossRef]
34. Phillips JM, Hayman DS. Improved procedures for clearing roots and staining parasitic and vesicular-arbuscular mycorrhizal fungi for rapid assessment of infection. Trans Br Mycol Soc. 1970;55(1):18–29. doi:10.1016/s0007-1536(70)80110-3. [Google Scholar] [CrossRef]
35. Barrs HD, Weatherley PE. A re-examination of the relative turgidity technique for estimating water deficits in leaves. Aust J Biol Sci. 1962;15:413–28. [Google Scholar]
36. Arnon D. Copper enzymes in isolated chloroplasts. Polyphenoloxidase in Beta vulgaris. Plant Physiol. 1949;24:1–15. doi:10.1104/pp.24.1.1. [Google Scholar] [PubMed] [CrossRef]
37. Dubois M, Gilles KA, Hamilton JK, Rebers PA, Smith F. Colorimetric method for determination of sugars and related substances. Anal Chem. 1956;28(3):350–6. doi:10.1021/ac60111a017. [Google Scholar] [CrossRef]
38. Tejera García NA, Olivera M, Iribarne C, Lluch C. Partial purification and characterization of a non-specific acid phosphatase in leaves and root nodules of Phaseolus vulgaris. Plant Physiol Biochem. 2004;42:585–91. doi:10.1016/j.plaphy.2004.04.004. [Google Scholar] [PubMed] [CrossRef]
39. Savicka M, Škute N. Effects of high temperature on malondialdehyde content, superoxide production and growth changes in wheat seedlings (Triticum aestivum L.). Ekologija. 2010;56(1):26–33. doi:10.2478/v10055-010-0004-x. [Google Scholar] [CrossRef]
40. Velikova V, Yordanov I, Edreva A. Oxidative stress and some antioxidant systems in acid rain-treated bean plants protective role of exogenous polyamines. Phys Rev. 2000;176(5):1709–14. doi:10.1103/PhysRev.176.1709. [Google Scholar] [CrossRef]
41. Gauillard F, Richard-Forget F, Nicolas J. New sprectrophotometric assay for polyphenol oxidase activity. Anal Biochem. 1993;215(1):59–65. doi:10.1006/abio.1993.1554. [Google Scholar] [PubMed] [CrossRef]
42. Conti V, Romi M, Parri S, Aloisi I, Marino G, Cai G, et al. Morpho—physiological classification of italian tomato cultivars (Solanum lycopersicum l.) according to drought tolerance during vegetative and reproductive growth. Plants. 2021;10:1826. doi:10.3390/PLANTS10091826/S1. [Google Scholar] [CrossRef]
43. Ors S, Ekinci M, Yildirim E, Sahin U, Turan M, Dursun A. Interactive effects of salinity and drought stress on photosynthetic characteristics and physiology of tomato (Lycopersicon esculentum L.) seedlings. S Afr J Bot. 2021;137(918):335–9. doi:10.1016/j.sajb.2020.10.031. [Google Scholar] [CrossRef]
44. Anli M, Baslam M, Tahiri A, Raklami A, Symanczik S, Boutasknit A, et al. Biofertilizers as strategies to improve photosynthetic apparatus, growth, and drought stress tolerance in the date palm. Front Plant Sci. 2020;11:1560. doi:10.3389/fpls.2020.516818. [Google Scholar] [PubMed] [CrossRef]
45. Boutasknit A, Ait-El-Mokhtar M, Fassih B, Ben-Laouane R, Wahbi S, Meddich A. Effect of arbuscular mycorrhizal fungi and rock phosphate on growth, physiology, and biochemistry of carob under water stress and after rehydration in vermicompost-amended soil. Metabolites. 2024;14(4):202. doi:10.3390/metabo14040202. [Google Scholar] [PubMed] [CrossRef]
46. Benaffari W, Boutasknit A, Anli M, Ait-El-mokhtar M, Ait-Rahou Y, Ben-Laouane R, et al. The native arbuscular mycorrhizal fungi and vermicompost-based organic amendments enhance soil fertility, growth performance, and the drought stress tolerance of quinoa. Plants. 2022;11(3):393. doi:10.3390/plants11030393. [Google Scholar] [PubMed] [CrossRef]
47. Boutasknit A, Baslam M, Ait-El-mokhtar M, Anli M, Ben-Laouane R, Douira A, et al. Arbuscular mycorrhizal fungi mediate drought tolerance and recovery in two contrasting Carob (Ceratonia siliqua L.) ecotypes by regulating stomatal, water relations, and (in)organic adjustments. Plants. 2020;9(1):80. doi:10.3390/PLANTS9010080. [Google Scholar] [PubMed] [CrossRef]
48. Omena-Garcia RP, Oliveira Martins A, Medeiros DB, Vallarino JG, Mendes Ribeiro D, Fernie AR, et al. Growth and metabolic adjustments in response to gibberellin deficiency in drought stressed tomato plants. Environ Exp Bot. 2019;159:95–107. doi:10.1016/j.envexpbot.2018.12.011. [Google Scholar] [CrossRef]
49. Abdelhady SA, Abu El-Azm NA, El-Kafafi E-S. Effect of deficit irrigation levels and NPK fertilization rates on tomato growth, yield and fruits quality. Middle East J Agric Res. 2017;06:587–604. [Google Scholar]
50. Ahanger MA, Qi M, Huang Z, Xu X, Begum N, Qin C, et al. Improving growth and photosynthetic performance of drought stressed tomato by application of nano-organic fertilizer involves up-regulation of nitrogen, antioxidant and osmolyte metabolism. Ecotoxicol Environ Saf. 2021;216(4):112195. doi:10.1016/j.ecoenv.2021.112195. [Google Scholar] [CrossRef]
51. Tahiri A-I, Meddich A, Raklami A, Alahmad A, Bechtaoui N, Anli M, et al. Assessing the potential role of compost, PGPR, and AMF in improving tomato plant growth, yield, fruit quality, and water stress tolerance. J Soil Sci Plant Nutr. 2022;22(1):743–64. doi:10.1007/s42729-021-00684-w. [Google Scholar] [CrossRef]
52. Fuertes-Mendizábal T, Huérfano X, Ortega U, González-Murua C, Estavillo JM, Salcedo I, et al. Compost and pgp-based biostimulant as alternative to peat and NPK fertilization in chestnut (Castanea sativa mill.) nursery production. Forests. 2021;12(7):850. doi:10.3390/f12070850. [Google Scholar] [CrossRef]
53. Singh AK, Zhu X, Chen C, Wu J, Yang B. The role of glomalin in mitigation of multiple soil degradation problems. Crit Rev Environ Sci Technol. 2020;52(9):1604–38. doi:10.1080/10643389.2020.1862561. [Google Scholar] [CrossRef]
54. Liu Q, Chen Y, Liu Y, Wen X, Liao Y. Coupling effects of plastic film mulching and urea types on water use efficiency and grain yield of maize in the Loess Plateau, China. Soil Tillage Res. 2016;157(z2):1–10. doi:10.1016/j.still.2015.11.003. [Google Scholar] [CrossRef]
55. Wu QS, Zou YN. Arbuscular mycorrhizas and stress tolerance of plants. In: Wu QS, editor. Arbuscular mycorrhizas and stress tolerance of plants. Singapore: Springer; 2017. p. 25–41. doi:10.1007/978-981-10-4115-0. [Google Scholar] [CrossRef]
56. Jan B, Ali A, Wahid F, Shah SNM, Khan A, Khan F. Effect of arbuscular mycorrhiza fungal inoculation with compost on yield and phosphorous uptake of berseem in alkaline calcareous soil. Am J Plant Sci. 2014;5(9):1359–69. doi:10.4236/ajps.2014.59150. [Google Scholar] [CrossRef]
57. Üstüner Ö, Raviv M, Medina S, Wininger S, Gadkar V, Badani H, et al. Evaluation of different compost amendments with am fungal inoculum for optimal growth of chives. Compost Sci Util. 2009;17(4):257–65. doi:10.1080/1065657X.2009.10702432. [Google Scholar] [CrossRef]
58. Ortuño MF, Lorente B, Hernández JA, Sánchez-Blanco MJ. Mycorrhizal inoculation on compost substrate affects nutritional balance, water uptake and photosynthetic efficiency in Cistus albidus plants submitted to water stress. Braz J Bot. 2018;41(2):299–310. doi:10.1007/s40415-018-0457-9. [Google Scholar] [CrossRef]
59. Fall AF, Nakabonge G, Ssekandi J, Founoune-Mboup H, Apori SO, Ndiaye A, et al. Roles of arbuscular mycorrhizal fungi on soil fertility: contribution in the improvement of physical, chemical, and biological properties of the soil. Front Fungal Biol. 2022;3:723892. doi:10.3389/ffunb.2022.723892. [Google Scholar] [PubMed] [CrossRef]
60. Quiroga G, Erice G, Aroca R, Delgado-Huertas A, Ruiz-Lozano JM. Elucidating the possible involvement of maize aquaporins and arbuscular mycorrhizal symbiosis in the plant ammonium and urea transport under drought stress conditions. Plants. 2020;9(2):1–18. doi:10.3390/plants9020148. [Google Scholar] [PubMed] [CrossRef]
61. Xie W, Hao Z, Zhou X, Jiang X, Xu L, Wu S, et al. Arbuscular mycorrhiza facilitates the accumulation of glycyrrhizin and liquiritin in Glycyrrhiza uralensis under drought stress. Mycorrhiza. 2018;28(3):285–300. doi:10.1007/s00572-018-0827-y. [Google Scholar] [PubMed] [CrossRef]
62. Hammad HM, Khaliq A, Abbas F, Farhad W, Fahad S, Aslam M, et al. Comparative effects of organic and inorganic fertilizers on soil organic carbon and wheat productivity under arid region. Commun Soil Sci Plant Anal. 2020;51(10):1406–22. doi:10.1080/00103624.2020.1763385. [Google Scholar] [CrossRef]
63. Iqbal A, He L, Ali I, Ullah S, Khan A, Khan A, et al. Manure combined with chemical fertilizer increases rice productivity by improving soil health, post-anthesis biomass yield, and nitrogen metabolism. PLoS One. 2020;15(10):1–24. doi:10.1371/journal.pone.0238934. [Google Scholar] [PubMed] [CrossRef]
64. Yurkov AP, Puzanskiy RK, Kryukov AA, Gorbunova AO, Kudriashova TR, Jacobi LM, et al. The role of Medicago lupulina interaction with rhizophagus irregularis in the determination of root metabolome at early stages of AM symbiosis. Plants. 2022;11:2338. [Google Scholar] [PubMed]
65. Hussain A, Ahmad M, Mumtaz MZ, Ali S, Sarfraz R, Naveed M, et al. Integrated application of organic amendments with Alcaligenes sp. AZ9 improves nutrient uptake and yield of maize (Zea mays). J Plant Growth Regul. 2020;39(3):1277–92. doi:10.1007/s00344-020-10067-7. [Google Scholar] [CrossRef]
66. Khalid AA, Tuffour HO, Bonsu M. Influence of Poultry manure and NPK fertilizer on hydraulic properties of a sandy soil in Ghana. Int J Sci Res Agric Sci. 2014;1(2):16–22. doi:10.12983/ijsras-2014-p0016-0022. [Google Scholar] [CrossRef]
67. Roldán A, Carrasco L, Caravaca F. Stability of desiccated rhizosphere soil aggregates of mycorrhizal Juniperus oxycedrus grown in a desertified soil amended with a composted organic residue. Soil Biol Biochem. 2006;38(9):2722–30. doi:10.1016/j.soilbio.2006.04.024. [Google Scholar] [CrossRef]
68. Wu F, Dong M, Liu Y, Ma X, An L, Young JPW, et al. Effects of long-term fertilization on AM fungal community structure and Glomalin-related soil protein in the Loess Plateau of China. Plant Soil. 2011;342:233–47. doi:10.1007/s11104-010-0688-4. [Google Scholar] [CrossRef]
69. Zou Y, Wu H, Giri B, Wu Q, Ku K. Mycorrhizal symbiosis down-regulates or does not change root aquaporin expression in trifoliate orange under drought stress. Plant Physiol Biochem. 2019;144:292–9. doi:10.1016/j.plaphy.2019.10.001. [Google Scholar] [PubMed] [CrossRef]
70. Huang YM, Srivastava AK, Zou YN, Ni QD, Han Y, Wu QS. Mycorrhizal-induced calmodulin mediated changes in antioxidant enzymes and growth response of drought-stressed trifoliate orange. Front Microbiol. 2014;5:682. doi:10.3389/fmicb.2014.00682. [Google Scholar] [PubMed] [CrossRef]
71. Wagner MR, Lundberg DS, Del Rio TG, Tringe SG, Dangl JL, Mitchell-Olds T. Host genotype and age shape the leaf and root microbiomes of a wild perennial plant. Nat Commun. 2016;7:12151. doi:10.1038/ncomms12151. [Google Scholar] [PubMed] [CrossRef]
72. IJdo M, Cranenbrouck S, Declerck S. Methods for large-scale production of AM fungi: past, present, and future. Mycorrhiza. 2011;21:1–16. doi:10.1007/s00572-010-0337-z. [Google Scholar] [PubMed] [CrossRef]
73. Anli M, Symanczik S, El Abbassi A, Ait-El-Mokhtar M, Boutasknit A, Ben-Laouane R, et al. Use of arbuscular mycorrhizal fungus Rhizoglomus irregulare and compost to improve growth and physiological responses of Phoenix dactylifera ‘Boufgouss’. Plant Biosyst—Int J Dealing Aspects Plant Biol. 2021;155(4):763–71. doi:10.1080/11263504.2020.1779848. [Google Scholar] [CrossRef]
74. Wu HH, Zou YN, Rahman MM, Ni QD, Wu QS. Mycorrhizas alter sucrose and proline metabolism in trifoliate orange exposed to drought stress. Sci Rep. 2017;7(1):1–10. doi:10.1038/srep42389. [Google Scholar] [PubMed] [CrossRef]
75. Giovannetti M, Avio L, Sbrana C. Fungal spore germination and pre-symbiotic mycelial growth-physiological and genetic aspects. In: Koltai H, Kapulnik Y, editors. Arbuscular mycorrhizas: physiology and function. Dordrecht, The Netherlands: Springer; 2010. p. 3–32. [Google Scholar]
76. Giovannini L, Palla M, Agnolucci M, Avio L, Sbrana C, Turrini A, et al. Arbuscular mycorrhizal fungi and associated microbiota as plant biostimulants: research strategies for the selection of the best performing inocula. Agronomy. 2020;10(1):1–14. doi:10.3390/agronomy10010108. [Google Scholar] [CrossRef]
77. Bender SF, Conen F, Van der Heijden MGA. Mycorrhizal effects on nutrient cycling, nutrient leaching and N2O production in experimental grassland. Soil Biol Biochem. 2015;80:283–92. doi:10.1016/j.soilbio.2014.10.016. [Google Scholar] [CrossRef]
78. Pankaj U, Kurmi A, Lothe NB, Verma RK. Influence of the seedlings emergence and initial growth of palmarosa (Cymbopogon martinii (Roxb.) Wats. var. Motia Burk) by arbuscular mycorrhizal fungi in soil salinity conditions. J Appl Res Med Aromat Plants. 2021;24(2):100317. doi:10.1016/j.jarmap.2021.100317. [Google Scholar] [CrossRef]
79. Pinos NQ, Louro Berbara RL, Elias SS, van Tol de Castro TA, García AC. Combination of humic substances and arbuscular mycorrhizal fungi affecting corn plant growth. J Environ Qual. 2019;48(6):1594–604. doi:10.2134/jeq2019.01.0035. [Google Scholar] [CrossRef]
80. Liu C, Liu F, Ravnskov S, Rubæk GH, Sun Z, Andersen MN. Impact of wood biochar and its interactions with mycorrhizal fungi, phosphorus fertilization and irrigation strategies on potato growth. J Agron Crop Sci. 2017;203(2):131–45. doi:10.1111/jac.12185. [Google Scholar] [CrossRef]
81. Sun D, Li K, Bi Q, Zhu J, Zhang Q, Jin C, et al. Effects of organic amendment on soil aggregation and microbial community composition during drying-rewetting alternation. Sci Total Environ. 2017;574:735–43. doi:10.1016/j.scitotenv.2016.09.112. [Google Scholar] [PubMed] [CrossRef]
82. Ullah N, Ditta A, Imtiaz M, Li X, Jan AU, Mehmood S, et al. Appraisal for organic amendments and plant growth-promoting rhizobacteria to enhance crop productivity under drought stress: a review. J Agron Crop Sci. 2021;207(5):783–802. doi:10.1111/jac.12502. [Google Scholar] [CrossRef]
83. Abuchenari A, Hardani K, Abazari S, Naghdi F, Ahmady Keleshteri M, Jamavari A, et al. Clay-reinforced nanocomposites for the slow release of chemical fertilizers and water retention. J Compos Compd. 2020;2(3):85–91. doi:10.29252/jcc.2.2.4. [Google Scholar] [CrossRef]
84. Zhu XC, Song FB, Liu SQ, Liu TD, Zhou X. Arbuscular mycorrhizae improves photosynthesis and water status of Zea mays L. under drought stress. Plant, Soil Environ. 2012;58:186–91. [Google Scholar]
85. Haffani S, Mezni M, Ben Nasri M, Chaibi W. Comparative leaf water relations and anatomical responses of three vetch species (Vicia narbonensis L., V. sativa L. and V. villosa Roth.) to cope with water stress. Crop Pasture Sci. 2017;68:691–702. doi:10.1071/CP17029. [Google Scholar] [CrossRef]
86. Fernández DA, Roldán A, Azcón R, Caravaca F, Bååth E. Effects of water stress, organic amendment and mycorrhizal inoculation on soil microbial community structure and activity during the establishment of two heavy metal-tolerant native plant species. Microb Ecol. 2012;63:794–803. doi:10.1007/s00248-011-9972-y. [Google Scholar] [PubMed] [CrossRef]
87. Pan J, Huang C, Peng F, Zhang W, Luo J, Ma S, et al. Effect of Arbuscular Mycorrhizal Fungi (AMF) and Plant Growth-Promoting Bacteria (PGPR) inoculations on Elaeagnus angustifolia L. in saline soil. Appl Sci. 2020;10(3):945. doi:10.3390/app10030945. [Google Scholar] [CrossRef]
88. Gong M, Tang M, Chen H, Zhang Q, Feng X. Effects of two Glomus species on the growth and physiological performance of Sophora davidii seedlings under water stress. New Forests. 2013;44(3):399–408. doi:10.1007/s11056-012-9349-1. [Google Scholar] [CrossRef]
89. Bagheri V, Shamshiri MH, Shirani H, Roosta HR. Effect of mycorrhizal inoculation on ecophysiological responses of pistachio plants grown under different water regimes. Photosynthetica. 2011;49(4):531–8. doi:10.1007/s11099-011-0064-5. [Google Scholar] [CrossRef]
90. Wu QS, Xia RX, Zou YN. Improved soil structure and citrus growth after inoculation with three arbuscular mycorrhizal fungi under drought stress. Eur J Soil Biol. 2008;44(1):122–8. doi:10.1016/j.ejsobi.2007.10.001. [Google Scholar] [CrossRef]
91. Mo Y, Wang Y, Yang R, Zheng J, Liu C, Li H, et al. Regulation of plant growth, photosynthesis, antioxidation and osmosis by an arbuscular mycorrhizal fungus in watermelon seedlings under well-watered and drought conditions. Front Plant Sci. 2016;7(454):644. doi:10.3389/fpls.2016.00644. [Google Scholar] [PubMed] [CrossRef]
92. Yooyongwech S, Samphumphuang T, Tisarum R, Theerawitaya C, Cha-Um S. Arbuscular mycorrhizal fungi (AMF) improved water deficit tolerance in two different sweet potato genotypes involves osmotic adjustments via soluble sugar and free proline. Sci Hortic. 2016;198:107–17. doi:10.1016/j.scienta.2015.11.002. [Google Scholar] [CrossRef]
93. Mibei EK, Ambuko J, Giovannoni JJ, Onyango AN, Owino WO. Carotenoid profiling of the leaves of selected African eggplant accessions subjected to drought stress. Food Sci Nutr. 2017;5(1):113–22. doi:10.1002/fsn3.370. [Google Scholar] [PubMed] [CrossRef]
94. Duo LA, Liu CX, Zhao SL. Alleviation of drought stress in Turfgrass by the combined application of nano-compost and microbes from compost. Russ J Plant Physiol. 2018;65:419–26. doi:10.1134/S102144371803010X. [Google Scholar] [CrossRef]
95. Bashir A, Rizwan M, Zia ur Rehman M, Zubair M, Riaz M, Qayyum MF, et al. Application of co-composted farm manure and biochar increased the wheat growth and decreased cadmium accumulation in plants under different water regimes. Chemosphere. 2020;246:125809. doi:10.1016/j.chemosphere.2019.125809. [Google Scholar] [PubMed] [CrossRef]
96. Fallah M, Hadi H, Amirnia R, Hassanzadeh-Ghorttapeh A, Zuan ATK, Sayyed RZ. Eco-friendly soil amendments improve growth, antioxidant activities, and root colonization in lingrain (Linum Usitatissimum L.) under drought conditions. PLoS One. 2021;16(12):e0261225. doi:10.1371/journal.pone.0261225. [Google Scholar] [PubMed] [CrossRef]
97. Valarini PJ, Curaqueo G, Seguel A, Manzano K, Rubio R, Cornejo P, et al. Effect of compost application on some properties of a volcanic soil from central South Chile. Chil J Agric Res. 2009;69(3):416–25. doi:10.4067/s0718-58392009000300015. [Google Scholar] [CrossRef]
98. Goel S. Comparative influence of fertilizer and vermicompost addition on growth and quality of tomato plant. Ecol Environ Conserv. 2021;27:1254–6. [Google Scholar]
99. Chandrasekaran M. Arbuscular mycorrhizal fungi mediated alleviation of drought stress via non-Enzymatic antioxidants: a meta-analysis. Plants. 2022;11(19):2448. doi:10.3390/plants11192448. [Google Scholar] [PubMed] [CrossRef]
100. Das K, Roychoudhury A. Reactive oxygen species (ROS) and response of antioxidants as ROS-scavengers during environmental stress in plants. Front Environ Sci. 2014;2:53. doi:10.3389/fenvs.2014.00053. [Google Scholar] [CrossRef]
101. Sharma P, Jha AB, Dubey RS, Pessarakli M. Reactive oxygen species, oxidative damage, and antioxidative defense mechanism in plants under stressful conditions. J Bot. 2012;2012:1–26. doi:10.1155/2012/217037. [Google Scholar] [CrossRef]
102. He JD, Zou YN, Wu QS, Kuča K. Mycorrhizas enhance drought tolerance of trifoliate orange by enhancing activities and gene expression of antioxidant enzymes. Sci Hortic. 2020;262:108745. doi:10.1016/j.scienta.2019.108745. [Google Scholar] [CrossRef]
103. Huang YM, Zou YN, Wu QS. Alleviation of drought stress by mycorrhizas is related to increased root H2O2 efflux in trifoliate orange. Sci Rep. 2017;7(1):1–9. doi:10.1038/srep42335. [Google Scholar] [PubMed] [CrossRef]
104. Kapilan R, Vaziri M, Zwiazek JJ. Regulation of aquaporins in plants under stress. Biol Res. 2018;51(1):1–11. doi:10.1186/s40659-018-0152-0. [Google Scholar] [PubMed] [CrossRef]
105. Jia-Dong H, Tao D, Hui-Hui W, Zou YN, Wu QS, Kamil K. Mycorrhizas induce diverse responses of root TIP aquaporin gene expression to drought stress in trifoliate orange. Sci Hortic. 2019;243:64–9. doi:10.1016/j.scienta.2018.08.010. [Google Scholar] [CrossRef]
106. Khalafallah AA, Abo-Ghalia HH. Effect of arbuscular mycorrhizal fungi on the metabolic products and activity of antioxidant system in wheat plants subjected to short-term water stress, followed by recovery at different growth stages. J Appl Sci Res. 2008;4:559–69. [Google Scholar]
107. Murshed R, Lopez-Lauri F, Sallanon H. Effect of water stress on antioxidant systems and oxidative parameters in fruits of tomato (Solanum lycopersicon L, cv. Micro-tom). Physiol Mol Biol Plants. 2013;19(3):363–78. doi:10.1007/s12298-013-0173-7. [Google Scholar] [PubMed] [CrossRef]
Cite This Article
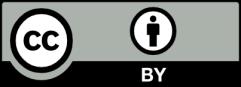
This work is licensed under a Creative Commons Attribution 4.0 International License , which permits unrestricted use, distribution, and reproduction in any medium, provided the original work is properly cited.