Open Access
REVIEW
Lignan Enhancement: An Updated Review on the Significance of Lignan and Its Improved Production in Crop Plants
1 Department of Botany, School of Chemical and Life Sciences, Jamia Hamdard University, New Delhi, 11062, India
2 Centre of Excellence in Unani Medicine (Pharmacognosy & Pharmacology), Bioactive Natural Product Laboratory (BNPL), Department of Pharmacognosy & Phytochemistry, School of Pharmaceutical Education & Research, Jamia Hamdard University, New Delhi, 110062, India
* Corresponding Authors: Noushina Iqbal. Email: ; Shahid Umar. Email:
(This article belongs to the Special Issue: Influence of Biotic and Abiotic Stresses Signals on Plants and their Performance at Different Environments)
Phyton-International Journal of Experimental Botany 2024, 93(12), 3237-3271. https://doi.org/10.32604/phyton.2024.057189
Received 10 August 2024; Accepted 25 November 2024; Issue published 31 December 2024
Abstract
Lignans are a prominent group of phenolic compounds ubiquitously present in the plant kingdom, playing a critical role in both plant physiology and human health. Structurally they are characterized by the dimerization of two phenylpropane units to attain diverse chemical configurations that contribute to their wide range of biological activities. In plants, lignans function primarily as defense molecules, protecting against pathogens, herbivores, and environmental stressors. These compounds also participate in plant growth regulation and lignification processes. From a nutritional and medicinal perspective, lignans are valued for their significant health benefits. They act as phytoestrogens, which can modulate estrogen receptors in humans, thereby offering protective effects against hormone-related diseases such as breast cancer and menopausal symptoms. Additionally, lignans possess potent antioxidant and anti-inflammatory properties, contributing to cardiovascular health and cancer prevention. This review aims to provide a comprehensive overview of the importance of lignans in plants, highlighting their chemical diversity, health-promoting properties and stress adaptation. By synthesizing recent research findings, this review underscores the significance of lignans in enhancing plant resilience under stress and human health. It explores their potential applications in the food and pharmaceutical industries. Besides, it will also focus on the widely used method for enhancing the production of these high-value secondary metabolites in plant cell cultures to meet their requirement in pharmaceutical industries.Keywords
List of Abbreviations
AG | Astragalosides |
AHP | Anhydropodorhizol |
ALA | α-linolenic acid |
ANHSEC | Anhydrosecoisolariciresinol |
BA | Betulinic acid |
BHA | Butylated hydroxyanisole |
BHT | Butylated hydroxytoluene |
CA | Cinnamic acid |
CAD | Cinnamyl alcohol dehydrogenase |
CAT | Catalase |
CCR | Cinnamoyl-CoA reductase |
CHD | Coronary heart disease |
DCG | Dehydrodiconiferyl alcohol glucoside |
DOP | Deoxypodophyllotoxin |
END | Enterodiol |
ENL | Enterolactone |
GGCG | Guaiacylglycerol-β-coniferyl alcohol ether glucoside |
HPH | Hypophyllanthin |
HSQC | Heteronuclear Single Quantum Coherence |
LARI | Lariciresinol |
LDG | Lariciresinol diglucoside |
MAT | Matairesinol |
MED | Medioresinol |
MeJa | Methyl jasmonate |
MPTOX | Methoxypodophyllotoxin |
NAA | Naphthaleneacetic acid |
NH | Niranthin |
OA | Oleanolic acid |
PA | Phenylalanine |
PAL | Phenylalanine ammonio-lyase |
PCBER | Phenylcoumaran benzylic ether reductase |
PDDG | Pinoresinol di-β-d-glucoside |
PDG | Pinoresinol-β-d-glucoside |
PH | Phyllanthin |
PINO | Pinoresinol |
PT | Phyltetralin |
PTOX | Podophyllotoxin |
ROS | Reactive oxygen species |
SDG | Secoisolariciresinol diglucoside |
SECO | Secoisolariciresinol |
Ses | Sesamin |
SOD | Superoxide dismutase |
Syr | Syringaresinol |
UA | Ursolic acid |
WIKS | Wikstromol |
Lignans are secondary metabolites formed through the oxidative coupling of phenylpropanoid units [1]. These naturally occurring compounds are primarily found in plants, often as glycosides or oligomers [2]. Lignans are widespread across various plant species and possess several pharmacological properties, including antiviral, antimicrobial, antioxidant, antifungal, antifeeding, and insecticidal activities [2,3]. In plants, lignans serve a critical function in protecting against pests and pathogens while also supporting the plant’s overall growth and development. Over the years, hundreds of lignans have been identified in numerous plant species, and some of these compounds are converted by gut microbiota into enterolignans-such as enterolactone and enterodiol-which exhibit various beneficial biological effects, including antitumor, anti-estrogenic, and antioxidant activities [4].
Early research focused mainly on two lignans, secoisolariciresinol (SECO) and matairesinol (MAT), which were known to be metabolized into enterolignans. However, recent studies have expanded this understanding to include secoisolariciresinol diglucoside (SDG), the most prevalent lignan found in linseed. The gut microbiota also converts SDG into enterodiol and enterolactone. Asia, Europe, and the Mediterranean cultivate linseed, a herb particularly rich in SDG [5,6]. SDG is essentially the glycosylated form of SECO [7]. The pharmacological properties and commercial use of linseed are due to its high α-linolenic acid (ALA) content and a range of bioactive compounds, including lignan [8]. Lignans are also present in other foods, including grains, seeds, fruits, vegetables, coffee, wine, and tea. New enterolignan precursors such as arctigenin, lariciresinol (LARI), 7-hydroxy-matairesinol (MAT), syringaresinol, and pinoresinol (PINO) have also been recently discovered [9].
The concentration and bioactivity of lignans are influenced by several factors, including plant species, environmental conditions, and the stage of ripening. Environmental elements such as soil composition, rainfall, UV exposure, pathogenic infections, air pollution, mechanical injury, and temperature extremes can all impact the polyphenol content in plants. Additionally, cultivation methods such as greenhouse, field, organic, or biological practices can also affect lignan production. Ripening stages significantly influence the concentration of phenolic compounds and anthocyanins. Beyond their role in plant defense, lignans contribute to abiotic stress tolerance and improve the nutritional quality of plant-based foods, highlighting their importance in both plant physiology and pharmacology [10].
Given the growing global population and the increasing need for crops that are both resilient to environmental stress and capable of improving human health, enhancing lignan production has become a priority [11]. However, extracting lignans can be challenging due to the complexity and variability in the structures and polarities of phenolic compounds. To improve extraction efficiency, methods that increase surface area, such as reducing sample particle size, are often employed. Since lignan concentrations in plants are typically low, which may not meet the demand in pharmacological applications, strategies like biotic and abiotic elicitation, as well as genetic engineering, are being explored to enhance lignan biosynthesis in plants [7,12,13].
This review aims to summarize recent progress in understanding the sources, types, and significance of lignans in crop plants. We will discuss the biological roles of lignans in plant growth under stress and their potential therapeutic applications in human health. Additionally, we will evaluate current biotechnological approaches to increase lignan production and explore future research directions aimed at optimizing lignan content. Ultimately, this review seeks to highlight the potential of enhancing lignan production as a strategy for improving agricultural sustainability, crop resilience, and human nutrition.
2 Structure, Synthesis, Sources, and Type of Lignans
Plant lignans are diphenolic compound groups made up of coniferyl alcohol precursors. Based on the cyclization pattern and oxygen incorporation, lignans are classified into dibenzylbutyrolactone, dibenzocyclooctadiene, dibenzylbutane, dibenzylbutyrolactol, aryltetralin, arylnaphthalene, furofuran, and furan (Fig. 1a–g). Synthesizing these lignans results in several sub-classes, among which artigenin, sesamin, enterolactone, medioresinol, SECO, MAT, LARI, and PINO are major lignans exhibiting various pharmacological properties [14,15]. A complete detail of the synthesis can be found in the review by Dar et al. [16].
Figure 1: Structure of different types of lignans (a) dibenzylbutyrolactone, (b) dibenzocyclooctadiene, (c) dibenzylbutane, (d) aryltetralin, (e) arylnaphthalene, (f) furofuran, (g) furan
Being a natural product, lignans are widely available in several species of plants. Motyka et al. [17] studied the podophyllotoxin (PTOX) belonging to the aryltetralin lactone lignan. Other lignans exhibiting similarity in structure with human estrogens were used as the cancer-preventing substance [18]. Several food sources of lignan are oilseeds and nuts, fruits, non-alcoholic beverages, alcoholic beverages, vegetables, and cereals, which are shown in Fig. 2. Gupta et al. [19], studied the presence of PTOX in the Podophyllum hexandrum Royle, which is a Himalayan native plant. They found a rich PTOX content in their rhizomes and roots. But owing to their overuse, they have been declared as endangered species. Various trials have been carried out for a constant supply of high-yielding clones of PTOX.
Figure 2: Schematic representation of various sources of lignan. The major sources of lignan are fruits, cereals, vegetables, oilseeds, nuts, and alcoholic, and non-alcoholic beverages
PTOX accumulates in various plant species belonging to Linaceae, Cupressaceae, Lamiaceae, and Podophyllaceae [20].
Anjum et al. [9] studied the lignan accumulation in Linum species and found that the commercial Linum usitatissimum, a fiber-rich species, accumulated the PTOX. But they are in small quantities and not suitable for production on a large scale. Several other studies on the plant culture were carried out with Linum nodiflorum, Linum flavum, and Linum album for lignan accumulation. PTOX accumulation was observed mainly in L. album, and the accumulation of 6-methoxypodophyllotoxin (6MPTOX) was observed in L. nodiflorum and L. flavum species. To optimize the cell culture further, the addition of phytohormones was carried out in the medium of growth. Experiments revealed that the Murashige & Skoog medium, when supplemented with a calculated amount of naphthaleneacetic acid and sucrose, was an optimal growth medium for Linum suspensions. The accumulation rate of PTOX in these media is found to be lesser than that of P. hexandrum root or rhizomes. However, the biomass generation of Linum is comparatively higher than the Podophyllum species. The cell culture of L. nodiflorum resulted in a higher accumulation of 6MPTOX than that of the P. peltatum roots and rhizomes. Various studies were carried out using various elicitors for inducing a defense reaction that increases the lignan accumulation [21].
Doussot et al. [22] investigated lignans such as deoxypodophyllotoxin (DOP) and 6MPTOX contents in plant species L. flavum, Juniperus, and Callitris. In addition to these lignans, new types of lignans such as MAT, PTOX, PTOX glucoside, SECO glucoside, and yatein were also found to be present in Callitris and Junipers. Koulman et al. [23] proposed a fast procedure for the extraction of lignans present in Anthriscus sylvestris. In their method, they separated lignans such as DOP, anhydropodorhizol (AHP), and yatein in large concentration variations from the species Anthriscus sylvestris growing at several geographical locations.
Podophyllum peltatum L., commonly known as Himalayan Mayapple, is a major source of PTOX. Tashackori et al. [24] reported the presence of PTOX in Podophyllum peltatum L. and enhanced its production using a fungal elicitor. Szopa et al. [25] observed lignan accumulation in Schisandra chinensis through high-performance liquid chromatography (HPLC). They found that Schisandrol A and B were present in different concentrations in the fruits and leaves of Schisandra chinensis. Nikule et al. [26] identified polyphenolic lignans in Phyllanthus tenellus using HPLC analysis. They detected phyllanthin (PH), niranthin (NH), phyltetralin (PT), and hypophyllanthin (HPH) in varying concentrations. The lignan profile of Linum tauricum and the influence of AM bacterial strain were studied by Ionkova et al. [27], who observed the accumulation of 4-demethyl-6-methoxypodophylotoxin (4DMMPTOX) and 6MPTOX through hairy root culture of the species.
Kancharla et al. [28] investigated the lignan content accumulation in Sesamum indicum, where the major lignans were sesamolin and sesamin. Other lignans, such as sesaminol, PINO, sesamol, LARI, MAT, piperitol, episesamin, and samin, were found in lower concentrations with some of them formed by transformation during the processing of oil and seeds. Andargie et al. [29] studied the production of lignans in Daphne odora cell culture and callus. The lignans such as LARI, PINO, SECO, MAT, and wikstromol (WIKS) were produced through cell suspension culture. The different types of lignan are reported in various crops, fruits, nuts, and vegetables [7]: however, the level of lignan ingestion and its bioavailability, depend on the type of diet consumed, which can be variable.
3 Lignan and Human Health (Food and Pharmaceutical Importance)
3.1 Lignan in the Food Industry
The increasing prevalence of diseases linked to unhealthy lifestyles has driven a growing demand for healthy foods and nutritional supplements, raising awareness of the significant benefits of lignans. The global lignan market is projected to surpass $90 million by 2026 [30]. Currently, efforts are underway to determine lignan content based on specific genotypes for biofortification breeding and the development of functional foods [31]. In recent years, new plant varieties have been bred to enhance lignan content.
There is increasing interest in lignan-containing materials within the food industry, with primary sources including oilseeds, cereals, legumes, vegetables, and fruits [7] (Fig. 2). Among oilseeds, sesame seeds (Sesamum indicum L.), which contain sesaminol have the highest lignan content after flaxseed (Linum usitatissimum L.), while cashew nuts (Anacardium occidentale L.) also provide a relatively rich source of lignans. In cereals, lignans are predominantly found in the outer layers of the grain, with significantly lower concentrations in the endosperm. Some of the crops richest in lignans include rye (Secale cereale L.), wheat (Triticum aestivum L.), oats (Avena sativa L.), and triticale (×Triticosecale), while lower concentrations are found in other grains [2]. Lignans can improve the nutritional value of foods such as cereals, breads, and dairy products. Flaxseed, a potent source of lignan, is frequently incorporated into products to promote cardiac health [5]. Lignan bioavailability varies on its method of consumption. A study involving healthy adults who consumed whole, crushed, and ground flaxseed (0.3 g/kg body weight) for 10 days revealed that whole flaxseed significantly increased the bioavailability of enterolignans by 28% and crushed flaxseed by 43% compared to control [32]. Sprouting flaxseed enhanced both lignan production and mineral content [33]. Lignan mitigates the oxidation of lipids and oils, thereby prolonging the shelf life of items such as sauces, baked goods, and munchies [34]. A significant milestone has been achieved in the development of solin flax (edible oil) through mutagenesis, resulting in a point mutation in the LuFAD3A and LuFAD3B genes, which encode microsomal desaturases. This advancement has broadened the applications of linseed oil for edible purposes [35].
3.2 Lignan and Pharmaceutical Importance
3.2.1 Lignan in the Prevention of Cancer
Lignans offer notable health benefits to humans, particularly in the treatment of chronic diseases such as cancer. Due to their phenolic structure, lignans possess strong antioxidant properties, making them highly effective in combating breast and prostate cancers. Teodor et al. [36] reviewed the biological effects of lignans derived from medicinal plants in humans. They were involved in the prevention of cancer and have antioxidant, anticarcinogenic, antimutagenic, and anti-estrogenic effects.
The role of lignans in human health is mainly in reducing the risk of colon cancer, breast cancer, prostate cancer, endometrial cancer, and cardiovascular diseases. The gut flora present in the human digestive system converts plant lignans into enterolignans, which are proactive against chronic diseases and reduce the risk of tumors [37]. Podophyllotoxin is one of the most notable lignans, with its semi-synthetic derivatives showing significant pharmacological activities, including antineoplastic properties. Derivatives such as etoposide and teniposide are used to treat testicular cancers and acute lymphoblastic leukemia, respectively [38]. Lignans are being investigated for their potential function in the prevention and treatment of hormone-dependent malignancies, with a particular emphasis on breast cancer, because of their estrogen-modulating effects [4]. Lignans, particularly those made from flaxseeds, have demonstrated potential in inhibiting the proliferation of prostate cancer cells by regulating hormone metabolism and generating anti-inflammatory effects [39].
3.2.2 Lignan in Cardiovascular Disease Treatment
Lignans are known to reduce the risk of cardiovascular diseases. Taulabi et al. [40] showed the influence of flaxseed lignan in lowering blood pressure and reducing cardiovascular disease risks. The high content of alpha-linolenic acid and omega-3 fatty acids helps lower total cholesterol levels and supports weight loss. Research suggests that consuming flaxseed powder for 12 weeks can result in reductions in blood pressure, total cholesterol, and body mass index (BMI). Furthermore, higher doses of flaxseed and prolonged consumption may be even more effective in reducing certain cardiovascular risk factors. Nutraceuticals: Lignan-rich supplements are marketed based on their potential to reduce the risk of cardiovascular diseases and hormone-related diseases (e.g., breast cancer and prostate cancer) [41].
Neolignans and lignans can reduce cholesterol levels in plasma, lowering the risk of developing cardiovascular disease [42] and preventing hypertension [43]. A higher long-term intake of lignans has been linked to a notably reduced risk of total coronary heart disease (CHD) in both men and women. There may be synergistic effects between lignan and fiber consumption in reducing CHD risk, potentially by boosting the production of enterolignans [44].
Researchers also identified lignans as antidiabetic compounds, with SDG from flax seeds being the most prominent antidiabetic lignan [45]. Furthermore, studies have shown that regular consumption of products enriched with SDG lowers C-reactive protein levels. Dietary lignan intake, evaluated through a 7-day diet record, was linked to reduced HbA1c levels (with percentage changes ranging from −0.92% to 1.50%), along with decreased C-reactive protein levels and improved lipid profiles. Thus, long-term lignan consumption was associated with a reduced risk of type 2 diabetes, especially in individuals with obesity and premenopausal women [46]. Lignans may exhibit advantageous effects in the management of type 2 diabetes by enhancing insulin sensitivity and reducing blood glucose levels [47].
3.2.4 Other Miscellaneous Roles of Lignan
Lignans are recognized as phytoestrogens due to their structural similarity to steroids, exhibiting both estrogenic and antiestrogenic properties. Research has indicated that these phytoestrogens can alleviate menopausal symptoms, such as osteoporosis, climacteric symptoms, and estrogen-dependent cancers in postmenopausal women [48]. Enterolignans, which are metabolites of lignans, can bind to estrogen receptors alpha and beta, which are present in various tissues. Although lignans have been reported to weakly inhibit aromatase, also known as estrogen synthetases or synthases, which are enzymes crucial for estrogen biosynthesis [49], they may also inhibit 5α-reductase enzymes and stimulate the production of sex hormone-binding globulin [50]. Some lignans, like LARI, MAT, SECO, PINO, and nortrachelogenin, are excellent at fighting free radicals, which makes them useful for both prevention and treatment [51]. Lignans have the potential to promote neuroprotection by crossing the blood-brain barrier and exhibiting antioxidant and anti-inflammatory responses. This enables the creation of lignan-based remedies for neurodegenerative diseases such as Parkinson’s and Alzheimer’s [52,53]. Lignans have demonstrated antiviral properties in numerous studies, which has sparked interest in their potential as a source of infection-fighting compounds [54]. Lignans have the potential to function as potent antioxidants, effectively neutralizing destructive free radicals that can cause cellular damage. This property renders them functional in the development of neuroprotective, anti-inflammatory, and anti-aging medications [4]. The Fig. 3 provides a quick overview of lignan’s role in disease, infection, obesity, and stress.
Figure 3: Lignan and its impact on plants and humans. The above figure shows the deleterious impact of lignan deficiency on human mental and physical health and in plants in the excessive ROS generation and visible stress symptoms
4 Lignan and Abiotic/Biotic Stress Tolerance
Lignans are a potent weapon that plants use to withstand stress [1]. SECO, PINO, MAT, sesamin (Ses), syringaresinol (Syr), and LARI are the seven major types of lignans, which possess antibacterial and antifungal properties [4]. Plants, when confronted with stress, adapt to it by altering the gene expression of proteins that regulate the biosynthesis of metabolites, which are critical for interactions between the plant and its environment. Polyphenols represent a key category of specialized metabolites that are essential for many physiological processes in a plant’s life cycle, particularly in stress responses. Adverse environmental conditions such as drought, extreme temperatures, salinity, heavy metal exposure, and ultraviolet radiation trigger the phenylpropanoid biosynthetic pathway, leading to the accumulation of diverse phenolic compounds [55]. Plants cope with stress either through mechanical or chemical defense. The first line of defense is the mechanical defense, and lignan can contribute to the plant’s defense by being incorporated into cell walls, making them slightly more resistant to degradation and reinforcing the plant’s structural barriers against pathogens and herbivores. The xylem tissue of linseed contains lignan, which makes the stem stiff and strong and lowers the permeability of the cell wall to prevent pathogens from entering [56].
The other mode of defense includes the chemical defense in which secondary metabolites play an essential role. In response to environmental stress signals, plants can resist stress by modulating the accumulation of secondary metabolites [57]. Plant phenolics, or polyphenols, are widely distributed secondary metabolites that are derivatives of the shikimate, phenylpropanoid, and pentose pathways [58]. The precursor of the shikimate pathway, shikimic acid is generated via erythrose 4-phosphate (pentose phosphate pathway) combined with phosphoenol pyruvate (glycolytic pathway). Phenylalanine obtained from the shikimate/phenylpropanoid pathway leads to synthesizing other phenylpropanoid metabolites [59]. Several other metabolites, such as flavonoids, lignans, lignins, tannins, etc., are formed through this pathway [58,60]. Therefore, the phenylpropanoid pathway serves as a boundary of carbon metabolic flux of primary metabolites into secondary metabolites [61].
Lignan synthesized via the phenylpropanoid pathway is structurally analogous to lignin, a molecule that fortifies cell walls [62]. Many lignans are naturally harmful to pathogens. They can damage the membranes of invading microorganisms or interfere with their metabolic processes to provide a direct antimicrobial effect [63]. Some lignans serve as precursors of signaling molecules essential for immunological responses. They can modulate the concentrations of reactive oxygen species and other defense-related hormones, optimizing the immune response to avert excessive damage to plant tissues [64]. Lignans play a crucial role in plant immunity by increasing structural integrity and biochemical resistance [65].
Initially, in vitro studies demonstrated the potential of the lignans Ses, asarin, and PINO to combat insect stress [66]. Researchers found that SDG can raise the levels of catalase (CAT) and superoxide dismutase (SOD), which are involved in free radical scavenging [4]. Lignans are reported to have an important role in helping plants cope with biotic and abiotic stresses during growth and development [67]. The antioxidant, antiviral, antibacterial, and antifungal properties of lignans are responsible for their plant defense mechanisms [68]. Wan et al. [69] revealed that lignan possesses antifungal properties in sesame tissue extracts. Similarly, Li et al. [70] reported the insecticidal properties of furofuran lignans derived from the Himalayan shrub lopseed (Phryma leptostachya L.). Lignans function as antioxidants that can scavenge ROS, which typically accumulate excessively under stressful conditions [21]. Drought stress may alter the concentration of lignans in the primary sesame (Sesamum indicum L.). More drought-tolerant genotypes exhibited elevated levels of sesamin and sesamolin [71]. Phenolic compounds have been shown to play a beneficial role in protecting living organisms from various oxidative stressors. They are crucial for maintaining redox homeostasis and offer potential strategies for improving plant resistance to stress [72]. Lipid peroxidation is a damaging oxidative process that alters membrane structures, thereby affecting their biological functions. Lignans can protect membranes from this damage by scavenging free radicals [73].
It has been shown that increasing LARI biosynthesis in Isatis indigotica, especially in tetraploids compared to diploids, helped roots grow and made the plants better able to handle salt and drought stress. A key enzyme in lignan biosynthesis, 4-coumarate A ligase 3 (Ii4CL3), is activated by the transcription factor IiWRKY34. Drought stress may change the level of lignans in the main sesame, depending on genotypes. More drought-tolerant genotypes exhibited higher levels of sesamin and/or sesamolin [74].
Dirigent (DIR) proteins have been shown to mediate the biosynthesis of lignans and lignin in plants under stress, indicating their role in adaptive plant responses [75]. The expression of IiWRKY34 is positively associated with LARI accumulation and the enhanced stress resistance observed in these plants [76]. Analysis of the expression patterns of selected DIR genes in flax revealed the involvement of specific DIRs in the formation of (−)-PINO in seed coats and (+)-PINO in vegetative tissues, as well as in response to hormonal or fungal elicitor stress [76]. Lignan biosynthesis is also stimulated by stress hormones, such as jasmonic acid (JA), salicylic acid (SA), and abscisic acid (ABA) [21].
The expression of genes involved in the phenylpropanoid pathway, which is essential for the synthesis of lignans, is frequently altered by abiotic stress [77]. Phenylalanine ammonia-lyase (PAL), cinnamate-4-hydroxylase (C4H), and 4-coumarate-CoA ligase (4CL) are among the critical enzymes in this pathway that are upregulated in response to stress [78]. This ultimately results in a higher production of lignan precursors, such as coniferyl alcohol, which is subsequently converted into lignans through the phenylpropanoid pathway [79]. Lignans play protective roles in plants, particularly during abiotic stress. Two flax varieties, Flanders and Astella, were compared for their salt tolerance ability based on lignan accumulation by Debnath et al. [80]. It was observed that Flander exhibited a greater up-regulation of antioxidants and osmoprotective mechanisms, indicating its superior ability to scavenge ROS and protect cells compared to Astella. Additionally, the two-tailed qPCR analysis revealed a more pronounced upregulation of three miRNAs associated with lignan biosynthesis: miR168a, miR399g, and miR828a. Phenylcoumaran benzylic ether reductase (PtPCBER) is a member of the PINO-LARI, isoflavone, phenylcoumaran benzylic ether reductase (PIP) family, which plays a role in the lignan biosynthetic pathway. Two soluble enzymes, pinoresinol-lariciresinol reductase (PLR) and PCBER, catalyze the reduction of benzylic ether in the 8–8′ and 8–5′ linked lignans. PCBER facilitates the formation of various lignans through NAD(P)H-dependent 7-O-4 reduction of precursor compounds [79,81]. Finally, lignans undergo glycosylation modification and are stored as lignan glycosides in plant tissues [82,83]. Overexpression of PtPCBER enhanced lignan production, which in turn scavenged ROS generated by salt stress in poplar. This reduction in ROS alleviated oxidative damage due to high salt stress, resulting in increased salt tolerance in transgenic poplar plants [84].
Lignan structure makes them effective antioxidants, capable of scavenging harmful ROS that typically accumulate under stress conditions. For instance, the flaxseed lignan SDG and its mammalian metabolites, ED and EL, exhibit antioxidant activity without prooxidant effects by reducing lipid peroxidation and decreasing deoxyribose oxidation and DNA strand breakage [85,86]. Suja et al. [87] screened the antioxidant activities of isolated and purified compounds from sesame cake, including sesamol, sesamin, sesamolin, sesaminol diglucoside, and sesaminol triglucoside. Using the β-carotene-bleaching assay and the inhibition of linoleic acid peroxidation by the thiocyanate method, they found varying degrees of antioxidative activity among the lignans, with free-form lignans generally showing greater antioxidant power than glucosides. Lignans from the root bark of fringe tree (Chionanthus virginicus L.), such as phillyrin, pinoresinol-β-d-glucoside (PDG), and pinoresinol di-β-d-glucoside (PDDG), demonstrated significant antioxidant activity in comparison to standard antioxidants like butylated hydroxyanisole (BHA), butylated hydroxytoluene (BHT), and α-tocopherol and its water-soluble analog trolox in a series of in vitro tests [88]. In these assays, it was observed that lignans with an oxygen-free benzylic position exhibited higher radical-scavenging activity [89]. The antioxidant activity of lignans is a fundamental aspect of their positive role in plant responses and tolerance to abiotic stress. Additionally, their participation in lignification and cell-wall synthesis contributes to stress responses. For example, drought stress can alter lignan levels in sesame (Sesamum indicum L.), with more drought-tolerant genotypes showing higher levels of sesamin and/or sesamolin [71].
Experiments on metal stress have shown that the Cd-tolerant CB671 genotype of oilseed rape (Brassica napus) accumulates lignans and cell-wall saccharides. This suggests that cell-wall priming may play a role in its tolerance. In contrast, the Cd-sensitive genotype (ZD622) accumulates phenolics, particularly from the upstream subclasses of flavonoids. The observed accumulation of lignans and cell-wall saccharides in the Cd-tolerant CB671 genotype may indicate an enhanced antioxidant capacity within the cell wall, which likely helps mitigate the stress caused by elevated cadmium (Cd) levels [90].
Beyond their role in responding to abiotic stress, lignans are crucial for plant defense against pathogens. They achieve this by inhibiting microbe-derived degradative enzymes like cellulases, polygalacturonases, glucosidases, and laccases. Additionally, lignans can act as insecticides by disrupting the endocrine systems of insects [77].
Biotic and abiotic stress conditions are often associated with elevated levels of stress hormones such as SA. Research has shown that applying these stress hormones externally can boost lignan biosynthesis. For instance, adding 50 μM sSA to flax (Linum usitatissimum L.) cell cultures resulted in a two-to four-fold increase in the production of lignans like SDG and lariciresinol diglucoside (LDG), as well as neolignans such as dehydrodiconiferyl alcohol glucoside (DCG) and diacylglycerol-β-coniferyl alcohol ether glucoside (GGCG) compared to controls [91]. Additionally, methyl jasmonate was found to stimulate lignan biosynthesis in Isatis indigotica hairy root cultures, enhancing both gene expression and metabolite accumulation of coniferin, LARI, SECO, and PINO [92]. Utilizing stress hormones to elicit phenylpropanoid biosynthesis is increasingly becoming a favored biotechnological approach for producing pharmacologically valuable polyphenols, including biologically active lignans [21].
Hamade et al. [93], through NMR and LC-MS-based metabolomics studies, observed the role of lignan in flax osmotic stress tolerance. Their observations suggested that the mechanism of osmotic stress response of the transgenic line (deficient in lignan synthesis) is somehow different from that of the wildtype, leading to the hypothesis that balanced lignan content may be important for proper stress response.
5 Lignan Content Determination
The demand for lignan sources, as well as methods for their identification, extraction, purification, and analysis, has been steadily increasing in recent years. Among the various extraction and identification methods, mass spectrometry and liquid and gas chromatography were the most common. Charlet et al. [94] presented an HPLC-based quantification procedure for SECO in flaxseed. They used the procedure for the purification of anhydrosecoisolariciresinol (ANHSEC) from the hydrolyzed extract of flaxseed. They carried out a time study for the optimum acid hydrolysis condition evaluation. This method was used in the extract of various organs of plants from roots to fruits for the distribution study of lignans.
The SECO lignan contents in flaxseed were measured through gas chromatography. Before performing hydrolysis, they performed ethanol lignan extraction from both trimmed and untrimmed flaxseeds and observed a lower lignan concentration yield than that of the direct hydrolysis method. Also, through analysis, they observed similar lignan content both in trimmed and untrimmed flaxseeds. They achieved an efficient recovery by reducing the steps of preparation and resources [95]. Bhatnagar et al. [96] presented a fast lignan evaluation method using the ultraviolet absorption-based spectrophotometric procedure for the lignan content determination in sesame oil. Since the extraction of sesamol and sesamin using HPLC needs solvents and columns, they claimed that the developed method is fast and less expensive in sesame oil lignan determination. The experiment results showed that the UV method has the same correlation coefficient in lignan determination and a slightly higher value of lignan contents compared to that of the HPLC method.
A new approach based on Heteronuclear Single Quantum Coherence (HSQC) NMR spectrometry was adopted by Xiao et al. [97] to determine the lignan contents of Sambucus williamsii. Those lignans were bioactive containing carbon signals from oxybenzyl. They compared the resonance signal peak of oxybenzyl carbon with PINO. The experimental results showed that the NMR spectrometry method was reliable in the quantization of complex botanical mixtures with better accuracy. They also calculated the amount of lignan to be approximately 10%–15% of the total extract. Various experimentation methods for the quantitative analysis of lignan content have been researched till now using different species rich in lignan content.
HPLC is frequently used to quantify and separate lignans. HPLC can detect the interaction between the stationary phase of the chromatograph and specific lignan compounds [98]. Liquid chromatography-mass spectrometry (LC-MS) is employed to quantify and identify lignan metabolites [99]. Nuclear Magnetic Resonance (NMR) Spectroscopy provides precise structural information on lignan molecules [93]. Fourier-transform infrared (FTIR) spectroscopy helps in identifying the functional groups present in lignans [100]. Solvent extraction is a prevalent technique that uses solvents such as methanol or ethanol to isolate lignans from plant sources. Supercritical Fluid Extraction (SFE) is a more sophisticated method used for the extraction of lignans [98]. Fig. 4 provides a view of the various methods used for lignan content extraction.
Figure 4: Major lignan type with their extraction, purification and determination method
6.1 Lignan Production Enhancement through Biotechnological Methods
Lignan production from plants is declining due to factors such as suboptimal cultivation practices, high extraction costs, low lignan content, and long generation cycles. Additionally, factors like species type, plant maturity, age, genetic composition, environmental conditions, and physiological factors all limit the lignan content. To address this, lignan production needs to be enhanced through efficient and sustainable methods. In this regard, various studies have focused on biosynthetic approaches and metabolic strategies to boost lignan yields. Therefore, biotechnological methods for producing lignan have garnered significant attention in recent years. Among several methods available for lignan enhancement, the most efficient, reliable, and commonly used include in vitro suspension cultures, using microbial elicitors, and genetic engineering methods [7,9,101]. In this review, the impact of these three methods on lignan accumulation in various plant species is presented.
6.1.1 In Vitro Suspension Culture Method for Lignan Enhancement
In vitro, or micropropagation, is a method of plant multiplication asexually in an aseptic condition to enhance the lignan contents in the plant species that are vital commercially. Initially, the tissue culture method of Linum species was introduced, and since then various species were involved in this process. Yildiz et al. [102] studied the explant’s source effects on greenhouse and in vitro-influenced Linum usitatissimum. Through experiments, they analyzed the explants producing the shoot, the shoot number, and the overall number of shoots for each sample. They observed better regeneration of shoots by hypocotyl explants, and the seedlings grown in vitro were more suitable than the ones that were grown through the greenhouse method.
Samadi et al. [103] presented the hairy root culture method in Linum mucronatum species using Agrobacterium rhizogenes stains for lignan production. They also used liquid culture media based on Murashige-Skoog for the hairy root establishment. They observed the successful hairy root production of transgenic nature. Highly transforming hypocotyl segment-type explants were the reason for the production of hairy roots, and these roots provided a suitable platform for the optimization and production of aryltetralin lignans like PTOX and its derivatives. Similarly, in vitro solid cultures (microshoots and callus), cultivation periods (10, 20, and 30 days), and different concentrations of plant growth regulators-BA, IBA, and GA3 (ranging from 0 to 3 mg/L) in Murashige and Skoog medium were tested for the presence of several lignans, including dibenzocyclooctadiene lignans (schisandrin, gomisin G, schisantherin A and B, deoxyschisandrin, and schisandrin C), dibenzylbutane lignans (hernicine B), aryltetralin lignans (wulignan A1 and A2, epiwulignan A1, enshicine, epienshicine, and dimethylwulignan A1), as well as triterpenoids (kadsuric acid and schisanhenric acid). Their presence was confirmed using UHPLC–MS/MS analysis. The total amounts of secondary metabolites in the extracts from in vitro cultures were found to be 13.0, 7.0, and 1.4 times higher than those in leaf extracts, which were analyzed for comparison. This is the first report on the biosynthetic potential of cells from Schisandra henryi in vitro cultures [104]. In Linum usitatissimum, a high level of lignan accumulation was recorded through root-derived calli. Lignans such as SDG, LARI diglucoside (LDG), dehydrodiconiferyl alcohol glucoside (DCG), and guaiacylglycerol-β-coniferyl alcohol ether glucoside (GGCG) were observed in the culture (100). Both in vitro cultures of flax (both callus and adventitious root) were studied. However, it was the adventitious root culture that efficiently produced a higher amount of lignans (at day 40) and neolignans (at day 30) than the callus culture of flax [9].
Cell culture of L. persicum was done to study lignan production and evaluation for anti-carcinogenic activity on the MCF7 cell line. Lignan contents were studied in cells, callus, plantlets, capsules, leaves, stems, and roots using the HPLC technique. It was observed that the lignan PTOX was highest in capsules and pinoresinol in leaf and root organs. The shoot extract of the in-vitro plantlets has greater cytotoxic effect on breast cancer cells (IC50 = 5 μg/mL) [105]. Hepatoprotective and anticancer polyphenolic lignans, phyllanthin, hypophyllanthin, niranthin, and phyltetralin were observed both in natural plants and in vitro cultures of Phyllanthus tenellus Roxb. In a culture medium incorporated with 1.0 mg/L of 6-benzylaminopurine (BAP), there was maximum shoot regeneration, while indole-3-acetic acid (IAA) supplementation at 2 mg/L was superior for induction of rooting in in-vitro raised shoots. Such plantlets showed 100% survival under field conditions. The content of lignan was higher in callus grown on Murashige and Skoog (MS) medium supplemented with 2.0 mg/L Naphthaleneacetic acid (NAA). These concentrations of nutrients and variations in hormones (auxin and cytokinin) can be used to develop protocols for mass propagation of lignan and biotechnological approaches for the improvement of P. tenellus [26].
Khan et al. [56] produced potent lignans and antioxidant secondary metabolites in linseed through in vitro micropropagation. They used hypocotyl explants, supplemented them with thidiazuron at 0.5 mg/L concentration with kinetin at 0.5 mg/L in the basal growth media, and observed 86.87% shoot induction frequency and higher shoot organogenesis in 4 weeks. Thidiazuron, with kinetin, activated the antioxidant system (total phenol, flavonoid, DPPH free radical scavenging, phenylalanine ammonia-lyase activity, SOD expression). It also enhanced the pharmacologically active lignans such as SECO, SDG, and ANHSEC diglucoside in the regenerated shoots. This method can be used by scaling it up for commercial lignan production to meet the requirement.
Scarlet flax (Linum grandiflorum L.) in vitro culture was done to obtain lignans and neolignans for antioxidant and anti-inflammatory applications. The hypocotyl and cotyledon explants were supplemented with different concentrations of α-naphthalene acetic acid (NAA) and thidiazuron either alone or in combinations. NAA (1.0 mg/l) was better in inducing callus formation in hypocotyl explants than thidiazuron alone. The impact of NAA was higher compared to both the NAA and thidiazuron combination, although lesser than thidiazuron alone. This shows that there was no synergy between NAA and thidiazuron. The biomass of NAA-treated explants was higher, but in combination with thidiazuron, it further increased, showing a synergy between the two PGRs, particularly for cotyledon-derived explants. Lignans like SECO, LARI, and neolignan (dehydrodiconiferyl alcohol (DCA)) naturally accumulate in their glycoside forms. The study focuses on enhanced biomass accumulation and efficient production of (neo) lignans in L. grandiflorum callus cultures [106].
Lignan-like phyllanthin and hypophyllanthin were observed in the shoot culture of P. amarus Schum. & Thonn. by direct organogenesis. Various plant growth regulators (PGRs) were used in different combinations and their impact on lignan was observed (PGRs: kinetin(Kin), 6-benzylaminopurine (BAP), 2-isopentenyladenine (2iP), 1-phenyl-3-(1,2,3-thiadiazol-5-yl)urea, thidiazuron, auxin, andindole-3-butyric acid (IBA)). Lignan production was maximum in the shoot culture grown on Murashige and Skoog’s (MS) medium supplemented with Kin 0.25 mg/L. Both phyllanthin and hypophyllanthin increased, showing the potential for large-scale cultivation of P. amarus shoots for medicinally important lignan production [107].
In 2024, Silva et al. [108] observed that biomass production and PTOX content were measured in the root cultures of Hyptis suaveolens (L.) Poit grown in liquid medium supplemented with different concentrations of indole-3-butyric acid (IBA), 1-naphthaleneacetic acid (NAA), vitamins, and myo-inositol. High-performance liquid chromatography with diode-array detection (HPLC-DAD) was used for PTOX quantitation. MS medium supplemented with 1 mg/L IBA and 0.5 mg/L NAA was maximally effective in enhancing PTOX concentration in the roots and also root dry weight. Vitamin and myo-inositol supplementation also enhanced PTOX content.
In another study for enhancing lignan production, Dougué Kentsop et al. [101] observed adventitious (ARL) and hairy roots (HRL) grown under in vitro conditions in Linum lewisi. L lewis synthesizes arylnaphthalene lignans such as justicidin B and also has phenol content and antioxidant activity. Four other lignans were also observed through NMR spectroscopy in the root extracts. The ARL and HRL cultures showed a higher justicidin B production compared to other Linum species cultures, and the production was further enhanced by elicitation with MeJa. Overall, the growth performance of HRL lines was better than ARL lines, and HRL6 showed higher justicidin B content than ARL1 at 3, 4, and 5 weeks (p ≤ 0.05). HPLC analysis on methanolic extracts of HRL6 and ARL1 revealed the presence of other molecules besides justicidin B. It is worth noting that in-vitro culture enhances lignan production to a much higher extent than plants growing under natural conditions and in this regard, various PGRs and other chemicals play an essential role. Accumulation of lignan was observed using suspension culture of seedlings of Linum album after the addition of DOP. DOP was found to induce the production of PTOX and 6MPTOX lignans [109]. A similar experiment was done on Linum album through the accumulation of feeding experiments with hairy root culture after the addition of coniferaldehyde. Lignans LARI, PINO, and PTOX accumulation were enhanced by 14.8, 8.7, and 1.5 times, respectively, with coniferaldehyde [110]. In Linum flavum, lignan (5MPTOX) production in hairy root culture was improved by 1.5% to 3.5% on dry weight compared to untransformed tissue [111]. In Phyllanthus amarus shoot culture, the application of PGRs affected shoot development and the accumulation of biologically active lignans through direct organogenesis. The total lignan content, comprising phyllanthin and hypophyllanthin, was approximately 8–10 mg/g DW, comparable to or exceeding the levels found in plant material harvested from natural environments [107]. The in vitro method is further used for lignan enhancement by the elicitation method, which is discussed in the next section.
6.1.2 Elicitation Methods for Lignan Enhancement Using In Vitro Culture Methods
Elicitors are foreign molecules that stimulate the production of secondary metabolites by triggering stress responses or defense mechanisms in living cells. Depending on their nature, elicitors are classified into biotic and abiotic categories. Biotic elicitors include substances like yeast or fungal extracts, chitosan, cellulase, and chitin, while abiotic elicitors are physical or chemical stressors that induce similar responses. (Fig. 5). The physical stressors include UV light, temperature, pH, etc., and the chemical stressors include heavy metal ions like silver (Ag), copper (Cu), cadmium (Cd), Nickel (Ni), etc. In addition, the third component of abiotic elicitors includes signaling compounds such as methyl jasmonate (MeJa) and SA. It has been evident from various research that the use of elicitors improves lignan production.
Figure 5: Schematic representation of different types of elicitors. The major classes of elicitors are abiotic elicitors (physical, chemical, and intracellular) and biotic elicitors
Biotic Elicitation
Li et al. [112] studied the effect of yeast extract as a biotic elicitor on lignan production in Salvia castaneta. They recorded the accumulation of tanshinone and plant growth in root cultures. Experiment results showed a 1.8–1.99 times increase in tanshinone contents by using yeast extract in comparison with silver ion and MeJa, respectively. Yeast extracts also enhanced the dry mass and cryptotanshinone content. Through gene expression study it was evident that the yeast extracts heightened the expression levels of isopentenyl isomerase. Zhao et al. [113] presented a study using yeast polysaccharides as an elicitor for the stimulation of secondary metabolites in the root culture of Fagopyrum tataricum. They recorded a 3.2-times enhancement in flavonoid yield compared to the control culture and enhanced the flavonoid accumulation. Ming et al. [114] reported the influence of mycelium extract and the fraction of polysaccharide produced from Trichoderma atroviride on the secondary metabolites of the root culture of Salvia miltiorrhiza. They performed an HPLC analysis procedure and observed that steady growth in hairy roots was recorded in the growth period, and it was rapid in treated groups. Also, the enhancement effect of both mycelium and polysaccharides on the production of tanshinones was similar, and a slight increase was recorded when treated with polysaccharides for an exposure period greater than 18 days. Szopa et al. [12], through their study, reported that elicitors like chitosan (200 mg/L), yeast extract (3000 mg/L), ethephon (25 µM/L), and methyl jasmonate (50 µM/L) affect lignan production in microshoot cultures of both female (F) and male (M) Schisandra rubriflora Rehd. et Wils. Elicitors were given at 10 days of culture, and samples were collected after 1, 2, 4, 6, and 8 days post-elicitation. UHPLC–MS/MS analysis revealed the presence of dibenzocyclooctadiene, aryltetralin, dibenzylbutane, and tetrahydrofuran lignans and neolignans in the biomass extracts. All elicitors enhanced lignan production. The male cultures in bioreactors exhibited the highest total lignan content in their biomass extract, with MeJa yielding 153.20 mg/100 g DW. Among the bioreactors and agitated culture, it was the agitated culture that showed 3.29 times and bioreactors 1.13 times higher lignan than in non-elicited cultures. Tashackori et al. [24] found that the elicitation by the root endophytic fungus Piriformospora indica produced H2O2 molecules in the hairy roots of Linum album, resulting in alterations in physiological, biochemical, and molecular responses. These changes included adjustments in the antioxidant mechanisms and the synthesis of lignans. Analyzing the metabolic responses to this elicitation reveals that both enzymatic and non-enzymatic defense mechanisms play crucial roles in the adaptation of L. album hairy roots to the influence of P. indica, besides enhancing lignan production.
Fungal elicitor Fusarium graminearum extract was used to study lignan PTOX and MPTOX enhancement in Linum album. Its extract resulted in the highest increases of PTOX, reaching 190 μg g−1 DW, and LARI, at 260 μg g−1 DW, which were two-fold and three-fold higher than the untreated control, respectively. In contrast, Trichoderma viride extract boosted the accumulation of MPTOX, rather than PTOX, up to 160 µg g−1 DW, representing a 2.4-fold increase compared to the control. The enhancement of lignan production by these fungal elicitors was associated with increased expression of several key genes involved in their biosynthesis, including phenylalanine ammonia-lyase, cinnamoyl-CoA reductase, cinnamyl-alcohol dehydrogenase, and PINO-LARI reductase [115].
Gononcharuk et al. [116] studied the effect of yeast extract as an elicitor at various concentrations (200–1000 mg/L) on the accumulation of phenolic compounds in Linum grandiflorum Desf. cells cultured in vitro. It was observed that both the total phenol content and the levels of phenylpropanoids increased significantly in the cell culture, particularly at higher YE concentrations (500 and 1000 mg/L). Such an increase in the content of phenylpropanoids and the expression of genes involved in the phenylpropanoid pathway following exogenous treatment of in vitro plant cultures with biotic elicitors, such as polysaccharides, suggest, a regulatory role for phenylpropanoids, which are characterized by their strong radical-scavenging ability, in maintaining redox homeostasis in plant cells. The finding of elicitation by biotic elicitors is summarized in Table 1.
Abiotic Elicitation
Gai et al. [122] studied the use of ultraviolet (UV) radiation as an elicitor in Astragalus membranaceu (AM) HRC for the enhancement of Astragalosides (AG) production. They observed that maximum production of AG was recorded in the AM of 32-day-old exposed to UV radiation. This production was 1.32 times higher than that of the non-treated control production. Zhang et al. [123] evaluated the impact of silver nanoparticles as an elicitor in Artemisia annua for the enhancement of artemisinin production. They recorded a 3.9 times increase in the production of artemisinin in the 20-day root culture of Artemisia annua. An investigation on the impact of KCl and CaCl2 with applied salt stress as an elicitation method on the hyoscyamine content of various Datura species was performed by Harfi et al. [124]. KCl with a concentration of 2 g/L was applied to Datura tatula for 10 h contact time and on Datura stramonium, innoxia for a 24 h contact time. The results were compared with the application of CaCl2 on both the species for 10 and 24 h elicitation time. They observed a maximum hyoscyamine content production in D. tatula by using CaCl2 over an elicitation period of 24 h.
The use of signal molecules such as MeJa and SA on the secondary metabolite accumulation in Rhinacanthus nasutus was investigated by Cheruvathur et al. [125]. They carried out a time study on the production of rhinacanthin without elicitor for 4 weeks and with the addition of MeJa and SA for a culture period of 6 weeks in MS medium. They observed the highest accumulation of RC content with the addition of MeJa.
Similar enhanced lignan production was observed by Sykłowska-Baranek et al. [126], who studied the potential for accumulating six lignans in two lines of Taxus x media hairy roots. Hairy root cultures from the KT and ATMA lines were treated with various precursors, including coniferyl alcohol (CA at 1, 10, or 100 µM), l-phenylalanine (100 µM), and methyl jasmonate (100 µM). Additionally, two-phase in vitro cultures utilizing perfluorodecalin (PFD) as a gas carrier and in situ extractant were employed. It was noted that the two hairy root lines exhibited different profiles in lignan production. While in the untreated controls, KT roots did not produce SECO or LARI lignan, ATMA roots lacked MAT. Treatment with CA at 1 or 10 µM in ATMA roots led to the production of both LARI and SECO, whereas only LARI was detected at 100 µM CA. Elicitation with 1 µM CA and MeJa together resulted in the highest yields of hydroxymatairesinol aglyca and lariciresinol glucosides, measuring 37.88 and 3.19 µg/g DW, respectively. It was the combined treatment of 1 µM CA, PHEN, and MeJa that significantly stimulated lignan production, particularly in cultures supplemented with PFD-aerated or degassed conditions. In ATMA roots, these conditions were optimal for MAT reaching levels of 199.86 and 160.25 µg/g DW in PFD-aerated and PFD-degassed cultures, respectively. For KT roots, only hydroxymatairesinol and coniferin/CA levels increased, with maximum yields of 59.29 and 134.60 µg/g DW in PFD-aerated and PFD-degassed conditions, respectively.
To make lignan extraction more cost-effective, chitosan can be used, which is a low-cost, toxic non-toxic effective elicitor for lignan production. Jiao et al. [127] observed that chitosan stimulated the production of lignan 6MPTOX in the Linum album. This production was mediated by the signaling of second messengers, including cytosolic free Ca2+, hydrogen peroxide (H2O2), and nitric oxide (NO), by activating plasma-membrane Ca2+ channels and NADPH oxidase, as well as nitrate reductase/nitric oxide synthase-like enzymes. Inhibitors like EGTA and W-7 reduced the induction of key enzymes involved in lignan biosynthesis, indicating the role of secondary messengers in lignan synthesis. Additionally, chitosan increased SA levels, which in turn stimulated 6MPTOX production by affecting phenylalanine ammonia-lyase (PAL) and pinoresinol-lariciresinol reductase (PLR) activity. Real-time PCR results suggested that mitogen-activated protein kinase 6 (MAPK6) function downstream of these second messengers and SA signaling pathways, potentially integrating signals that modulate lignan biosynthesis. Overall, the findings highlight the regulatory role of Ca2+/CaM, H2O2, and NO in lignan biosynthesis, with SA and MAPK6 serving as key mediators in this process [128].
Sagharyan et al. [129] reported that MeJa-induced lignan productions except for LARI and the highest amounts of PTOX and 6MPTOX, approximately 4 and 5 times higher, were analyzed at 50 μM MeJa concentration, which was 4 and 5 times that of the control, respectively. MeJa was found to induce oxidative changes by redirecting free sugars and amino acids toward the production of lignans in L. album cells.
Andi et al. [130] performed their experiment on V. vinifera, which is known for its rich content of stilbene compounds, particularly resveratrol, which are thought to offer dietary protection against cardiovascular diseases and certain cancers. Two light conditions (135.1 μmol s−1m−2 radiation vs. dark) and varying concentrations of phenylalanine (Phe; 0, 0.1, 0.5, and 1 mM) and methyl jasmonate (MeJa; 0 and 25 μM) were tested, both individually and in combination. It was reported that dark-grown cultures accumulated significantly higher levels of total stilbenes (resveratrol + piceid) compared to those exposed to high light. Notably, the combinations of dark with 1 mM Phe and dark with 25 μM MeJa resulted in increased levels of total phenolics, flavonoids, and stilbenes.
Compounds like SA and MeJa were found to enhance the production of secondary metabolites. Benavide et al. [131] cultivated the hairy roots of Phyllanthus acuminatus in 250 mL flasks under a photoperiod of 16 h of light and 8 h of darkness for four weeks, using diffused light. Elicitors were tested at concentrations of 50 and 200 μM. Non-targeted analysis of the different treatments was performed using high-resolution mass spectrometry (HR-MS). The identified metabolites were categorized into phenylpropanoids, phenols, and mucic acids, and a statistical analysis of their relative concentrations was conducted. Compound intensities were assessed using heat maps to visualize the over-expression of metabolites for each elicitation. Dendrograms were used to group compounds exhibiting similar responses across the various treatments. SA at 50 μM concentration shows higher production of secondary metabolites. Karimzadeh et al. [132] found that using nanoparticles could act as another method of elicitation for lignan enhancement. They found that the highest activity of CAD (cinnamyl alcohol dehydrogenase) occurred at a concentration of 60 mg/L of nano-ZnO at various time points. Additionally, a concentration of 150 mg/L of nano-TiO2 also enhanced CAD activity. The maximum level of total phenols was found to be 150 mg/L of nano-TiO2. Various concentrations of nano-TiO2 consistently increased total lignan levels at all intervals. The greatest amounts of total phenols and lignans were recorded at 30 and 60 mg/L of ZnO. This study highlighted the differing effects of nanoparticles on enzyme activity and secondary metabolite production in flax plant cell suspension cultures, influenced by both the concentration and type of nanoparticles used.
Anjum et al. [9] reported that the adventitious root culture in flax caused more efficient production of lignans (at day 40) and neolignans (at day 30) compared to callus culture. HPLC analysis showed that the accumulations of SDG, 5.5 mg g−1 DW, and dehydrodiconiferyl alcohol glucoside (DCG, 21.6 mg/g DW) were twice as high, while diacylglycerol-β-coniferyl alcohol ether glucoside (GGCG, 4.9 mg/g DW) and lariciresinol glucoside (LDG, 11.9 mg/g DW) were 1.5 times higher in adventitious root culture than in callus culture. Additionally, the highest total phenolic production (119.01 mg/L) was recorded in adventitious root culture at day 40, showing an antioxidant-free radical scavenging activity of 91.01%. Thus, it could be suggested that adventitious root culture is a promising candidate for scaling up industrial production of these pharmacologically and nutritionally valuable metabolites. Various other studies are also reported in Table 2.
6.1.3 Genetic Engineering Methods for Lignan Enhancement
Genetic engineering is identified as a promising factor for the evolution of genetic pathways during regulatory functional limitations. In the metabolic pathway elucidation and enzyme identification, this approach of capturing and analyzing the DNA, proteins, metabolites, and mRNA of the targeted cell constitutes a potential tool. Several kinds of research have been triggered after the increased accumulation of cinamoil derivatives reported by Howles et al. [148] in the plant species of transgenic tobacco. Various strategies in genetic engineering have been evolved to enhance lignan production and plant growth. Elevated lignan levels in a transgenic wheat line have been successfully attained by genetically engineering the overexpression of the PLR gene [149].
Chang et al. [150] investigated the phenylalanine ammonia-lyase and hydroxycinnamoyl transferase overexpression impact on the chlorogenic acid accumulation in transgenic tobacco. The impact on the phenolic acid and rutin generation was also carried out. They observed a 3-fold increased accumulation of chlorogenic acid due to overexpression and a 12-fold enhancement in rutin level in the plant species. Kim et al. [151] presented a genetic engineering method for the biosynthesis of lignan in the suspension cell culture of Forsythia koreana. The transgenic overexpression of RNA interference was generated by them, and they observed the impact on the production of PINO. They observed a MAT loss and enhanced PINO accumulation compared with that of the non-transformant. Further, they reported the production of sesamin through the transgenic co-expression of CYP81Q1 enzymes in sesame seeds.
In a study by Zhang et al. [152], lignan biosynthesis was studied in the hairy roots of Isatis indigotica by using MeJa as an elicitor. They investigated the metabolic and transcriptional changes, identifying potential key catalytic steps and regulatory genes involved in lignan biosynthesis. The process was categorized into three phases: signal response, transcriptional activation of metabolic pathways, and metabolite accumulation. A total of 17 hub genes were identified through co-expression network analysis, with 4CL3 selected for validation. RNA interference of 4CL3 led to a significant decrease in lignan production, indicating its crucial role in the biosynthesis pathway, specifically through the esterification of caffeic acid. Overall, the study offers new insights into lignan biosynthesis and highlights targets for metabolic engineering in I. indigotica.
Amani et al. [153] showed that elicitation on Ficus carica hairy roots, using MeJa and cell extract from the fungus Piriformospora indica enhanced the total phenolic and flavonoid content, antioxidant activity (assessed using DPPH and FRAP assays), gene expression related to phenolic and flavonoid biosynthesis, and HPLC analysis of key phenolic compounds was evaluated. MeJa treatment induced high expression levels of chalcone synthase (CHS), flavonoid 3′-hydroxylase (F3′H), and transcription factors MYB3 and bHLH. In contrast, P. indica elicitation resulted in the highest expression levels of phenylalanine ammonia-lyase (PAL) and UDP-glucose flavonoid 3-O-glucosyltransferase (UFGT). Furthermore, P. indica treatment led to significant increases in the content of several phenolic compounds, including gallic acid, caffeic acid, rutin, quercetin, and apigenin. The enhancement in gene expression paves the way for further enhancement of different secondary metabolites through the use of elicitors.
To ensure a reliable supply of natural products, new production strategies have been developed, including gene transfer into heterologous organisms and metabolic engineering [154]. Perrin et al. [155] used the cofactor boosting approach to enhance the precursor of lignan PTOX in yeast. The biosynthesis of PTOX occurs through a three-step process: first, tyrosine and phenylalanine are deaminated to produce p-coumaric acid (pCA), which is then hydroxylated and O-methylated to yield caffeic acid (CaA) and ferulic acid (FA), respectively. Each of these enzymatic steps, catalyzed by heterologous enzymes, requires cofactors such as NADPH, FAD (H2), and SAM, whose availability has been optimized through engineered synthesis and recycling methods. This approach not only streamlines the production of essential cofactors but also significantly boosts the overall yield of PTOX precursors in yeast, paving the way for more efficient biosynthesis of valuable natural products. Chen et al. [156] highlighted the crucial role of managing the supply of cofactors utilized by heterologously expressed enzymes. Their integrated approach, which involved synthesizing and recycling three distinct cofactors, as well as enzyme overexpression, subcellular pathway reorganization, and the degradation of inhibitory by-products, led to a significant increase in the synthesis of CaA and FA. This strategy not only facilitates the high production of PTOX and other lignan-derived compounds but, when combined with traditional optimization methods, is likely to enhance the synthesis of many other natural products that require cofactors shortly.
Environmental conditions can impact the biosynthesis of secondary metabolites by altering gene expression through mechanisms involving miRNAs and transcription factors. The phenylpropanoid pathway is involved in secondary metabolite, formation, and miRNAs are known to participate in phenylpropanoid biosynthesis by targeting transcription factors. Thus, miRNA-based regulation of phenylpropanoid biosynthesis is extremely important [157].
The transcriptional activity of the key gene involved in lignan synthesis, pinoresinol-lariciresinol reductase (PLR), was examined using RT-PCR and promoter-reporter transgenesis during flax seed development. The LuPLR gene was found to be expressed in the seed coat, indicating its role in the production of the PLR enzyme in mature seeds, which supports its participation in SDG synthesis. To further investigate the function of the PLR enzyme in lignan biosynthesis, an RNAi approach utilizing hairpin dsRNA structures was employed for functional analysis of the LuPLR1 gene. Transgenic plants with silenced LuPLR1 genes showed a failure to accumulate SDG, and HPLC analysis revealed a significant reduction in SDG levels in these transgenic seeds [158]. They said that miRNAs can regulate lignan biosynthesis, and therefore they can be targeted to increase lignan production through the phenylpropanoid pathway.
The glycosylation of lignan is achieved by uridine glycosyltransferases (UGTs). It has been shown by Chen et al. [159] that the UGT71B5s are involved in lignan glycosylation in Isatis indigotica. Several other studies on the genetic engineering method of lignan enhancement of different plant species are given in Table 3.
6.2 Breeding Approach for Lignan Production
The presence of lignans, such as sesamin and sesamol, enhances the pharmaceutical value of sesame oil. Although wild sesame (Sesamum mulayanum) has lower oil yields, it contains significantly higher lignan levels. To improve the oil profile of cultivated Indian sesame (S. indicum), interspecific hybridization was performed between S. indicum and S. mulayanum. The selected F6 recombinant lines exhibited high oil content and a superior lignan profile, along with distinct phenotypic traits. Sesamin synthase, a crucial enzyme in the lignan biosynthetic pathway, was studied during seed development in both parent species using semi-quantitative and qRT-PCR analysis. The recombinant lines with elevated sesamin content showed increased expression of the sesamin synthase gene, which may serve as a potential candidate for allele-based molecular marker development in future sesame breeding programs [166]. The development of molecular markers has accelerated the process of flax molecular breeding and has improved yield and quality. Presently, simple sequence repeat (SSR) and single nucleotide polymorphism (SNP) markers in the whole genome have been developed for flax. Development and application of novel InDel (insertion/deletion) markers in flax (Linum usitatissimum L.) through whole-genome re-sequencing could be used for flax germplasm identification, genetic diversity analysis, and molecular marker-assisted breeding [29].
Additionally, breeding programs may use the identification of Linum spp. Cultivars with significant lignan content to introduce and enrich genes encoding pathway enzymes and produce cultivars with higher lignan content. There is genetic variability that can be used for breeding, as evidenced by the significant variations in lignan content between accessions and cultivars. The heredity of the primary lignan concentration in sesame seeds must be understood in order to develop sesame as a functional diet. There is little information on how lignan content is inherited. There have been reports of breeding initiatives in India to increase the lignan content of sesame [167]. The crossing of a parent with high lignan content with a low content tester produced offspring with lower lignan content than parents. Efforts should be made to cross two high-lignan-content parents to observe a potential benefit. Breeding sesame for lignan content is still in its infancy, and systematic efforts to target lignans have only recently begun. The development of sesame cultivars with higher lignan levels presents a valuable opportunity for farmers to tap into diverse industries, as highlighted by Khuimphukhieo et al. [168]. The heritability findings from the RIL population studied by Wu et al. [169] further support the notion that lignan-rich sesame lines can be effectively selected from appropriate parental crosses. This genetic insight allows for targeted breeding strategies that can enhance the nutritional and commercial value of sesame seeds.
Xiao et al. [74] reported that IiW.RKY34 expression, which is involved in lignan biosynthesis, activated Ii4CL3, a crucial rate-limiting enzyme in lignan biosynthesis. The expression of IiWRKY34 enhanced the polyploidy vigor of I. indigotica and its lignan content.
This review has examined the significant progress made in lignan research, emphasizing the enhanced production of lignans in crop plants. The findings illustrate the critical roles that lignans play in plant defense and their promising health benefits for humans, particularly in relation to cancer prevention and cardiovascular health. By employing innovative biotechnological approaches, such as genetic engineering and metabolic pathway optimization, researchers have opened new avenues for increasing lignan content. Looking ahead, the trend of enhancing lignan production offers promising opportunities for both agriculture and health. Lignan-rich foods not only serve as health supplements but also possess disease-fighting properties, while in plants, lignans play a role in stress tolerance. Future research should focus on optimizing production methods and investigating the ecological impact of lignan-enhanced crops. This will help ensure that these advancements support both agricultural sustainability and public health in a balanced and beneficial way.
We have presented a comprehensive analysis of various biotechnological approaches for enhancing lignan production in different plant species, with a focus on in vitro culture techniques, elicitor application, plant breeding, and genetic engineering. In vitro culture has been identified as a highly effective method for lignan production, particularly in Linum species, providing a viable alternative to rare plant species. This approach has enabled the successful production of lignans such as PTOX, MPTOX, dPTOX, SDG, and sesamin from Linum species, as well as from Sesamin indicum and Phyllanthus amarus. The use of biotic elicitors (e.g., yeast extract, NAA) and abiotic elicitors (e.g., UV, temperature, pH, and chemical agents such as Ag, Al2O3, SiO2, TiO2, ZnO, and MeJa) has been shown to significantly enhance lignan accumulation. Through the manipulation of metabolic pathways and the analysis of DNA, proteins, metabolites, and mRNA in target cells, substantial increases in the production of lignans could be achieved. Future research should focus on the discovery of novel enzymes involved in lignan biosynthesis and the development of methods to increase the production of lignan to exploit its antioxidant, anti-inflammatory, and anticancer properties. Fig. 6 summarizes the major elicitors for lignan production and the importance of lignan, which is already discussed in the text.
Figure 6: Different sources and elicitation methods for lignan production and their impact on humans
Acknowledgement: None.
Funding Statement: Ragini Singh is very thankful to the Jamia Hamdard University Grants Commission, India for research grant No. 162000681.
Author Contributions: The authors confirm contribution to the paper as follows: study conception and design: Ragini Singh, Noushina Iqbal; data collection: Ragini Singh, Noushina Iqbal; first draft manuscript preparation: Ragini Singh; writing review and editing: Noushina Iqbal, Sayeed Ahmad, Shahid Umar; supervision: Shahid Umar. All authors reviewed the results and approved the final version of the manuscript.
Availability of Data and Materials: The datasets generated during and/or analysed during the current study are available from the corresponding author on reasonable request.
Ethics Approval: Not applicable.
Conflicts of Interest: The authors declare no conflicts of interest to report regarding the present study.
References
1. Ushimaru R. Functions and mechanisms of enzymes assembling lignans and norlignans. Curr Opin Chem Biol. 2024;80:102462. [Google Scholar] [PubMed]
2. Ražná K, Nôžková J, Vargaová A, Harenčár Ľ, Bjelková M. Biological functions of lignans in plants. Agriculture (Pol’nohospodárstvo). 2021;67(4):155–65. [Google Scholar]
3. Saarinen NM, Warri A, Makela SI, Eckerman C, Reunanen M, Ahotupa M, et al. Hydroxymatairesinol, a novel enterolactone precursor with antitumor properties from coniferous tree (Picea abies). Nutr Cancer. 2000;36(2):207–16. [Google Scholar] [PubMed]
4. Jang WY, Kim MY, Cho JY. Antioxidant, anti-inflammatory, anti-menopausal, and anti-cancer effects of lignans and their metabolites. Int J Mol Sci. 2022;23(24):15482. [Google Scholar] [PubMed]
5. Tse TJ, Guo Y, Shim YY, Purdy SK, Kim JH, Cho JY, et al. Availability of bioactive flax lignan from foods and supplements. Critical Rev Food Sci Nutr. 2023;63(29):9843–58. [Google Scholar]
6. Al-Madhagy S, Ashmawy NS, Mamdouh A, Eldahshan OA, Farag MA. A comprehensive review of the health benefits of flaxseed oil in relation to its chemical composition and comparison with other omega-3-rich oils. Eur J Med Res. 2023;28(1):240. [Google Scholar] [PubMed]
7. Chhillar H, Chopra P, Ashfaq MA. Lignans from linseed (Linum usitatissimum L.) and its allied species: retrospect, introspect and prospect. Crit Rev Food Sci Nutr. 2021;61(16):2719–41. [Google Scholar] [PubMed]
8. Morya S, Menaa F, Jiménez-López C, Lourenço-Lopes C, BinMowyna MN, Alqahtani A. Nutraceutical and pharmaceutical behavior of bioactive compounds of miracle oilseeds: an overview. Foods. 2022;11(13):1824. [Google Scholar] [PubMed]
9. Anjum S, Komal A, Drouet S, Kausar H, Hano C, Abbasi BH. Feasible production of lignans and neolignans in root-derived in vitro cultures of flax (Linum usitatissimum L.). Plants. 2020;9(4):409. [Google Scholar] [PubMed]
10. Hong HT, Phan AD, O’hare TJ. Temperature and maturity stages affect anthocyanin development and phenolic and sugar content of purple-pericarp supersweet sweetcorn during storage. J Agric Food Chem. 2021;69(3):922–31. [Google Scholar] [PubMed]
11. Ahlawat YK, Singh M, Manorama K, Lakra N, Zaid A, Zulfiqar F. Plant phenolics: neglected secondary metabolites in plant stress tolerance. Braz J Bot. 2024;47(3):703–21. [Google Scholar]
12. Szopa A, Dziurka M, Kubica P, Jafernik K, Siomak O, Ekiert H. Stimulation of lignan production in Schisandra rubriflora in vitro cultures by elicitation. Molecules. 2022;27(19):6681. [Google Scholar] [PubMed]
13. Madke SS, Cherian KJ, Badere RS. Salicylic acid stimulates the biosynthesis of lignans in the cell suspension culture of Gmelina Arborea Roxb. J Ind Bot Soc. 2021;101(1and2):40–8. [Google Scholar]
14. Rodríguez-García C, Sánchez-Quesada C, Toledo E, Delgado-Rodríguez M, Gaforio JJ. Naturally lignan-rich foods: a dietary tool for health promotion? Molecules. 2019;24(5):917. [Google Scholar]
15. Adam-Dima I, Olteanu AA, Olaru OT, Popa DE, Purdel C. Methods of analysis of phytoestrogenic compounds: an up-to-date of the present state. Separations. 2024;11(7):205. [Google Scholar]
16. Dar AA, Arumugam N. Lignans of sesame: purification methods, biological activities and biosynthesis–a review. Bioorg Chem. 2013;50:1–10. [Google Scholar] [PubMed]
17. Motyka S, Skała E, Ekiert H, Szopa A. Health-promoting approaches of the use of chia seeds. J Funct Foods. 2023;103:105480. [Google Scholar]
18. Mikac S, Markulin L, Drouet S, Corbin C, Tungmunnithum D, Kiani R, et al. Bioproduction of anticancer podophyllotoxin and related aryltretralin-lignans in hairy root cultures of Linum flavum L. In: Ramawat KG, Ekiert HM, Goyal S, editors. Plant cell and tissue differentiation and secondary metabolites. Switzerland: Springer; 2021. p. 503–40. [Google Scholar]
19. Gupta R, Sethi KL. Conservation of medicinal plant resources in the Himalayan region. In: Jain SK, Mehra KL, editors. Conservation of tropical plant resources. Howarh: Botanical Survey of India; 1983. p. 101–7. [Google Scholar]
20. Shah Z, Gohar UF, Jamshed I, Mushtaq A, Mukhtar H, Zia-UI-Haq M, et al. Podophyllotoxin: history, recent advances and future prospects. Biomolecules. 2021;11(4):603. [Google Scholar] [PubMed]
21. Šamec D, Karalija E, Šola I, Vujčić Bok V, Salopek-Sondi B. The role of polyphenols in abiotic stress response: the influence of molecular structure. Plants. 2021;10:118. [Google Scholar] [PubMed]
22. Doussot J, Mathieu V, Colas C, Molinié R, Corbin C, Montguillon J, et al. Investigation of the lignan content in extracts from Linum, Callitris and Juniperus species in relation to their in vitro antiproliferative activities. Planta Med. 2017;34(06):574–81. [Google Scholar]
23. Koulman A, Bos R, Medarde M, Pras N, Quax WJ. A fast and simple GC MS method for lignan profiling in Anthriscus sylvestris and biosynthetically related plant species. Planta Med. 2001;67(9):858–62. [Google Scholar] [PubMed]
24. Tashackori H, Sharifi M, Ahmadian Chashmi N, Behmanesh M, Safaie N, Sagharyan M. Physiological, biochemical, and molecular responses of Linum album to digested cell wall of Piriformospora indica. Physiol Mol Biol Plants. 2021;27:2695–708. [Google Scholar] [PubMed]
25. Szopa A, Ekiert H. Lignans in Schisandra chinensis in vitro cultures. Die Pharmazie-An Int J Pharm Sci. 2011;66(8):633–4. [Google Scholar]
26. Nikule HA, Nitnaware KM, Chambhare MR, Kadam NS, Borde MY, Nikam TD. In-vitro propagation, callus culture and bioactive lignan production in Phyllanthus tenellus Roxb: a new source of phyllanthin, hypophyllanthin and phyltetralin. Sci Rep. 2020;1(10):10668. [Google Scholar]
27. Ionkova I, Fuss E. Influence of different strains of Agrobacterium rhizogenes on induction of hairy roots and lignan production in Linum tauricum ssp. tauricum. Pharmacog Mag. 2009;5(17):14–8. [Google Scholar]
28. Kancharla PK, Arumugam N. Variation of oil, sesamin, and sesamolin content in the germplasm of the ancient oilseed crop Sesamum indicum L. J Am Oil Chem’ Soc. 2020;97(5):475–83. [Google Scholar]
29. Andargie M, Vinas M, Rathgeb A, Möller E, Karlovsky P. Lignans of sesame (Sesamum indicum L.a comprehensive review. Molecules. 2021;26(4):883. [Google Scholar] [PubMed]
30. Global Market Size. Lignans Market revenue to hit $90 million by 2026. Says Global Market Insights, Inc. Available from: https://www.globenewswire.com/news-release/2020/03/25/2006051/0/en/Lignans-Market-revenue-to-hit-90-million-by-2026-Says-Global-Market-Insights-Inc.html. [Accessed 2024]. [Google Scholar]
31. Sahiner M, Yilmaz AS, Gungor B, Ayoubi Y, Sahiner N. Therapeutic and nutraceutical effects of polyphenolics from natural sources. Molecules. 2022;27(19):6225. [Google Scholar] [PubMed]
32. Kuijsten A, Arts IC, van’t Veer P, Hollman PC. The relative bioavailability of enterolignans in humans is enhanced by milling and crushing of flaxseed. The J Nutr. 2005;135(12):2812–6. [Google Scholar] [PubMed]
33. Khare B, Sangwan V, Rani V. Influence of sprouting on proximate composition, dietary fiber, nutrient availability, antinutrient, and antioxidant activity of flaxseed varieties. J Food Process Preserv. 2021;45(4):e15344. [Google Scholar]
34. Arab R, Casal S, Pinho T, Cruz R, Freidja ML, Lorenzo JM, et al. Effects of seed roasting temperature on sesame oil fatty acid composition, lignan, sterol and tocopherol contents, oxidative stability and antioxidant potential for food applications. Molecules. 2022;27(14):4508. [Google Scholar] [PubMed]
35. Povkhova LV, Pushkova EN, Rozhmina TA, Zhuchenko AA, Frykin RI, Novakovskiy RO, et al. Development and complex application of methods for the identification of mutations in the FAD3A and FAD3B genes resulting in the reduced content of linolenic acid in flax oil. Plants. 2022;12(1):95. [Google Scholar] [PubMed]
36. Teodor ED, Moroeanu V, Radu GL. Lignans from medicinal plants and their anticancer effect. Mini Rev Med Chem. 2020;20(12):1083–90. [Google Scholar] [PubMed]
37. Baldi S, Tristán Asensi M, Pallecchi M, Sofi F, Bartolucci G, Amedei A. Interplay between lignans and gut microbiota: nutritional, functional and methodological aspects. Molecules. 2023;28(1):343. [Google Scholar] [PubMed]
38. Shaik BB, Katari NK, Jonnalagadda SB. Role of natural products in developing novel anticancer agents: a perspective. Chem Biodiv. 2022;19(11):e202200535. [Google Scholar]
39. Mueed A, Deng Z, Korma SA, Shibli S, Jahangir M. Anticancer potential of flaxseed lignans, their metabolites and synthetic counterparts in relation with molecular targets: current challenges and future perspectives. Food Funct. 2023;14(5):2286–303. [Google Scholar] [PubMed]
40. Toulabi T, Yarahmadi M, Goudarzi F, Ebrahimzadeh F, Momenizadeh A, Yarahmadi S. Effects of flaxseed on blood pressure, body mass index, and total cholesterol in hypertensive patients: a randomized clinical trial. Explore. 2022;18(4):438–45. [Google Scholar] [PubMed]
41. Rawat M, Varshney A, Rai M, Chikara A, Pohty AL, Joshi A, et al. A comprehensive review on nutraceutical potential of underutilized cereals and cereal-based products. J Agric Food Res. 2023;12:100619. [Google Scholar]
42. Xia M, Chen Y, Li W, Qian C. Lignan intake and risk of cardiovascular disease and type 2 diabetes: a meta-analysis of prospective cohort studies. Int J Food Sci Nutr. 2023;74(4):501–9. [Google Scholar] [PubMed]
43. Singh A. Ethnopharmacological overview on natural lignan and neolignan for the treatment of cardiovascular diseases and their potential pharmacological mechanism. Asian J Res Chem. 2023;16(5):394–8. [Google Scholar]
44. Hu Y, Li Y, Sampson L, Wang M, Manson JE, Rimm E, et al. Lignan intake and risk of coronary heart disease. J Am College Cardiol. 2021;78(7):666–78. doi:10.1016/j.jacc.2021.05.049. [Google Scholar] [PubMed] [CrossRef]
45. Plaha NS, Awasthi S, Sharma A, Kaushik N. Distribution, biosynthesis and therapeutic potential of lignans. 3 Biotech. 2022;12(10):255. doi:10.1007/s13205-022-03318-9. [Google Scholar] [PubMed] [CrossRef]
46. Shahwan M, Alhumaydhi F, Ashraf GM, Hasan PM, Shamsi A. Role of polyphenols in combating Type 2 Diabetes and insulin resistance. Int J Biol Macromol. 2022;206(4):567–79. doi:10.1016/j.ijbiomac.2022.03.004. [Google Scholar] [PubMed] [CrossRef]
47. Barre DE, Mizier-Barre KA. Lignans’ potential in pre and post-onset type 2 diabetes management. Curr Diabetes Rev. 2020;16(1):2–11. doi:10.2174/1573399814666180914094520. [Google Scholar] [PubMed] [CrossRef]
48. Rowe IJ, Baber RJ. The effects of phytoestrogens on postmenopausal health. Climacteric. 2021;24(1):57–63. doi:10.1080/13697137.2020.1863356. [Google Scholar] [PubMed] [CrossRef]
49. Wang C, Mäkelä T, Hase T, Adlercreutz H, Kurzer MS. Lignans and flavonoids inhibit aromatase enzyme in human preadipocytes. J Steroid Biochem Mol Biol. 1994;50(3–4):205–12. [Google Scholar] [PubMed]
50. Musazadeh V, Nazari A, Natami M, Hajhashemy Z, Kazemi KS, Torabi F, et al. The effect of flaxseed supplementation on sex hormone profile in adults: a systematic review and meta-analysis. Front Nutr. 2023;10:1222584. [Google Scholar] [PubMed]
51. Karimi R, Rashidinejad A. Lignans: properties, health effects, and applications. In: Jafari SM, Rashidinejad A, Simal Gandara J, editors. Handbook of food bioactive ingredients: properties and applications. Cham: Springer International Publishing; 2022. p. 1–26. [Google Scholar]
52. dos Santos Maia M, Rodrigues GC, de Sousa NF, Scotti MT, Scotti L, Mendonça-Junior FJ. Identification of new targets and the virtual screening of lignans against Alzheimer’s disease. Oxid Med Cell Longev. 2020;2020(1):3098673. [Google Scholar] [PubMed]
53. Li Z, Zheng Y, Liu K, Liang Y, Lu J, Li Q, et al. Lignans as multi-targeted natural products in neurodegenerative diseases and depression: recent perspectives. Phytother Res. 2023;37(12):5599–621. [Google Scholar] [PubMed]
54. Xu XY, Wang DY, Li YP, Deyrup ST, Zhang HJ. Plant-derived lignans as potential antiviral agents: a systematic review. Phytochem Rev. 2022;21(1):239–89. [Google Scholar] [PubMed]
55. Sharma A, Shahzad B, Rehman A, Bhardwaj R, Landi M, Zheng B. Response of phenylpropanoid pathway and the role of polyphenols in plants under abiotic stress. Molecules. 2019;24(13):2452. [Google Scholar] [PubMed]
56. Khan I, Khan MA, Shehzad MA, Ali A, Mohammad S, Ali H, et al. Micropropagation and production of health promoting lignans in Linum usitatissimum. Plants. 2020;9(6):728. [Google Scholar] [PubMed]
57. Jan R, Asaf S, Numan M, Lubna, Kim KM. Plant secondary metabolite biosynthesis and transcriptional regulation in response to biotic and abiotic stress conditions. Agronomy. 2021;11(5):968. [Google Scholar]
58. Cheynier V, Comte G, Davies KM, Lattanzio V, Martens S. Plant phenolics: recent advances on their biosynthesis, genetics, and ecophysiology. Plant Physiol Biochem. 2013;72:1–20. [Google Scholar] [PubMed]
59. Jaiswal D, Pandey-Rai S, Agrawal SB. Untangling the UV-B radiation-induced transcriptional network regulating plant morphogenesis and secondary metabolite production. Environ Exp Bot. 2021;192:104655. [Google Scholar]
60. Lattanzio V, Ramawat KG, Merillon JM. Phenolic compounds: introduction. In: Ramawat KG, Merillon JM, editors. Natural products. Berlin, Heidelberg: Springer; 2013. p. 1543–80. [Google Scholar]
61. Fraser CM, Chapple C. The phenylpropanoid pathway in Arabidopsis. In: The arabidopsis book. USA: The American Society of Plant Biologists; 2011. vol. 9, p. e0152. doi:10.1199/tab.0152. [Google Scholar] [CrossRef]
62. Saini N, Anmol A, Kumar S, Bakshi M, Dhiman Z. Exploring phenolic compounds as natural stress alleviators in plants–a comprehensive review. Physiol Mol Plant Path. 2024;133(24):102383. doi:10.1016/j.pmpp.2024.102383. [Google Scholar] [CrossRef]
63. Ortiz A, Sansinenea E. Phenylpropanoid derivatives and their role in plants’ health and as antimicrobials. Curr Microbiol. 2023;80(12):380. doi:10.1007/s00284-023-03502-x. [Google Scholar] [PubMed] [CrossRef]
64. Osmakov DI, Kalinovskii AP, Belozerova OA, Andreev YA, Kozlov SA. Lignans as pharmacological agents in disorders related to oxidative stress and inflammation: chemical synthesis approaches and biological activities. Int J Mol Sci. 2022;23(11):6031. doi:10.3390/ijms23116031. [Google Scholar] [PubMed] [CrossRef]
65. Jha Y, Mohamed HI. Plant secondary metabolites as a tool to investigate biotic stress tolerance in plants: a review. Gesunde Pflanzen. 2022;74(4):771–90. doi:10.1007/s10343-022-00669-4. [Google Scholar] [CrossRef]
66. Haller HL, McGovran ER, Goodhue LD, Sullivan WN. The synergistic action of sesamin with pyrethrum insecticides. The J Org Chem. 1942;7(2):183–4. doi:10.1021/jo01196a011. [Google Scholar] [CrossRef]
67. Tak Y, Kaur M, Gautam C, Kumar R, Tilgam J, Natta S. Phenolic biosynthesis and metabolic pathways to alleviate stresses in plants. In: Lone R, Khan S, Al-Sadi AM, editors. Plant phenolics in abiotic stress management. Singapore: Springer Nature Singapore; 2023. p. 63–87. [Google Scholar]
68. Katarína R, Janka N, Angéla V, Marie B. Biological functions of lignans in plants. Agriculture. 2021;67(4):155–65. [Google Scholar]
69. Wan Y, Zhou Q, Zhao M, Hou T. Byproducts of sesame oil extraction: composition, function, and comprehensive utilization. Foods. 2023;12(12):2383. [Google Scholar] [PubMed]
70. Li Y, Wei J, Fang J, Lv W, Ji Y, Aioub AA, et al. Insecticidal activity of four lignans isolated from Phryma leptostachya. Molecules. 2019;24(10):1976. [Google Scholar] [PubMed]
71. Baghery MA, Kazemitabar SK, Dehestani A, Mehrabanjoubani P. Sesame (Sesamum indicum L.) response to drought stress: susceptible and tolerant genotypes exhibit different physiological, biochemical, and molecular response patterns. Physiol Mol Biol Plants. 2023;29(9):1353–69. [Google Scholar] [PubMed]
72. Kiani R, Arzani A, Mirmohammady Maibody SA. Polyphenols, flavonoids, and antioxidant activity involved in salt tolerance in wheat, Aegilops cylindrica and their amphidiploids. Front Plant Sci. 2021;25(12):646221. [Google Scholar]
73. Bhuyan U, Handique JG. Plant polyphenols as potent antioxidants: highlighting the mechanism of antioxidant activity and synthesis/development of some polyphenol conjugates. Stud Natural Products Chem. 2022;75:243–66. [Google Scholar]
74. Xiao Y, Feng J, Li Q, Zhou Y, Bu Q, Zhou J, et al. IiWRKY34 positively regulates yield, lignan biosynthesis and stress tolerance in Isatis indigotica. Acta Pharma Sin B. 2020;10(12):2417–32. [Google Scholar]
75. Deng J, Li W, Chen X, Liu D. WRKY11 up-regulated dirigent expression to enhance lignin/lignans accumulation in Lilium regale Wilson during response to Fusarium wilt. J Integr Agric. 2024;23(8):2703–22. [Google Scholar]
76. Corbin C, Drouet S, Markulin L, Auguin D, Lainé É, Davin LB, et al. genome-wide analysis of the flax (Linum usitatissimum L.) dirigent protein family: from gene identification and evolution to differential regulation. Plant Mol Biol. 2018;97:73–101. [Google Scholar] [PubMed]
77. Dong NQ, Lin HX. Contribution of phenylpropanoid metabolism to plant development and plant-environment interactions. J Integr Plant Biol. 2021;63(1):180–209. [Google Scholar] [PubMed]
78. Yadav V, Wang Z, Wei C, Amo A, Ahmed B, Yang X, et al. Phenylpropanoid pathway engineering: an emerging approach towards plant defense. Pathogens. 2020;9(4):312. [Google Scholar] [PubMed]
79. Vassão DG, Kim SJ, Milhollan JK, Eichinger D, Davin LB, Lewis NG. A pinoresinol-lariciresinol reductase homologue from the creosote bush (Larrea tridentata) catalyzes the efficient in vitro conversion of p-coumaryl/coniferyl alcohol esters into the allylphenols chavicol/eugenol, but not the propenylphenols p-anol/isoeugenol. Arch Biochem Biophy. 2007;465(1):209–18. [Google Scholar]
80. Debnath AJ, Harenčár Ĺ, Kučka M, Kovár M, Ivanišová E, Mistríková V, et al. A comparative analysis between two flax varieties indicates lignan-mediated salt stress adaptiveness. bioRxiv. 2024;13:2024–10. [Google Scholar]
81. Kwon M, Davin LB, Lewis NG. In situ hybridization and immunolocalization of lignan reductases in woody tissues: implications for heartwood formation and other forms of vascular tissue preservation. Phytochemistry. 2001;57(6):899–914. [Google Scholar] [PubMed]
82. Le Roy J, Huss B, Creach A, Hawkins S, Neutelings G. Glycosylation is a major regulator of phenylpropanoid availability and biological activity in plants. Front Plant Sci. 2016;7:735. [Google Scholar] [PubMed]
83. Sahraeian S, Rashidinejad A, Golmakani MT. Recent advances in the conjugation approaches for enhancing the bioavailability of polyphenols. Food Hydrocolloid. 2024;146(8):109221. doi:10.1016/j.foodhyd.2023.109221. [Google Scholar] [CrossRef]
84. Wei M, Yu C, Ge B, Liu Y, Zhang H, Duan C, et al. The phenylcoumaran benzylic ether reductase gene PtPCBER improves the salt tolerance of transgenic poplar through lignan-mediated reactive oxygen species scavenging. Environ Exp Bot. 2022;201(6):104946. doi:10.1016/j.envexpbot.2022.104946. [Google Scholar] [CrossRef]
85. Kitts DD, Yuan YV, Wijewickreme AN, Thompson LU. Antioxidant activity of the flaxseed lignan secoisolariciresinol diglycoside and its mammalian lignan metabolites enterodiol and enterolactone. Mol Cell Biochem. 1999;202(1/2):91–100. doi:10.1023/A:1007022329660. [Google Scholar] [PubMed] [CrossRef]
86. Touré A, Xueming X. Flaxseed lignans: source, biosynthesis, metabolism, antioxidant activity, bio-active components, and health benefits. Comprehens Rew Food Saf. 2010;9(3):261–9. doi:10.1111/j.1541-4337.2009.00105.x. [Google Scholar] [PubMed] [CrossRef]
87. Suja KP, Jayalekshmy A, Arumughan C, Takaku NT, Wattanawikkit P, Sakakibara N, et al. Antioxidant activity of sesame cake extract. Food Chem. 2005 Jun 1;91(2):213–9. Okunishi T, Takaku N, Wattanawikkit P, Sakakibara N, Suzuki S, Sakai F, Umezawa T, Shimada M. Lignan production in Daphne odora cell cultures. J Wood Sci. 2002;48:237–41. [Google Scholar]
88. Gülçin İ, Elias R, Gepdiremen A, Boyer L. Antioxidant activity of lignans from fringe tree (Chionanthus virginicus L.). Eur Food Res Technol. 2006;223:759–67. [Google Scholar]
89. Yamauchi S, Masuda T, Sugahara T, Kawaguchi Y, Ohuchi M, Someya T, et al. Antioxidant activity of butane type lignans, secoisolariciresinol, dihydroguaiaretic acid, and 7,7′-oxodihydroguaiaretic Acid. Biosci Biotechnol Biochem. 2008;72:2981–6. [Google Scholar] [PubMed]
90. Mwamba TM, Islam F, Ali B, Lwalaba JL, Gill RA, Zhang F, et al. Comparative metabolomic responses of low-and high-cadmium accumulating genotypes reveal the cadmium adaptive mechanism in Brassica napus. Chemosphere. 2020;250:126308. [Google Scholar] [PubMed]
91. Nadeem M, Ahmad W, Zahir A, Hano C, Abbasi BH. Salicylic acid-enhanced biosynthesis of pharmacologically important lignans and neo lignans in cell suspension culture of Linum ussitatsimum L. Eng Life Sci. 2019;19(3):168–74. [Google Scholar] [PubMed]
92. Chen R, Li Q, Tan H, Chen J, Xiao Y, Ma R, et al. Gene-to-metabolite network for biosynthesis of lignans in MeJA-elicited Isatis indigotica hairy root cultures. Front Plant Sci. 2015;6:952. [Google Scholar] [PubMed]
93. Hamade K, Fliniaux O, Fontaine JX, Molinié R, Otogo Nnang E, Bassard S, et al. NMR and LC-MS-based metabolomics to study osmotic stress in lignan-deficient flax. Molecules. 2021;26(3):767. [Google Scholar] [PubMed]
94. Charlet S, Bensaddek L, Raynaud S, Gillet F, Mesnard F, Fliniaux MA. An HPLC procedure for the quantification of anhydrosecoisolariciresinol. Application to the evaluation of flax lignan content. Plant Physiology Biochem. 2002;40(3):225–9. [Google Scholar]
95. SaraJliJa H, ČukelJ N, NoVotNi D, Mršić G, Brnčić M, Ćurić D. Preparation of flaxseed for lignan determination by gas chromatography-mass spectrometry method. Czech J Food Sci. 2012;30(1):45–52. [Google Scholar]
96. Bhatnagar AS, Hemavathy J, Gopala Krishna AG. Development of a rapid method for determination of lignans content in sesame oil. J Food Sci Technol. 2015;52:521–7. [Google Scholar]
97. Xiao HH, Lv J, Mok D, Yao XS, Wong MS, Cooper R. NMR applications for botanical mixtures: the use of HSQC data to determine lignan content in Sambucus williamsii. J Nat Prod. 2019;82(7):1733–40. [Google Scholar] [PubMed]
98. Patyra A, Kołtun-Jasion M, Jakubiak O, Kiss AK. Extraction techniques and analytical methods for isolation and characterization of lignans. Plants. 2022;11(17):2323. [Google Scholar] [PubMed]
99. Nadeem M, Taj Khan I, Khan F, Ajmal Shah M, Silva AS, Nabavi SF, et al. Lignans and flavonolignans. In: Silva AS, Nabavi SF, Saeedi M, Nabavi SM, editors. Recent advances in natural products analysis. Amsterdam, Netherland: Elsevier; 2020. p. 98–116. [Google Scholar]
100. Acemi A. Polymerization degree of chitosan affects structural and compositional changes in the cell walls, membrane lipids, and proteins in the leaves of Ipomoea purpurea: an FT-IR spectroscopy study. Int J Biol Macromol. 2020;162:715–22. [Google Scholar] [PubMed]
101. Dougué Kentsop RA, Consonni R, Alfieri M, Laura M, Ottolina G, Mascheretti I, et al. Linum lewisii adventitious and hairy-roots cultures as lignan plant factories. Antioxidants. 2022;11(8):1526. [Google Scholar]
102. Yildiz M, Özcan S, Er C. The effect of different explant sources on adventitious shoot regeneration in flax (Linum usitatissimum L.). Turk J Biol. 2002;26(1):37–40. [Google Scholar]
103. Samadi A, Carapetian J, Heidari R, Jafari M, Gorttapeh AH. Hairy root induction in Linum mucronatum ssp. mucronatum, an anti-tumor lignans producing plant. Notulae botanicae horti agrobotanici cluj-napoca. 2012;40(1):125–31. [Google Scholar]
104. Jafernik K, Szopa A, Barnaś M, Dziurka M, Ekiert H. Schisandra henryi C. B. Clarke in vitro cultures: a promising tool for the production of lignans and phenolic compounds. Plant Cell Tiss Organ Cult. 2020;143:45–60. [Google Scholar]
105. Esfandiari M, Sharifi M, Mohamadyar-Toupkanlou F, Hanaee-Ahwaz H, Yousefzadi M, Jafari A, et al. Optimization of cell/tissue culture of Linum persicum for production of lignans derivatives including Podophyllotoxin. Plant Cell, Tissue Organ Culture (PCTOC). 2018;133:51–61. [Google Scholar]
106. Asad B, Khan T, Gul FZ, Ullah MA, Drouet S, Mikac S, et al. Scarlet flax Linum grandiflorum (L.) in vitro cultures as a new source of antioxidant and anti-inflammatory lignans. Molecules. 2021;26(15):4511. [Google Scholar] [PubMed]
107. Sparzak-Stefanowska B, Krauze-Baranowska M. Phyllanthus amarus shoot cultures as a source of biologically active lignans: the influence of selected plant growth regulators. Sci Rep. 2022;12(1):11505. [Google Scholar] [PubMed]
108. Silva ST, de Assis RM, Coelho AD, Mendonça SC, Texeira LF, Bertolucci SK, et al. Accumulation of podophyllotoxin in the root culture of Hyptis suaveolens (L.) Poit: a potential natural lignan for clinically useful anticancer drugs. Biocat Agric Biotech. 2024;19:103416. [Google Scholar]
109. Federolf K, Alfermann AW, Fuss E. Aryltetralin-lignan formation in two different cell suspension cultures of Linum album: deoxypodophyllotoxin 6-hydroxylase, a key enzyme for the formation of 6-methoxypodophyllotoxin. Phytochemistry. 2007;68(10):1397–406. [Google Scholar] [PubMed]
110. Ahmadian Chashmi N, Sharifi M, Behmanesh M. Lignan enhancement in hairy root cultures of Linum album using coniferaldehyde and methylenedioxycinnamic acid. Preparat Biochem Biotech. 2016;46(5):454–60. [Google Scholar]
111. Oostdam A, Mol JN, van der Plas LH. Establishment of hairy root cultures of Linum flavum producing the lignan 5-methoxypodophyllotoxin. Plant Cell Rep. 1993;12:474–7. [Google Scholar] [PubMed]
112. Li B, Wang B, Li H, Peng L, Ru M, Liang Z, et al. Establishment of Salvia castanea Diels f. tomentosa Stib. hairy root cultures and the promotion of tanshinone accumulation and gene expression with Ag+, methyl jasmonate, and yeast extract elicitation. Protoplasma. 2016;253:87–100. [Google Scholar] [PubMed]
113. Zhao JL, Zou L, Zhang CQ, Li YY, Peng LX, Xiang DB, et al. Efficient production of flavonoids in Fagopyrum tataricum hairy root cultures with yeast polysaccharide elicitation and medium renewal process. Pharmacogn Mag. 2014;10(39):234. [Google Scholar] [PubMed]
114. Ming Q, Su C, Zheng C, Jia M, Zhang Q, Zhang H, et al. Elicitors from the endophytic fungus Trichoderma atroviride promote Salvia miltiorrhiza hairy root growth and tanshinone biosynthesis. J Exp Bot. 2013;18:5687–94. [Google Scholar]
115. Esmaeilzadeh Bahabadi S, Sharifi M, Ahmadian Chashmi N, Murata J, Satake H. Significant enhancement of lignan accumulation in hairy root cultures of Linum album using biotic elicitors. Acta Physiol Plant. 2014;36:3325–31. [Google Scholar]
116. Goncharuk EA, Saibel OL, Zaitsev GP, Zagoskina NV. The elicitor effect of yeast extract on the accumulation of phenolic compounds in Linum grandiflorum cells Cultured in vitro and their antiradical activity. Biol Bull. 2022;49(6):620–8. [Google Scholar]
117. Baldi A, Srivastava AK, Bisaria VS. Fungal elicitors for enhanced production of secondary metabolites in plant cell suspension cultures. In: Varma A, Kharkwal AC, editors. Symbiotic fungi: principles and practice. Berlin, Heidelberg: Springer; 2009. p. 373–80. [Google Scholar]
118. Kumar V, Rajauria G, Sahai V, Bisaria VS. Culture filtrate of root endophytic fungus Piriformospora indica promotes the growth and lignan production of Linum album hairy root cultures. Process Biochem. 2012;47(6):901–7. [Google Scholar]
119. Ahmad W, Zahir A, Nadeem M, Garros L, Drouet S, Renouard S, et al. Enhanced production of lignans and neolignans in chitosan-treated flax (Linum usitatissimum L.) cell cultures. Process Biochem. 2019;79:155–65. [Google Scholar]
120. Kamalipourazad M, Sharifi M, Maivan HZ, Behmanesh M, Chashmi NA. Induction of aromatic amino acids and phenylpropanoid compounds in Scrophularia striata Boiss. cell culture in response to chitosan-induced oxidative stress. Plant Physiol Biochem. 2016;107:374–84. [Google Scholar] [PubMed]
121. Farjaminezhad R, Garoosi G. Improvement and prediction of secondary metabolites production under yeast extract elicitation of Azadirachta indica cell suspension culture using response surface methodology. AMB Express. 2021;11(1):43. [Google Scholar] [PubMed]
122. Gai QY, Jiao J, Luo M, Wang W, Zhao CJ, Fu YJ, et al. UV elicitation for promoting astragaloside production in Astragalus membranaceus hairy root cultures with transcriptional expression of biosynthetic genes. Indus Crops Prod. 2016;84:350–7. [Google Scholar]
123. Zhang B, Zheng LP, Li WY, Wang JW. Stimulation of artemisinin production in Artemisia annua hairy roots by Ag-SiO2 core-shell nanoparticles. Curr Nanosci. 2013;9(3):363–70. [Google Scholar]
124. Harfi B, Khelifi-Slaoui M, Bekhouche M, Benyammi R, Hefferon K, Makhzoum A, et al. Hyoscyamine production in hairy roots of three Datura species exposed to high-salt medium. In Vitro Cell Dev Biol-Plant. 2016;52:92–8. [Google Scholar]
125. Cheruvathur MK, Thomas TD. Effect of plant growth regulators and elicitors on rhinacanthin accumulation in hairy root cultures of Rhinacanthus nasutus (L.) Kurz. Plant Cell Tiss Org. 2014;118:169–77. [Google Scholar]
126. Sykłowska-Baranek K, Łysik K, Jeziorek M, Wencel A, Gajcy M, Pietrosiuk A. Lignan accumulation in two-phase cultures of Taxus x media hairy roots. Plant Cell Tiss Org. 2018;133:371–84. [Google Scholar]
127. Jiao J, Gai QY, Wang X, Qin QP, Wang ZY, Liu J, et al. Chitosan elicitation of Isatis tinctoria L. hairy root cultures for enhancing flavonoid productivity and gene expression and related antioxidant activity. Ind Crops and Prod. 2018;124:28–35. [Google Scholar]
128. Samari E, Chashmi NA, Ghanati F, Sajedi RH, Gust AA, Haghdoust F, et al. Interactions between second messengers, SA and MAPK6 signaling pathways lead to chitosan-induced lignan production in Linum album cell culture. Ind Crops and Prod. 2022;177:114525. [Google Scholar]
129. Sagharyan M, Sharifi M, Samari E. Methyl jasmonate redirects the dynamics of carbohydrates and amino acids toward the lignans accumulation in Linum album cells. Plant Physiol Biochem. 2023;198:107677. [Google Scholar] [PubMed]
130. Andi SA, Gholami M, Ford CM, Maskani F. The effect of light, phenylalanine and methyl jasmonate, alone or in combination, on growth and secondary metabolism in cell suspension cultures of Vitis vinifera. J Photochem Photobiol. 2019;199:111625. [Google Scholar]
131. Benavides K, Sánchez-Kopper A, Jiménez-Quesada K, Perez R, Garro-Monge G. Evaluation of salicylic acid and methyl jasmonate as elicitors in Phyllanthus acuminatus hairy roots by non-targeted analysis using high-resolution mass spectrometry. Molecules. 2023;29(1):80. [Google Scholar] [PubMed]
132. Karimzadeh F, Haddad R, Garoosi GH, Khademian R. Effects of nanoparticles on activity of lignan biosynthesis enzymes in cell suspension culture of Linum usitatissimum L. Russ J Plant Physiol. 2019;66:756–62. [Google Scholar]
133. Hazra S, Bhattacharyya D, Chattopadhyay S. Methyl jasmonate regulates podophyllotoxin accumulation in Podophyllum hexandrum by altering the ROS-responsive podophyllotoxin pathway gene expression additionally through the down regulation of few interfering miRNAs. Front Plant Sci. 2017;8:164. [Google Scholar] [PubMed]
134. Abbasi BH, Zahir A, Ahmad W, Nadeem M, Giglioli-Guivarc’h N, Hano C. Biogenic zinc oxide nanoparticles-enhanced biosynthesis of lignans and neolignans in cell suspension cultures of Linum usitatissimum L. Artif Cells, Nanomed Biotech. 2019;47(1):1367–73. [Google Scholar]
135. Anjum S, Abbasi BH, Hano C. Trends in accumulation of pharmacologically important antioxidant-secondary metabolites in callus cultures of Linum usitatissimum L. Plant Cell Tiss Org. 2017;129:73–87. [Google Scholar]
136. Zaeem A, Drouet S, Anjum S, Khurshid R, Younas M, Blondeau JP, et al. Effects of biogenic zinc oxide nanoparticles on growth and oxidative stress response in flax seedlings vs. in vitro cultures: a comparative analysis. Biomolecules. 2020;10(6):918. [Google Scholar] [PubMed]
137. Renouard S, Corbin C, Drouet S, Medvedec B, Doussot J, Colas C, et al. Investigation of Linum flavum (L.) hairy root cultures for the production of anticancer aryltetralin lignans. Int J Mol Sci. 2018;19(4):990. [Google Scholar] [PubMed]
138. Sasheva P, Ionkova I, Stoilova N. Methyl jasmonate induces enhanced podophyllotoxin production in cell cultures of thracian flax (Linum thracicum ssp. thracicum). Nat Prod Commun. 2015;10(7):1225–8. [Google Scholar] [PubMed]
139. Ma R, Xiao Y, Lv Z, Tan H, Chen R, Li Q, et al. AP2/ERF transcription factor, Ii049, positively regulates lignan biosynthesis in Isatis indigotica through activating salicylic acid signaling and lignan/lignin pathway genes. Front Plant Sci. 2017;8:1361. [Google Scholar] [PubMed]
140. Shams-Ardakani M, Hemmati SH, Mohagheghzadeh AA. Effect of elicitors on the enhancement of podophyllotoxin biosynthesis in suspension cultures of Linum album. DARU. 2005;2(13):56–60. [Google Scholar]
141. Sharifi-Rad R, Esmaeilzadeh Bahabadi S, Samzadeh-Kermani A, Gholami M. The effect of non-biological elicitors on physiological and biochemical properties of medicinal plant Momordica charantia L. Iran J Sci Technol Trans A: Sci. 2020;5:1315–26. [Google Scholar]
142. Nourozi E, Hosseini B, Maleki R, Mandoulakani BA. Pharmaceutical important phenolic compounds overproduction and gene expression analysis in Dracocephalum kotschyi hairy roots elicited by SiO2 nanoparticles. Indus Crops Prod. 2019;133:435–46. [Google Scholar]
143. İşlek C, Ünal BT, Aydın S. Effects of zinc sulphate used as an elicitor on superoxide dismutase, peroxidase and total phenolic compounds of pepper calluses. Turk J Agric-Food Sci Technol. 2020;8(12):2780–4. [Google Scholar]
144. Chung IM, Rajakumar G, Subramanian U, Venkidasamy B, Thiruvengadam M. Impact of copper oxide nanoparticles on enhancement of bioactive compounds using cell suspension cultures of Gymnema sylvestre (Retz.). R Br App Sci. 2019;9(10):2165. [Google Scholar]
145. Kralova K, Jampilek J. Responses of medicinal and aromatic plants to engineered nanoparticles. Appl Sci. 2021;11(4):1813. [Google Scholar]
146. Jeong YJ, An CH, Park SC, Pyun JW, Lee J, Kim SW, et al. Methyl jasmonate increases isoflavone production in soybean cell cultures by activating structural genes involved in isoflavonoid biosynthesis. J Agric Food Chem. 2018;66(16):4099–105. [Google Scholar] [PubMed]
147. Shahhoseini R, Azizi M, Asili J, Moshtaghi N, Samiei L. Comprehensive assessment of phytochemical potential of Tanacetum parthenium (L.phenolic compounds, antioxidant activity, essential oil and parthenolide. J Essent Oil Bear Plants. 2019;22(3):614–29. [Google Scholar]
148. Howles PA, Sewalt VJ, Paiva NL, Elkind Y, Bate NJ, Lamb C, et al. Overexpression of L-phenylalanine ammonia-lyase in transgenic tobacco plants reveals control points for flux into phenylpropanoid biosynthesis. Plant Physiol. 1996;112(4):1617–24. [Google Scholar] [PubMed]
149. Xiao Y, Ji Q, Gao S, Tan H, Chen R, Li Q, et al. Combined transcriptome and metabolite profiling reveals that Ii PLR1 plays an important role in lariciresinol accumulation in Isatis indigotica. J Exp Bot. 2015;66(20):6259–71. [Google Scholar] [PubMed]
150. Chang J, Luo J, He G. Regulation of polyphenols accumulation by combined overexpression/silencing key enzymes of phyenylpropanoid pathway. Acta Biochim Biophys Sin. 2009;41(2):123–30. [Google Scholar] [PubMed]
151. Kim HJ, Ono E, Morimoto K, Yamagaki T, Okazawa A, Kobayashi A, et al. Metabolic engineering of lignan biosynthesis in Forsythia cell culture. Plant Cell Physiol. 2009;50(12):2200–9. [Google Scholar] [PubMed]
152. Zhang L, Chen J, Zhou X, Chen X, Li Q, Tan H, et al. Dynamic metabolic and transcriptomic profiling of methyl jasmonate-treated hairy roots reveals synthetic characters and regulators of lignan biosynthesis in Isatis indigotica Fort. Plant Biotech J. 2016;14(12):2217–27. [Google Scholar]
153. Amani S, Khademvatan S, Mohebodini M, Jafari M, Kumar V. Ficus carica hairy roots: In vitro: anti-leishmanial activity against: leishmania major: promastigotes and amastigotes. Asian Pacif J Trop Med. 2022;15(5):220–9. [Google Scholar]
154. Courdavault V, O’Connor SE, Jensen MK, Papon N. Metabolic engineering for plant natural products biosynthesis: new procedures, concrete achievements and remaining limits. Nat Prod Rep. 2021;38(12):2145–53. [Google Scholar] [PubMed]
155. Perrin J, Besseau S, Papon N, Courdavault V. Boosting lignan-precursor synthesis in yeast cell factories through co-factor supply optimization. Front Bioeng Biotechnol. 2022;10:1079801. [Google Scholar] [PubMed]
156. Chen R, Gao J, Yu W, Chen X, Zhai X, Chen Y, et al. Engineering cofactor supply and recycling to drive phenolic acid biosynthesis in yeast. Nature Chem Biol. 2022;18(5):520–9. [Google Scholar]
157. Ražná K, Harenčár Ľ, Kučka M. The involvement of microRNAs in plant lignan biosynthesis-current view. Cells. 2022;11(14):2151. [Google Scholar] [PubMed]
158. Hano C, Martin I, Fliniaux O, Legrand B, Gutierrez L, Arroo RR, et al. Pinoresinol-lariciresinol reductase gene expression and secoisolariciresinol diglucoside accumulation in developing flax (Linum usitatissimum) seeds. Planta. 2006;224:1291–301. [Google Scholar] [PubMed]
159. Chen X, Chen J, Feng J, Wang Y, Li S, Xiao Y, et al. Tandem UGT71B5s catalyze lignan glycosylation in Isatis indigotica with substrates promiscuity. Front Plant Sci. 2021;12:637695. [Google Scholar] [PubMed]
160. Javadian N, Karimzadeh G, Sharifi M, Moieni A, Behmanesh M. In vitro polyploidy induction: changes in morphology, podophyllotoxin biosynthesis, and expression of the related genes in Linum album (Linaceae). Planta. 2017;245:1165–78. [Google Scholar] [PubMed]
161. Markulin L, Drouet S, Corbin C, Decourtil C, Garros L, Renouard S, et al. The control exerted by ABA on lignan biosynthesis in flax (Linum usitatissimum L.) is modulated by a Ca2+ signal transduction involving the calmodulin-like LuCML15b. J Plant Physiol. 2019;236:74–87. [Google Scholar] [PubMed]
162. Tamagnone L, Merida A, Parr A, Mackay S, Culianez-Macia FA, Roberts K, et al. The AmMYB308 and AmMYB330 transcription factors from Antirrhinum regulate phenylpropanoid and lignin biosynthesis in transgenic tobacco. Plant Cell. 1998;10(2):135–54. [Google Scholar] [PubMed]
163. Corbin C, Decourtil C, Marosevic D, Bailly M, Lopez T, Renouard S, et al. Role of protein farnesylation events in the ABA-mediated regulation of the pinoresinol-lariciresinol Reductase 1 (LuPLR1) gene expression and lignan biosynthesis in flax (Linum usitatissimum L.). Plant Physiol Bochem. 2013;72:96–111. [Google Scholar] [PubMed]
164. Koyama T, Matsumoto E, Okuda T, Murata J, Horikawa M, Hata N, et al. Transgenic Forsythia plants expressing sesame cytochrome P450 produce beneficial lignans. Sci Rep. 2022;12(1):10152. [Google Scholar] [PubMed]
165. Pei Y, Cao W, Yu W, Peng C, Xu W, Zuo Y, et al. Identification and functional characterization of the dirigent gene family in Phryma leptostachya and the contribution of PlDIR1 in lignan biosynthesis. BMC Plant Biol. 2023;23(1):291. [Google Scholar] [PubMed]
166. Usman SM, Viswanathan P, Manonmani S, Uma D. Genetic studies on sesamin and sesamolin content and other yield attributing characters in sesame (Sesamum indicum L.). El J Plant Breed. 2020;11:132–8. [Google Scholar]
167. Jiang H, Pan G, Liu T, Chang L, Huang S, Tang H, et al. Development and application of novel InDel markers in flax (Linum usitatissimum L.) through whole-genome re-sequencing. Gen Res Crop Evol. 2022;69(4):1471–83. [Google Scholar]
168. Khuimphukhieo I, Khaengkhan P. Combining ability and heterosis of sesamin and sesamolin content in sesame. Sabrao J Breed Gen. 2018;50:180–91. [Google Scholar]
169. Wu K, Wu WW, Yang MM, Liu HY, Hao GC, Zhao YZ. QTL mapping for oil, protein and sesamin contents in seeds of white sesame. Acta Agron Sin. 2017;43(7):1003–11 (In Chinese). doi:10.3724/SP.J.1006.2017.01003. [Google Scholar] [CrossRef]
Cite This Article
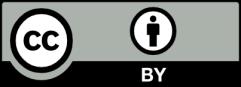
This work is licensed under a Creative Commons Attribution 4.0 International License , which permits unrestricted use, distribution, and reproduction in any medium, provided the original work is properly cited.