Open Access
VIEWPOINT
Recent Breakthroughs in the Characterization of Abscisic Acid Efflux Transporters: Shedding New Light on Abscisic Acid Dynamics and Regulation
UMR 6553 Ecosystems-Biodiversity-Evolution, University of Rennes/CNRS, Campus de Beaulieu, bâtiment 14A, Rennes, F-35042, France
* Corresponding Author: Ivan Couée. Email:
(This article belongs to the Special Issue: Regulation of Phytohormone Biosynthesis and Signaling)
Phyton-International Journal of Experimental Botany 2024, 93(11), 2707-2714. https://doi.org/10.32604/phyton.2024.058101
Received 04 September 2024; Accepted 06 November 2024; Issue published 30 November 2024
Abstract
The 15-carbon terpenoid abscisic acid (ABA) acts in vascular plants as a versatile hormone playing essential roles in reproductive development, vegetative development and growth, stress-development interactions, and physiological responses to abiotic and biotic stresses. Over the past 60 years, ABA dynamics, regulation, and responses have been progressively characterized: synthesis, transport and translocation, conjugation and deconjugation, metabolism, sensing, signal transduction, and downstream responses. In this context, the discovery of ABA exporters and importers has added novel dimensions to the understanding of ABA regulation. Moreover, since the initial discovery of the adenosine triphosphate-binding cassette (ABC) AtABCG25 exporter and AtABCG40 importer, several transporters of ABA have been characterized. As ABA is synthesized within cells, efflux transport of ABA to extracellular spaces is an important controlling step before ABA movements from cell to cell and from source to target. Until now, structural and functional mechanistic interactions between ABA and its transporters have remained poorly understood. Recent structure-function studies have unveiled key features of the mechanisms and functional dynamics of ABA-transporter interactions in the case of the ABCG25 exporter. ABCG25 has been established as a bona fide ABA efflux transporter involved in signal transmission leading to stomatal closure. It has also been shown to transport ABA-glucosyl ester (ABA-GE) conjugated form, thus implying involvement in conjugation and deconjugation dynamics. All of these mechanisms are essential for distribution throughout source tissues, source-to-target transmission, distribution throughout target tissues, and signal shape kinetics in target cells. This opinion piece emphasizes the pivotal role of transport discoveries in the history of ABA research, and how recent studies on ABA and ABA-GE transporters yield a more integrative vision of ABA regulation and adaptive responses, open new avenues of research on signaling crosstalks, and constitute opportunities as well as challenges for crop improvement strategies.Keywords
The 15-carbon terpenoid abscisic acid (ABA) acts in vascular plants as a versatile hormone involved in developmental, physiological, and stress-adaptive processes [1–5]. It plays essential roles in reproductive development [1,5], vegetative development and growth [1,5], stress-development interactions [1–3], physiological responses to abiotic and biotic stresses [1–4], and climate change resilience [4,6,7]. Over the past 60 years, the processes that sustain ABA dynamics and responses have been elucidated: synthesis and catabolism [8], transport and translocation [9], conjugation and deconjugation [1,10], sensing [11–14], signal transduction [1,8,11,13], downstream responses [5,8,15]. In parallel, the components of the underlying genomic, proteomic, and metabolomic machinery have been progressively identified and characterized [1,8].
In this context, identification of exporters and importers of ABA has highlighted the multiple dimensions of ABA regulation [16–18]. Since the initial discovery of the adenosine triphosphate-binding cassette (ABC) AtABCG25 exporter [17] and AtABCG40 importer [16], several other transporters of ABA have been characterized [18]. Transport and translocation of ABA have thus been shown to be related to an array of efflux and influx transporters [16–20] that are involved in cell-to-cell and tissue-to-tissue processes [19,21,22]. Such transmembrane transporters of ABA have been characterized not only in Arabidopsis thaliana [9,16,17] but also in Brachypodium distachyon [23], Medicago truncatula [24], and Oryza sativa [25].
Until now, the structural and functional mechanistic interactions between ABA and its transporters have remained poorly understood. Several studies have very recently unveiled the mechanisms and functional dynamics of ABA-transporter interactions in the case of ABCG25 [18,20–22], which is the first ABA exporter that had been genetically identified in plants 15 years ago [17]. These structure-function studies have established the mechanistic features and the bona fide role of ABCG25 as an ABA efflux transporter [18,20,26] involved in signal transmission leading to stomatal closure [17,21]. It has also been shown that ABCG25 can transport the ABA-glucosyl ester (ABA-GE) conjugated form, thus implying involvement in conjugation and deconjugation dynamics [21,22].
All of these mechanisms must play essential roles in the spatial and temporal regulation of ABA signaling and homeostasis [27]. Recent reviews [5,7,10,27,28] have addressed this issue of ABA signaling and homeostasis with novel integrative and holistic outlooks taking into account transport processes. These novel outlooks on ABA signaling and homeostasis should lead to a better understanding of ABA-mediated adaptive responses to stresses [5,10] and to further strategies of crop adaptation in the context of climate change and agricultural security [12,29].
2 ABA Signaling Relies on Complex Protein Networks Functioning in the Whole-Plant Context of ABA Dynamics
Discovery of the core sensing and signaling pathway has been a decisive step in the understanding of ABA action [1,11,12,14]. ABA acts by eliciting the activation of a downstream kinase, which triggers the activity of regulatory proteins. Sensing of ABA is carried out by intracellular soluble pyrabactin resistance 1/PYR1-like/regulatory component of ABA receptor (PYR/PYL/RCAR) receptor proteins. The binding of ABA within the PYR/PYL/RCAR protein pocket leads to the inhibition of type 2C phosphatases (PP2Cs) that otherwise repress downstream ABA signaling transduction. Formation of the PYL–ABA–PP2C complex relieves sucrose non-fermenting 1-related protein kinase 2 (SnRK2) from the inhibitory complex with PP2C. In interaction with Raf-like protein kinases, ABA-activated SnRK2s activate transcription factors, ion channels, and additional proteins, thus initiating ABA responses [1,11,12,14].
However, this core mechanism does not function as a unilinear input-output system. All of the protein components of the pathway exist as a diversity of isoforms [11,12,14]. PYLs, PP2Cs, and SnRK2s consist of families of proteins, whose diversity may be related to the sub-functionalization of parallel ABA signaling pathways and/or to the robustness and plasticity of ABA signaling [1,12,14].
Moreover, activation of the whole sensing and signaling pathway depends on the availability of bioactive ABA that can reach its receptors [1]. This pool of bioactive ABA in interaction with the sensing machinery is the dynamic result of multiple processes, as illustrated in Fig. 1: (i) ABA synthesis, (ii) ABA catabolism, (iii) formation of ABA conjugates, of which ABA-GE is the major form, (iv) release of free ABA from conjugates. All of the enzymatic steps involved can be subjected to biochemical and molecular regulations [1]. At a higher level, the availability of bioactive ABA fluctuates, as the global pool of ABA depends on the developmental, nutritional, environmental, and stress-exposure status of the plant [1,3].
Figure 1: Integration of import and export processes of ABA and conjugated ABA in the global dynamics and sensing of ABA at cell level. ABA and conjugated ABA (small orange diamonds), importers (double grey ellipses), exporters (double purple ellipses) and cell domain (double green square) are shown. Processes and abbreviations are as described in the text
Finally, by its hormonal functions, ABA action depends on communication between source and target tissues [27]. However, it is not yet clear which cells synthesize ABA under normal conditions and stress conditions [27]. Furthermore, ABA movement within the plant is not straightforward and involves different relationships between tissues, with xylem and phloem saps playing essential roles in whole-plant transmission [27].
ABA is partially in the protonated uncharged form ABAH in the acidic apoplast environment [26]. ABA can thus diffuse freely across the plasma membrane, but these passive transfers are rate-limited and show poor efficiency [26]. This is why the contemporaneous discovery of the first ABA transmembrane transporters in Arabidopsis, the AtABCG25 exporter [17] and the AtABCG40 importer [16], revealed the essential link that was missing between ABA dynamics and ABA sensing. This breakthrough was reported by teams respectively led by Kazuo Shinozaki and by Enrico Martinoia and Youngsook Lee in 2010 in the same issue of Proceedings of the National Academy of Sciences [16,17], i.e., only one year after the discovery of the core sensing and signaling pathway [13].
AtABCG25 was described as an exporter of ABA from vascular tissue leading to stomatal regulation [17], whereas AtABCG40 was described as an importer of ABA leading to ABA delivery into target cells [16]. Since these landmark discoveries, several other ABA transporters have been identified in Arabidopsis thaliana, such as AtABCG31 and AtDTX50 as exporters, and AtABCG30, AtABCG17/18, and NRT1.2/NPF4.6 as importers [18,19].
The core pathway of ABA sensing and signaling should not therefore be considered in isolation. As illustrated in Fig. 1, it is integrated not only within the networks of ABA metabolism and conjugation-deconjugation but also within networks of ABA transport and diffusion. As ABA is synthesized within cells, the efflux transport of ABA to extracellular spaces must be an important controlling step before ABA moves from cell to cell and from source tissues to target tissues [20]. In parallel, as ABA cannot readily and easily cross cell membranes, the influx transport of ABA into the cytosol must be an important controlling step prior to interactions with the intracellular sensing machinery [27]. Furthermore, this integration of ABA signaling with ABA transport processes involves a complex array of different transporters [16–20].
These transport processes and the diversity of associated transporters, expressed and acting in different sets of cell populations, are bound to determine ABA distribution throughout source tissues, the source-to-target transmission of ABA, ABA distribution throughout target tissues, and signal shape kinetics of ABA in target cells as a result of influx/efflux equilibria [10,15,27]. Such transporters are therefore essential for the spatialization and temporal regulation of ABA homeostasis and signaling [10].
3 Recent Breakthroughs in the Characterization of ABA Transporters
Identification of ABA transporters [16–18] has highlighted the important role of the transmembrane domain (TMD)-ABC transporters [30], which typically present TMDs and nucleotide-binding domains (NBD). In particular, ABC transporters belonging to the G subfamily (ABCG) may constitute the largest group of ABA transporters [18,22].
However, despite ongoing interest in these ABCG transporters of ABA, structural insights have lagged since the discovery of AtABCG25 and AtABCG40 [16,17]. It is only very recently, nearly 15 years after initial identification, that mechanistic description of ABA transport was completed in the case of ABCG25 exporter [18,20,26]. Three recent studies using protein purification in micelle environments, cryogenic electron microscopy, and ectopic expression in different host cells (Xenopus oocytes, Sf9 insect cells, tobacco protoplasts) have characterized the molecular architecture of AtABCG25 for ABA binding, adenosine 5′-triphosphate (ATP) binding and ATP hydrolysis [18,20,26]. It must be kept in mind that, in such analyses, reconstitution of the transporter in experimental amphipathic environments or expression in heterologous vectors may result in artefactual discrepancies with in planta behavior. Notwithstanding these caveats, these independent studies give a coherent description of ABCG25 functioning. The inward-outward translocation of ABA is carried out between a cytoplasmic gate and an apoplastic gate, whose functioning requires conformational changes of protein domains [18,20,26]. After the closure of the NBD domain is induced by ATP, the resulting conformational change is transmitted to TMD domains, thus triggering a series of transitions that facilitate ABA translocation [18,20,26].
In parallel, additional functional studies, including measurements of transporter activity in tobacco cells, Arabidopsis cells, and yeast cells, have established that ABCG25 directly exports not only ABA but also ABA-GE [21]. Complementary analysis of abcg25 mutants further established that ABCG25 is involved in the accumulation of both ABA and ABA-GE in root tissues and xylem sap upon drought stress [21], thus emphasizing the role of ABCG25 in root-to-shoot communication and control of stomatal movements.
Finally, using a range of protein-protein interaction methods, Zhou et al. [22] have shown that ABCG25 can heterodimerize at the endoplasmic reticulum membrane with ABCG16, which they proved to be a plasma membrane ABA exporter besides its previously-known activity as a jasmonate exporter. Moreover, they also established that ABCG16-ABCG25 heterodimers could mediate specific entry of ABA-GE, rather than free ABA, into the endoplasmic reticulum compartment [22]. In other words, ABCG16 and ABCG25 could synergistically function at the plasma membrane for ABA efflux, whereas ABCG16-ABCG25 heterodimers would carry out ABA-GE transport in the endoplasmic reticulum [22].
At the same time, further progress was made to understand the regulatory context of ABCG25 expression, with the demonstration that strigolactones promote localization of ABCG25 at the plasma membrane in root cells [31], thus establishing links between osmotic stress, strigolactone regulations and ABA action on root cells.
In sum, knowledge of ABCG25 now covers binding and catalytic sites of ABA transmembrane efflux translocation [18,20,26], subcellular compartmentalization in the plasma membrane and endoplasmic reticulum [22], involvement in ABA-GE translocations [21,22], capacity to co-function with other ABCGs such as ABCG16 [22], and patterns of expression [17,22,31]. These newly-discovered features link ABCG25 functioning with novel regulatory aspects, such as ABA/ABA-GE balance [22], whole-plant transmission of ABA-GE [21], protein-protein interactions [22], ATP and energy metabolism [18,20,26], potential sterol binding [26] and potential disulfide dynamics [26].
4 Novel Insights and Potential Lines of Investigation for Future Research: Novel Regulatory Features and Mechanisms of Signaling Crosstalks
All of these breakthroughs are bringing about novel insights into different aspects of ABA functioning in plants: (i) ABA and ABA-GE translocation from source cells to apoplast and saps [21], (ii) dynamic control (kinetics, amplification, attenuation) of bioactive ABA levels through concomitant activities of influx, conjugation, deconjugation, metabolism, and efflux [9,22], (iii) spatiotemporal distribution of ABA and ABA-GE in source tissues and target tissues [9,21], (iv) mechanisms of spatialization of ABA responses at tissue level [10,21,27]. It will therefore be a major challenge to understand fully how these metabolic, transport, sensing, and signaling dimensions of ABA functioning are regulated and integrated [1,8] and how this integration is modulated under non-stress vs. stress conditions [21].
The most recent knowledge on ABCG25 [18,20–22,26] agrees with the regulatory features of plant ABC transporters [30] and points out potential regulations through protein phosphorylation, protein-protein interactions, membrane microdomain interactions, and redox status. Furthermore, all of the protein components involved in ABA dynamics exist as multiple isoforms [11,14,30,32], thus implying functional redundancy, sub-functionalization, combinatorial interactions, robustness, and/or plasticity. The complete picture of integration between metabolism, transport, sensing, and signaling will therefore require a better understanding of the functional specificities of existing isoforms. This ought to include an analysis of the full range of ABA transporters in terms of substrate specificities and regulatory mechanisms.
Furthermore, these recent discoveries on ABA transporters point out novel potential links with signaling crosstalks [18,20,22,26,31]. ABA functioning is known to be involved in numerous crosstalks, with other hormones [5], peptide signals [21], light [1,5], carbon and energy signals [33,34], sucrose [5], reactive oxygen species (ROS) [1], nitric oxide (NO) [1], and mineral nutrition signals [28]. These crosstalks are related to the capacity to perceive and adapt to combinations of stresses [5–7,34,35], and therefore to adapt to climate change [4,7]. However, they rely on a diversity of interfering mechanisms at different levels of transmission processes and signaling cascades. Strikingly, the low-affinity nitrate transporter NRT1.2/NPF4.6 functions as a dual importer that also carries out the transport of ABA, thus participating in the integration of ABA and nitrate signaling [28]. It would be of great interest to investigate further how transport-mediated steps of ABA action are involved in such crosstalk mechanisms and how they may contribute to resilience to stress combinations and climate change [6,28].
5 Opportunities and Challenges for Crop Improvement
The discovery of novel genetically encoded entities and mechanisms systematically raises the possibility of designing novel markers, treatments, or genetic tools for crop breeding and crop agricultural management [3,12,36]. The involvement of transport mechanisms in ABA-mediated resilience to abiotic stresses (drought, cold, heat, salinity) and climate change could lead to potential applications in plant breeding, agriculture, and food security in the context of climate change [18,26]. The dimension of transport-mediated control of ABA distribution and action may be considered to provide relevant genetic or functional steps for targeting genetic improvement strategies for stress tolerance and climate change resilience [18,26]. Depending on climate conditions, improvement of crop performance entails enhancing or reducing ABA responses [12]. Such modulation of ABA responses could be achieved through the modulation of ABA allocation and spatialization between sources and targets and therefore through genetic variation of transporter genes. It has been shown in the case of cytokinins that modulation of hormone spatialization led to improved grain development and salt tolerance in rice [37].
On the other hand, the characterization of multiple transport processes, as occurs with ABA and ABA-GE, highlights the complexity of phytohormone action, including highly precise responses at cell-specific levels [32] and highly dynamic and multi-dimensional responses, rather than off-on and unilinear switches [27]. In such complex systems, as pointed out by Pri-Tal et al. [14] in the case of ABA multiple receptors, overmanipulating single elements can result in tipping over endogenous balances, thus triggering non-adaptive or deleterious responses. Furthermore, as diverse ABA signaling proteins may be involved in various crosstalks with other signaling pathways, perturbations of these crosstalks could lead to negative trade-offs [5].
It may therefore be argued that the characterization of transport-related controls highlights novel layers of robust homeostasis and feedback [10,27,32] that may counter-regulate single-target genetic improvement, induce negative trade-offs in varieties under selection, or amplify disruptive effects of single-target genetic improvement. At any rate, the discovery of complex, multi-dimensional, and redundant networks of regulation [27,32] is a clear incentive to integrate genetic, structural, and functional studies with ecological and evolutionary approaches. This should lead to holistic approaches to genetic breeding that take into account the multi-dimensional homeostasis of plants and do not disrupt endogenous coordinated responses of the plant organism [5]. At this stage of progress in the field of ABA studies, integrative and holistic approaches are required, at the levels of genetic improvement, genotyping, and phenotyping, in order to link crop breeding with the maintenance of multi-dimensional ABA functioning and homeostasis [5,32,36].
Acknowledgement: The author wishes to acknowledge the institutional support of the University of Rennes (Brittany, France) and the Centre National de la Recherche Scientifique (CNRS, France).
Funding Statement: The author received no specific funding for this study.
Availability of Data and Materials: Data and materials sharing is not applicable as no new data or materials were generated.
Ethics Approval: Not applicable.
Conflicts of Interest: The author declares that they have no conflicts of interest to report regarding the present study.
References
1. Chen K, Li GJ, Bressan RA, Song CP, Zhu JK, Zhao Y. Abscisic acid dynamics, signaling, and functions in plants. J Integr Plant Biol. 2020;62(1):25–54. doi:10.1111/jipb.12899. [Google Scholar] [PubMed] [CrossRef]
2. Kumar R, Dasgupta I. Abscisic acid: an emerging player in plant-virus interactions. Plant Physiol Biochem. 2024;215:109046. doi:10.1016/j.plaphy.2024.109046. [Google Scholar] [PubMed] [CrossRef]
3. Zhu JK. Abiotic stress signaling and responses in plants. Cell. 2016;167:313–24. doi:10.1016/j.cell.2016.08.029. [Google Scholar] [PubMed] [CrossRef]
4. Bigot S, Buges J, Gilly L, Jacques C, Le Boulch P, Berger M, et al. Pivotal roles of environmental sensing and signaling mechanisms in plant responses to climate change. Global Change Biol. 2018;24:5573–89. doi:10.1111/gcb.14433. [Google Scholar] [PubMed] [CrossRef]
5. Kavi Kishor PB, Tiozon RN Jr, Fernie AR, Sreenivasulu N. Abscisic acid and its role in the modulation of plant growth, development, and yield stability. Trends Plant Sci. 2022;27(12):1283–95. doi:10.1016/j.tplants.2022.08.013. [Google Scholar] [PubMed] [CrossRef]
6. Segarra-Medina C, Alseekh S, Fernie AR, Rambla JL, Pérez-Clemente RM, Gómez-Cádenas A, et al. Abscisic acid promotes plant acclimation to the combination of salinity and high light stress. Plant Physiol Biochem. 2023;203:108008. doi:10.1016/j.plaphy.2023.108008. [Google Scholar] [PubMed] [CrossRef]
7. Jing Z, Liu N, Zhang Z, Hou X. Research progress on plant responses to stress combinations in the context of climate change. Plants. 2024;13:469. doi:10.3390/plants13040469. [Google Scholar] [PubMed] [CrossRef]
8. Finkelstein R. Abscisic acid synthesis and response. The Arabidopsis Book. 2013;11:e0166. doi:10.1199/tab.0166. [Google Scholar] [PubMed] [CrossRef]
9. Kuromori T, Seo M, Shinozaki K. ABA transport and plant water stress responses. Trends Plant Sci. 2018;23:513–22. doi:10.1016/j.tplants.2018.04.001. [Google Scholar] [PubMed] [CrossRef]
10. Wong C, Alabadi D, Blázquez MA. Spatial regulation of plant hormone action. J Exp Bot. 2023;74(19):6089–103. doi:10.1093/jxb/erad244. [Google Scholar] [PubMed] [CrossRef]
11. Raghavendra AS, Gonugunta VK, Christmann A, Grill E. ABA perception and signalling. Trends Plant Sci. 2010;15:395–401. doi:10.1016/j.tplants.2010.04.006. [Google Scholar] [PubMed] [CrossRef]
12. Miao C, Xiao L, Hua K, Zou C, Zhao Y, Bressan RA, et al. Mutations in a subfamily of abscisic acid receptor genes promote rice growth and productivity. Proc Natl Acad Sci U S A. 2018;115(23):6058–63. doi:10.1073/pnas.1804774115. [Google Scholar] [PubMed] [CrossRef]
13. Cutler SR, Rodriguez PL, Finkelstein RR, Abrams SR. Abscisic acid: emergence of a core signaling network. Annu Rev Plant Biol. 2010;61:651–79. doi:10.1146/arplant.2010.61.issue-1. [Google Scholar] [CrossRef]
14. Pri-Tal O, Sun Y, Dadras A, Fürst-Jansen JMR, Zimran G, Michaeli D, et al. Constitutive activation of ABA receptors in Arabidopsis reveals unique regulatory circuitries. New Phytol. 2024;241:703–14. doi:10.1111/nph.19363. [Google Scholar] [PubMed] [CrossRef]
15. Verma V, Ravindran P, Kumar PP. Plant hormone-mediated regulation of stress responses. BMC Plant Biol. 2016;16:86. doi:10.1186/s12870-016-0771-y. [Google Scholar] [PubMed] [CrossRef]
16. Kang J, Hwang JH, Lee M, Kim YY, Assmann SM, Martinoia E, et al. PDR-type ABC transporter mediates cellular uptake of the phytohormone abscisic acid. Proc Natl Acad Sci U S A. 2010;107(5):2355–60. doi:10.1073/pnas.0909222107. [Google Scholar] [PubMed] [CrossRef]
17. Kuromori T, Miyaji T, Yabuuchi H, Shimizu H, Sugimoto E, Kamiya A, et al. ABC transporter AtABCG25 is involved in abscisic acid transport and responses. Proc Natl Acad Sci U S A. 2010;107(5):2361–6. doi:10.1073/pnas.0912516107. [Google Scholar] [PubMed] [CrossRef]
18. Huang X, Zhang X, An N, Zhang M, Ma M, Yang Y, et al. Cryo-EM structure and molecular mechanism of abscisic acid transporter ABCG25. Nat Plants. 2023;9:1709–19. doi:10.1038/s41477-023-01509-7. [Google Scholar] [PubMed] [CrossRef]
19. Jarzyniak KM, Jasiński M. Membrane transporters and drought resistance—a complex issue. Front Plant Sci. 2014;5:687. doi:10.3389/fpls.2014.00687. [Google Scholar] [PubMed] [CrossRef]
20. Ying W, Liao L, Wei H, Gao Y, Liu X, Sun L. Structural basis for abscisic acid efflux mediated by ABCG25 in Arabidopsis thaliana. Nat Comm. 2023;9:1698–708. doi:10.1038/s41477-023-01510-0. [Google Scholar] [PubMed] [CrossRef]
21. Yang Q, Deng X, Liu T, Qian J, Zhang P, Zhu E, et al. Abscisic acid root-to-shoot translocation by transporter AtABCG25 mediates stomatal movements in Arabidopsis. Plant Physiol. 2024;195:671–84. doi:10.1093/plphys/kiae073. [Google Scholar] [PubMed] [CrossRef]
22. Zhou Y, Wang Y, Zhang D, Liang J. Endomembrane-biased dimerization of ABCG16 and ABCG25 transporters determines their substrate selectivity in ABA-regulated plant growth and stress responses. Mol Plant. 2024;17:478–95. doi:10.1016/j.molp.2024.02.005. [Google Scholar] [PubMed] [CrossRef]
23. Kuromori T, Sugimoto E, Shinozaki K. Brachypodium BdABCG25 is a homolog of Arabidopsis AtABCG25 involved in the transport of abscisic acid. FEBS Lett. 2021;595:954–9. doi:10.1002/1873-3468.13925. [Google Scholar] [PubMed] [CrossRef]
24. Pawela A, Banasiak J, Biala W, Martinoia E, Jasinski M. MtABCG20 is an ABA exporter influencing root morphology and seed germination of Medicago truncatula. Plant J. 2019;98:511–23. doi:10.1111/tpj.14234. [Google Scholar] [PubMed] [CrossRef]
25. Matsuda S, Takano S, Sato M, Furukawa K, Nagasawa H, Yoshikawa S, et al. Rice stomatal closure requires guard cell plasma membrane ATP-binding cassette transporter RCN1/OsABCG5. Mol Plant. 2016;9(3):417–27. doi:10.1016/j.molp.2015.12.007. [Google Scholar] [PubMed] [CrossRef]
26. Xin J, Zhou Y, Qiu Y, Geng H, Wang Y, Song Y, et al. Structural insights into AtABCG25, an angiosperm-specific abscisic acid exporter. Plant Comm. 2024;5:100776. doi:10.1016/j.xplc.2023.100776. [Google Scholar] [PubMed] [CrossRef]
27. Zhang Y, Berman A, Shani E. Plant hormone transport and localization: signaling molecules on the move. Annu Rev Plant Biol. 2023;74:453–79. doi:10.1146/arplant.2023.74.issue-1. [Google Scholar] [CrossRef]
28. Xu Y, Qi S, Wang Y, Jia J. Integration of nitrate and abscisic acid signaling in plants. J Exp Bot. 2024;75(11):3259–68. doi:10.1093/jxb/erae128. [Google Scholar] [PubMed] [CrossRef]
29. Couée I. Perspectives in plant abiotic stress signaling. Methods Mol Biol. 2023;2642:429–44. doi:10.1007/978-1-0716-3044-0. [Google Scholar] [CrossRef]
30. Do THT, Martinoia E, Lee Y, Hwang JU. 2021 update on ATP-binding cassette (ABC) transporters: how they meet the needs of plants. Plant Physiol. 2021;187:1876–92. doi:10.1093/plphys/kiab193. [Google Scholar] [PubMed] [CrossRef]
31. Russo G, Capitanio S, Trasoletti M, Morabito C, Krukowski PK, Visentin I, et al. Strigolactones promote the localization of the ABA exporter ABCG25 at the plasma membrane in root epidermal cells of Arabidopsis thaliana. J Exp Bot. 2023;74(18):5881–95. doi:10.1093/jxb/erad298. [Google Scholar] [PubMed] [CrossRef]
32. Zhang Y, Kilambi HV, Liu J, Bar H, Lazary S, Egbaria A, et al. ABA homeostasis and long-distance translocation are redundantly regulated by ABCG ABA importers. Sci Adv. 2021;7:eabf6069. doi:10.1126/sciadv.abf6069. [Google Scholar] [PubMed] [CrossRef]
33. Wang P, Zhao Y, Li Z, Hsu CC, Liu X, Fu L, et al. Reciprocal regulation of the TOR kinase and ABA receptor balances plant growth and stress response. Mol Cell. 2018;69(1):100–112.e6. [Google Scholar] [PubMed]
34. Dourmap C, Roque S, Morin A, Caubrière D, Kerdiles M, Béguin K, et al. Stress signalling dynamics of the mitochondrial electron transport chain and oxidative phosphorylation system in higher plants. Annals Bot. 2020;125:721–36. doi:10.1093/aob/mcz184. [Google Scholar] [PubMed] [CrossRef]
35. Vishwakarma K, Upadhyay N, Kumar N, Yadav G, Singh J, Mishra RK, et al. Abscisic acid signaling and abiotic stress tolerance in plants: a review of current knowledge and future prospects. Front Plant Sci. 2017;8:161. [Google Scholar] [PubMed]
36. Jiang N, Zhu XG. Modern phenomics to empower holistic crop science, agronomy, and breeding research. J Genet Genomics. 2024;51:790–800. doi:10.1016/j.jgg.2024.04.016. [Google Scholar] [PubMed] [CrossRef]
37. Yin W, Xiao Y, Niu M, Meng W, Li L, Zhang X, et al. ARGONAUTE2 enhances grain length and salt tolerance by activating BIG GRAIN3 to modulate cytokinin distribution in rice. Plant Cell. 2020;32:2292–306. doi:10.1105/tpc.19.00542. [Google Scholar] [PubMed] [CrossRef]
Cite This Article
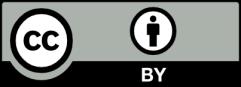
This work is licensed under a Creative Commons Attribution 4.0 International License , which permits unrestricted use, distribution, and reproduction in any medium, provided the original work is properly cited.