Open Access
ARTICLE
Mitigation of Detrimental Effects of Salinity on Sweet Pepper through Biochar-Based Fertilizers Derived from Date Palm Wastes
1 Centre for Scientific and Technical Research on Arid Regions, BP 1682 RP, Biskra, 07000, Algeria
2 Department of Nature and Life Sciences, Faculty of Sciences, Pole Urban Ouzera, University of Medea, Medea, 26000, Algeria
3 Department of Soil and Environmental Sciences, Faculty of Crop Production Sciences, The University of Agriculture, Peshawar, 25130, Pakistan
4 Pharmacy Department, University of Salerno, via Giovanni Paolo II 132, Fisciano (SA), 84084, Italy
5 Department of Silviculture, Forestry and Game Management Research Institute, Na Olivě 550, Opočno, 517 73, Czech Republic
* Corresponding Authors: Adil Mihoub. Email: ; Jakub Černý. Email:
(This article belongs to the Special Issue: Abiotic Stress Tolerance in Crop Plants: Physio-biochemical and Molecular Mechanisms)
Phyton-International Journal of Experimental Botany 2024, 93(11), 2993-3011. https://doi.org/10.32604/phyton.2024.057536
Received 20 August 2024; Accepted 21 October 2024; Issue published 30 November 2024
Abstract
Globally, salinity is a brutal environmental constraint that poses a major threat to agriculture worldwide, causing nutrient imbalances and oxidative stress, leading to reduced crop yields and quality. Date palm waste from the agro-industry is a major environmental problem, but its conversion to biochar for soil amendment could help alleviate the effects of salinity stress. Pepper is a commonly grown horticultural crop that is sensitive to salinity. That’s why the current experiment was conducted with the novel idea of exploring the potential use of biochar-based fertilizer derived from date palm waste as a mitigation strategy for salinity-related problems in pepper. The study was conducted as a pot experiment in a growth chamber under controlled conditions. The experiment consisted of four treatment groups: Control (no salinity stress); BM350E (biochar application only); NaCl (salinity stress without biochar application); BM350E+NaCl (combined biochar and salinity stress application). The soil was amended with biochar at a concentration of 3 g kg−1 soil and pepper seedlings were exposed to salinity stress with 150 mM NaCl for seven days. The morphological, biochemical, and physiological responses were then evaluated. The use of BM350E biochar significantly improved plant growth under saline conditions, increasing shoot fresh weight by 9.41%, root fresh weight by 15.32%, shoot length by 5.22%, and root length by 12.57%. It also increased chlorophyll a (Chl. a) by 8.28%, chlorophyll b (Chl. b) by 80.20%, and carotenoids (Car) by 52.43% while increasing antioxidant enzyme activities. In conclusion, BM350E biochar has the potential to effectively mitigate the negative effects of salinity on pepper growth.Keywords
Implementing sustainable agriculture in arid and semi-arid regions is facing multiple and complex challenges, including the declined soil fertility and soil salinization [1]. Mineral fertilization is one of the practices commonly used by small-scale farmers to improve crop yields on poor soils [2]. However, the high cost of fertilizers and their limited availability in production areas limit their access to the quantities needed to effectively improve crop productivity [3,4]. Furthermore, the excessive and continues use of chemical fertilizers certainly improves agricultural production but leads to serious environmental complications [5].
Soil salinity, ranked as the second most important factor in soil degradation following erosion, has consistently posed a major challenge for agriculture [6]. Currently, more than 1 billion hectares of irrigated land are considered to be salt residuals, and current irrigation and global climate change are expected to increase [7].
Sodium chloride (NaCl) poses significant challenges in soil salinity management, leading to stunted growth and reduced crop yields. High concentrations of Na+ cause significant damage to the cytosol of leaf cells. This damage occurs because Na+ interferes with various metabolic activities, including photosynthesis [8]. Salt stress induces the production of cytotoxically activated oxygen. This oxidative stress leads to damage in lipids, proteins, and nucleic acids [9]. Furthermore, salinity can generate reactive oxygen species (ROS), including hydroxyl radicals (OH) and superoxide radicals (O2−) [10].
Peppers, specifically Capsicum annuum L., are among the three most widely cultivated vegetable crops globally, alongside potatoes and tomatoes. It belongs to the family Solanaceae and the genus Capsicum [11]. However, several factors continue to have significant detrimental effects on peppers, reducing productivity and fruit quality. The most important stress is biotic, although peppers are also susceptible to abiotic stresses such as salinity [12].
Soil can be considered saline when its electric conductivity reaches 4 dS m−1 (~40 mM NaCl) [13]. Pepper has been reported to be moderately sensitive to salt, with a salinity threshold of 2 dS m−1. The yield decreases significantly when it reaches 4 dS m−1 [14]. Thus, salinity causes around 14% of yield loss [10]. Plants can combat salt stress by producing secondary metabolites, such as flavonoids and phenolic acids, which serve as key defensive mechanisms to minimize damage [15]. Numerous studies indicate that the response of plants to salt stress and its impact can vary significantly depending on the species and varieties [16–19]. Pepper has been a key field and greenhouse crop in the Mediterranean for centuries, making it economically profitable. It is a top vegetable grown in plastic houses in Biskra (Sothern Algeria) for export and local consumption. Greenhouse farming systems demand greater fertilizer input than field cultivation. However, the salt from these fertilizers resulted in the salinization of the cultivated area after harvesting crops. It is therefore necessary to implement immediate actions to reduce the impact of salinity, protect agricultural land, and sustainably improve crop production [20].
In the Algerian oases, huge quantities of date palm residues are generated every year (around 1 million tons every year) [21]. This residue, generated annually by various maintenance activities, has not attracted enough attention for its recovery [22]. The recovery and recycling of date palm waste could be considered an important economic activity and a real source of energy [23]. Moreover, the agricultural valorization of palm tree residues could provide a sustainable method for recycling waste in the framework of a circular economy [24].
Biochar, a by-product of biomass pyrolysis, could potentially improve soil organic status and carbon sequestration and optimize the mineral fertilization needed to support agricultural production in poor soils [25]. Biochar is a product of organic material pyrolysis. It can offer some highly promising solutions for mitigating soil salinity in agriculture [6]. It has a high cation exchange capacity, which may satisfactorily immobilize salt ions, hence reducing soil salinity [20]. The porous structure of biochar makes it very effective in improving water retention, hence reducing fast evaporation in saline soils. Biochar also fosters the activity of microbes, which seem to fix nutrients and, therefore, increase the fertility of the soil [26].
For improvement in the properties, many methods with respect to physical, chemical, and thermal modification have been applied to biochar by scientists until now [25]. Chemically modified biochars are often used only for the purpose of environmental remediation. To the best of our knowledge, there is not a single research in which the effect of date palm residues-derived biochar on mitigating salinity stress in vegetables has been reviewed, particularly with reference to its chemical modification. In this study, we assume that the newly produced biochar can be a good slow-release fertilizer to improve poor soils and, more importantly, mitigate the damage from salt stress. The overall aim of this research is to contribute to sustainable soil management by applying biochar-based fertilizers made from locally available and little-used wastes (lignocellulosic by-products of the date palm). Specifically, this study aims to examine the potential of biochar-based fertilizers produced from date palm waste as a solution for overcoming salt-induced challenges in growing salt-sensitive crops like sweet peppers under saline environments.
2.1 Collecting Feedstock, Biochar Preparation and Modification
Raw date palm agro-waste biomass material was gathered from date palm orchards located in Southern Algeria, specifically in El Outaya, Biskra (36°55′36.6″ N; 05°38′56″ E). The collected material undergoes a crushing process. The crushed material is washed with mineralized water and left to dry for 24 h under the sun’s heat before undergoing pyrolysis under aerobic conditions at a temperature of 350°C. The pyrolysis duration was four hours with a heating rate of ~6.5°C min−1 (Fig. 1). After the pyrolysis process, the material was allowed to cool, then crushed and grounded (≤1 mm). The yield of biochar was obtained by dividing the mass of the biochar on the initial mass of the raw materials subjected to pyrolysis [27].
Figure 1: Schematic illustration of the date palm biochar preparation and modification
The biochar was impregnated in an acidic system (5 M HCl–1:10 w/v) for 7 days and then exposed to ambient air conditions. The impregnation process was done to better enhance the porous structure of the material in mesopores and increase its specific surface area. This process also introduces oxygen-containing functional groups, such as carboxyl, phenolic, and hydroxyl groups, onto the surface of the biochar. Afterward, the biochar is washed with demineralized water using a fine mesh filter, after which it is spread out to air dry. After the impregnation period, a second impregnation step was conducted to enhance the biochar even further. This nutrient solution, impregnated into the biochar, was prepared by dissolving 50 g of Monoammonium phosphate MAP and 6 g of Potassium sulfate K2SO4 in 1000 mL of distilled water. After allowing the biochar to stir well in the solution for the greatest possible intake of the nutrients, it was carefully filtered through a fine mesh-sized sieve. Afterward, it was spread on a paper sheet and dried in the oven at 65°C for 48 h.
2.2 Characterization of Biochar Samples
The Biochar produced at 350°C was modified, and subsequently, a series of physicochemical and mineralogical analyses were carried out to understand its final quality. The physicochemical characterization was conducted as per the standard ASTM D1762-84 [28]. The surface morphology of the biochar sample obtained at 350°C was carried out by using a Prisma E SEM scanning electron microscope (Thermo Scientific, Waltham, MA, USA) at an accelerating voltage of 15.00 kV. X-Ray diffraction (XRD) spectroscopy was completed using D8 Advance diffractometer (Bruker AXS, Karlsruhe, Germany) operating at the tube voltage of 40 kV and tube current of 40 mA. The results of the biochar analysis are shown in Table 1.
Because biochar is typically alkaline and several studies have shown that adding biochar to agricultural soils can increase soil pH. As a result, we endeavored to develop a method that would make our Biochar useful in its properties without raising the alkaline soil pH. It is clear from the results that the addition of acid after pyrolysis significantly reduces the pH of the Biochar, which can be beneficial for use in soils with an alkaline pH. Acid modification after pyrolysis enhances cation exchange capacity (CEC). Chemical treatment doubles CEC values. The addition of acid after pyrolysis increased the EC of the Biochar. The biochars were subjected to scanning electron microscopy (SEM) at 10 µm magnifications.
Fig. 2 shows SEM images of unmodified and modified biochar. The images show that both types of Biochar have many small pores, making them porous. But after the modification of the Biochar, there was even more pore volume, which means that the acidification of the Biochar further develops the porous structure of the Biochar, which of course, increases its specific surface area. This helps retain water and nutrients near roots for plant utilization. Biochar also fosters the growth of beneficial bacteria, promoting nutrient uptake by plants.
Figure 2: Scanning electron microscope (SEM) images of unmodified (B350) and modified date palm biochars pyrolyzed at 350°C (BM350E)
X-ray diffraction analysis (XRD) was carried out to identify the presence of different crystalline minerals that have an impact on the properties of Biochar, influencing its various applications. Fig. 3 illustrates the changes in the crystalline structure of date palm biochar following various treatments. The Biochar modification process resulted in a decrease in the intensity of several peaks on the XRD plot. This decrease can be attributed to the acidification of the Biochar, which led to the deterioration of the crystalline structure of certain components such as sylvite (KCl), calcite (CaCO3) and halite (NaCl). However, in the unmodified biochar samples (B350), the peaks were more intense, probably due to the higher concentrations of calcium (Ca), potassium (K) and chlorine (Cl) compared with the treated biochars.
Figure 3: X-ray diffraction analysis spectra of unmodified (B350) and modified date palm biochars pyrolyzed at 350°C (BM350E)
2.3 Experimental Design and Plant Growing Conditions
The current investigation was conducted in a growth chamber with regulated conditions, maintaining temperature of 25°C ± 1°C, approximately 60% relative humidity, and a 16 h photoperiod, at the Faculty of Science laboratory at Yahia Fares University in Medea, Algeria. The plant test used in this research is ADINA, a variety of sweet pepper (Capsicum) from the Birtouta region (Algiers, Algeria). This variety was selected for its superior growth capacity and sensitivity to salinity. The pepper seedlings were transferred to the laboratory at the University of YAHIA Fares in Medea prior to pot experiment. The experiment was conducted using 6.5 cm diameter, 10 cm tall plastic pots filled with acid-washed sand (100 g per pot). Seedlings were transplanted into the pots (one seedling per pot), and the acid-washed sand served as the growing medium. The acid-washing of sand dissolves salts and calcareous particles, aiding in leaching and reducing soluble salt concentration. Modified biochar was applied in powdered form at a mass ratio of 3% (equivalent to 30 g per 1 kg of soil) and uniformly mixed with the soil before planting the pepper. Seedlings were watered with a nitrogen (N)-free nutrient solution containing: KH2PO4 (250 µmol), MgSO4 (1000 µmol), K2SO4 (750 µmol), CaCl2 (1650 µmol), Fe-EDTA (16 µmol), MnSO4 (6 µmol), H3BO3 (4 µmol), ZnSO4 (1 µmol), NaMoO4 (0.1 µmol), and CuSO4 (1 µmol). Subsequently, plants were watered with two levels of salinity: 0 mM and 150 mM. To achieve these salinity levels, NaCl was added to the irrigation water in conjunction with a nitrogen-free nutrient solution. The four treatment groups were labeled as follows: CK (Control without salinity stress); BM350E without salinity stress; NaCl (Control with salinity stress); BM350E + NaCl (BM350E, 3% with salinity stress). To ensure accuracy, the experiment was laid out in a Randomized Complete Block Design (RCBD) with the treatments replicated three (3) times. After one week of stress, parameters related to growth, photosynthesis, and oxidative stress metabolism were assessed.
All morphological parameters were assessed at the time of cutting, i.e., 7 days after planting.
The shoot and root length of the selected seedlings were first measured with a ruler. The fresh weight of the shoot and root was measured using an analytical balance.
2.4.2 Pigments and Photosynthetic Attributes
Chlorophyll and carotenoid levels were evaluated following the procedures of Arnon [29] and Davis [30], respectively. After determining the absorbance of the supernatants on a spectrophotometer (Hitachi U-2800, Tokyo, Japan), the concentrations of chlorophyll a and b were determined by measuring the absorbance at 663 nm and 645 nm, respectively. Carotenoid content was determined by recording the absorbance at 470 nm, with the findings expressed in mg g−1 per gram of fresh weight (FW).
2.4.3 Oxidative Stress Biomarkers
According to Velikova et al. [31], the amount of hydrogen peroxide (H2O2) was determined following the reaction with potassium iodide (KI). The reaction mixture was kept in the dark for 1 h. Using a standard curve generated with H2O2 solutions, the amount of H2O2 was determined spectrophotometrically (Ultraviolet-2600, Shimadzu, Japan) at 390 nm. The total protein content (TPC) of the leaves was determined using the method outlined by Ouzounidou et al. [32] was used, utilizing 100 mg of leaf tissue for the analysis. The level of lipid peroxidation was measured by the content of malondialdehyde using thiobarbituric acid according to the method described by Gupta et al. [16]. Briefly, 1 mL of the extract was mixed with a tube containing 20% (v/v) trichloroacetic acid and 0.5% (v/v) thiobarbituric acid. After cooling to room temperature, the mixture was heated in a water bath for 30 min at 95°C and centrifuged for 10 min at 10,000 g. Thiobarbituric acid reactive substances were measured at 530 and 600 nm absorbance, with data given here in µmol g−1 FW.
2.4.4 Total Polyphenol and Flavonoids Contents
The total polyphenol content of the plant samples was determined using the Folin-Ciocalteu assay. The technique is based on the reduction of the Folin-Ciocalteu reagent in an alkaline medium, generating blue-colored reduction products. Results were determined at 765 nm and expressed in milligrams of gallic acid equivalents per g−1 FW. Total flavonoids in the plant extracts were estimated with the aluminum chloride colorimetric assay. Mixed 1 mL of the extract with 1 mL AlCl3 solution, incubated for 10 minutes, and then read the absorbance at 430 nm. The TFC of the extracts is then expressed as mg of quercetin equivalents (QE) per gram of fresh weight (mg EQ g−1 FW).
2.4.5 Antioxidant and Detoxification Enzyme Activities
Shoot tissue samples were homogenized in an ice-cold extraction buffer comprising potassium phosphate buffer, EDTA, and polyvinylpyrrolidone. Ascorbic acid was added to extract ascorbate peroxidase. After centrifugation, supernatants were used for enzyme activity assay. The activities of the enzymes ascorbate peroxidase (APX), catalase (CAT), peroxidase (POD), glutathione S-transferase (GST) were assayed according to protocols reported by Nakano et al. [33], Aebi [34], Cakmak et al. [35], Habig et al. [36], respectively.
Statistical analysis was conducted with Graphpad Prism v8 software. The data are expressed as mean ± standard deviation (SD). To determine significant differences among treatments, a one-way analysis of variance (ANOVA) was conducted, followed by Duncan’s multiple range test, with the significance level established at p < 0.05. To visualize the data, principal Component Analysis (PCA), hierarchical cluster plots, and calculate Pearson correlations, the Origin software was used (OriginLab, 2021).
3.1 Effect of Biochar on Pepper Growth under Salt Stress Conditions
Pepper seedlings exposed to saline soil alone and/or with biochar for seven days showed dramatic effects, including a reduction in root and shoot length. On the other hand, the biochar amendment suppressed the alterations and phytotoxicity induced by NaCl and improved the growth of seedlings subjected to salt stress (Fig. 4) The biochar amendment increased the fresh weight of shoots by 3.15% (Fig. 5a), the fresh weight of roots by 16.71% (Fig. 5b), the length of shoots by 16.37% (Fig. 5c) and the length of roots by 8.28% (Fig. 5d) in the absence of salt stress compared with CK seedlings. Salt stress had a negative effect on shoot fresh weight 31.45% and root fresh weight 42.73% and shoot length 38.89% and root length 25.72% compared to CK seedlings. In the presence of saline stress, biochar amendment mitigated salinity-induced damage and improved shoot fresh weight 9.41%, root fresh weight 15.32%, shoot length 5.22% and root length 12.57% (Fig. 5).
Figure 4: Morphological response of pepper to biochar under induced (150 mM) salinity stress. CK (no salinity stress); BM350E (biochar application only); NaCl (salinity stress without biochar application); BM350E+NaCl (combined biochar and salinity stress application)
Figure 5: (A) shoot fresh weight (SFW), (B) root fresh weight (RFW), (C) shoot length (SL), and (D) root length (RL) in treated pepper plants (Capsicum annum L. var. ADINA) with or without modified biochar, both under salt stress and non-stress conditions. CK (no salinity stress); BM350E (biochar application only); NaCl (salinity stress without biochar application); BM350E+NaCl (combined biochar and salinity stress application). Soil was amended with 3 g kg−1 biochar; pepper seedlings were exposed to 150 mM NaCl salinity stress for 7 days. Bars followed by the same letters show no statistical difference at p < 0.05 (Duncan’s multiple range test). Values are given as bars showing mean ± standard error for three determinations
3.2 Effect of Biochar on Biochemical Parameters of Pepper under Salt Stress Conditions
Under salt stress, a decline of 37.45%, 33.56% and 52.09% was observed in chlorophyll a (Chl. a), chlorophyll b (Chl. b) and carotenoids (CAR) content, respectively, compared to the control, which was expected. The addition of BC resulted in an increase of 8.28% in Chl. a (Fig. 6a), 80.20% in Chl. b (Fig. 6b), and 52.43% in CAR (Fig. 6c). On the other hand, the incorporation of BC reduced the degradation of photosynthetic pigments, increasing their concentration.
Figure 6: (A) chlorophyll a, (B) chlorophyll b, and (C) carotenoids in treated pepper plants (Capsicum annum L. var. ADINA) with or without modified biochar, both under salt stress and non-stress conditions. CK (no salinity stress); BM350E (biochar application only); NaCl (salinity stress without biochar application); BM350E+NaCl (combined biochar and salinity stress application). Soil was amended with 3 g kg−1 biochar; pepper seedlings were exposed to 150 mM NaCl salinity stress for 7 days. Bars followed by the same letters show no statistical difference at p < 0.05 (Duncan’s multiple range test). Values are given as bars showing mean ± standard error for three determinations
3.3 Effect of Biochar on Oxidative Stress Biomarkers under Salt Stress Conditions
Salt stress induced significant oxidative stress in pepper seedlings, as evidenced by MDA content and H2O2 production, both of which were highly reactive and toxic, and induced considerable structural damage. NaCl treatment increased H2O2 and MDA content by 50.47% (Fig. 7a), and 54.38% (Fig. 7b), respectively in the shoots compared with CK. Biochar amendment effectively reduced H2O2 and MDA concentrations by 44.17% and 31.19% in shoots under salt stress compared with pepper seedlings subjected to salt stress without biochar amendment.
Figure 7: (A) hydrogen peroxide (H2O2) production and (B) malondialdehyde (MDA) content in treated pepper plants (Capsicum annum L. var. ADINA) with or without modified biochar, both under salt stress and non-stress conditions. CK (no salinity stress); BM350E (biochar application only); NaCl (salinity stress without biochar application); BM350E+NaCl (combined biochar and salinity stress application). Soil was amended with 3 g kg−1 biochar; pepper seedlings were exposed to 150 mM NaCl salinity stress for 7 days. Bars followed by the same letters show no statistical difference at p < 0.05 (Duncan’s multiple range test). Values are given as bars showing mean ± standard error for three determinations
3.4 Effect of Biochar on Antioxidant Enzymes of Pepper under Salt Stress Conditions
Antioxidant enzyme activity was spectrophotometrically assessed in the shoots of BC-treated pepper seedlings to explore the possible effects of Biochar on the antioxidant mechanism in NaCl-stressed pepper seedlings. Exposure to NaCl significantly induced antioxidant enzyme activity in shoots, resulting in a 20.68% and 10.36% increase in CAT and APX activities, respectively (Fig. 8a,b), compared to CK. In the shoots of NaCl-treated seedlings, biochar application significantly enhanced CAT, APX, GST, and POD activity by 49.1% (Fig. 8a), 0.37% (Fig. 8b), 15.88% (Fig. 8c), and 34.09% (Fig. 8d), compared with those seedlings that were under salt stress without application.
Figure 8: (A) catalase (CAT), (B) ascorbate peroxidase (APX), (C) glutathione-S-transferase (GST), and (D) peroxidase (POD) activities of treated pepper plants (Capsicum annum L. var. ADINA) with or without modified biochar, both under salt stress and non-stress conditions CK (no salinity stress); BM350E (biochar application only); NaCl (salinity stress without biochar application); BM350E+NaCl (combined biochar and salinity stress application). Soil was amended with 3 g kg−1 biochar; pepper seedlings were exposed to 150 mM NaCl salinity stress for 7 days. Bars followed by the same letters show no statistical difference at p < 0.05 (Duncan’s multiple range test). Values are given as bars showing mean ± standard error for three determinations
3.5 Effect of Biochar on Secondary Metabolites of Pepper under Salt Stress Conditions
Exposure of pepper seedlings to NaCl and/or BC for 7 days resulted in increased accumulation of total polyphenols. Applying NaCl and BC separately resulted in a 75.31% and 20.80% increase in total polyphenols in the shoots, which was higher compared to the control group. However, the combined application of NaCl and BC resulted in significantly reduced, i.e., (16.5%) polyphenols synthesis in shoots compared with control treatments (Fig. 9a). Individual application of NaCl and Biochar resulted in flavonoid levels of 48.2% and 19.02%, respectively which were comparatively higher than those measured in control seedling shoots (Fig. 9b). However, the combined application of NaCl and biochar resulted in significantly higher, i.e., polyphenols and flavonoid synthesis in plants compared to control seedlings.
Figure 9: (A) polyphenols (PPO) and (B) flavonoid (FLAV) levels in treated pepper plants (Capsicum annum L. var. ADINA) with or without modified biochar, both under salt stress and non-stress conditions. CK (no salinity stress); BM350E (biochar application only); NaCl (salinity stress without biochar application); BM350E+NaCl (combined biochar and salinity stress application). Soil was amended with 3 g kg−1 biochar; pepper seedlings were exposed to 150 mM NaCl salinity stress for 7 days. Bars followed by the same letters show no statistical difference at p < 0.05 (Duncan’s multiple range test). Values are given as bars showing mean ± standard error for three determinations
3.6 Principal Component Analysis and Hierarchical Cluster Analysis
Principal Component Analysis (PCA) is employed to assess the effects of biochar and salinity stress on various morpho-physiological traits and antioxidant enzymes. PCA is applied to 15 traits across 04 treatments, including the control. The results reveal that salinity considerably affects multiple morpho-physio-biochemical traits in every treatment. The first two components (PCs) explain 93% of the total variation, and the PCA-based biplot using these components illustrated associations between morphological traits (PH, RL, SDW, RFW, Chl. a, Chl. b, and CAR) and treatments BM350E (Fig. 10a). This reflects the positive effects of BM350E on pepper growth and related parameters. The compounds POD, GST, CAT, APX, H2O2, MDA, FLAV, and PPO have been observed to be more associated with the physiological responses observed in plants subjected to BM350E+NaCl and NaCl treatments. These responses highlight the complex interplay between salinity stress and plant defense mechanisms. Conversely, control treatments show negative relationships with all measured traits. The first notable cluster merges Relative Fresh Weight (RFW) and Chlorophyll a (Chl. a) with a similarity of 21.08. Similarly, Root Length and Shoot Fresh Weight (SFW) form a cluster at a similarity level of 27.76. Carotenoids (CAR) are grouped with a previous cluster at a similarity level of 31.02. Catalase (CAT) and Glutathione S-transferase (GST) exhibit a similarity of 40.99, suggesting their related antioxidant functions. Plant Height and Chlorophyll b cluster together with a similarity of 42.22, indicating possible associations between growth and photosynthetic pigments. Hydrogen peroxide (H2O2) and Polyphenol Oxidase (PPO) are clustered at a similarity of 44.44, reflecting their roles in oxidative stress responses. Other variables, such as Malondialdehyde (MDA) and their associated cluster merge at a higher similarity threshold of 52.33. Ascorbate Peroxidase (APX) and Flavonoids (FLAV) form another group with a similarity of 62.42, indicative of their role in the antioxidant defense system. Finally, Peroxidase (POD) shows a similarity of 63.43 with other antioxidant-related variables, highlighting the tight association of these enzymes in the plant stress response (Fig. 10b).
Figure 10: Cluster plot with convex hull for treatments (A) and hierarchical cluster plot for studied attributes (B). CK (no salinity stress); BM350E (biochar application only); NaCl (salinity stress without biochar application); BM350E+NaCl (combined biochar and salinity stress application). Soil was amended with 3 g kg−1 biochar; pepper seedlings were exposed to 150 mM NaCl salinity stress for 7 days. SFW (shoot fresh weight); RFW (root fresh weight); Chl. a (chlorophyll a); Chl. b (chlorophyll b); Car (carotenoids); MDA (malondialdehyde); H2O2 (hydrogen peroxide); PPO (polyphenols); FLAV (flavonoids); POD (peroxidase); CAT (catalase); GST (glutathione-S-transferase); APX (ascorbate peroxidase)
3.7 Pearson Correlation Analysis
Plant height shows moderate positive correlations with relative fresh weight (RFW) (r = 0.515), chlorophyll b (Chl. b) (r = 0.493), and root length (r = 0.340), indicating that taller plants tend to have greater biomass and higher chlorophyll content. Root length also has a strong positive correlation with shoot fresh weight (SFW) (r = 0.667) and RFW (r = 0.551), suggesting that root development supports overall biomass production. Chlorophyll a (Chl. a) is strongly correlated with RFW (r = 0.747), SFW (r = 0.638), and root length (r = 0.550), implying its role in promoting plant growth and biomass accumulation. Similarly, carotenoids (Car) show positive associations with SFW (r = 0.649) and RFW (r = 0.691), reflecting their role in photosynthesis and stress tolerance. Interestingly, malondialdehyde (MDA), an indicator of oxidative stress, shows weak correlations with most growth-related parameters, but a moderate positive correlation with Chl. b (r = 0.447), hinting at its involvement in plant stress responses. Hydrogen peroxide (H2O2), another stress marker, shows no strong correlations with other variables, indicating that its accumulation may not be directly linked to growth traits. Antioxidant enzymes exhibit negative correlations with growth-related parameters. Catalase (CAT) is negatively correlated with root length (r = −0.566), SFW (r = −0.332), and RFW (r = −0.471), suggesting that higher antioxidant activity may be linked to stress rather than growth. Ascorbate peroxidase (APX) also shows strong negative correlations with RFW (r = −0.586), Chl. a (r = −0.433), and Chl. b (r = −0.554), further supporting this trend. Peroxidase (POD), on the other hand, has a positive correlation with MDA (r = 0.354) and hydrogen peroxide (H2O2) (r = 0.399), indicating its role in the plant’s oxidative stress response. Glutathione S-transferase (GST) has negative correlations with root length (r = −0.581), SFW (r = −0.589), and CAT (r = −0.508), while polyphenol oxidase (PPO) displays a strong negative correlation with SFW (r = −0.698), reflecting the inverse relationship between oxidative stress responses and growth. Finally, flavonoids (FLAV) show moderate negative correlations with RFW (r = −0.314) and carotenoids (r = −0.525), suggesting a potential balance between secondary metabolites and growth traits in plant stress adaptation (Fig. 11).
Figure 11: Pearson correlation chart of measured parameters. CK (no salinity stress); BM350E (biochar application only); NaCl (salinity stress without biochar application); BM350E+NaCl (combined biochar and salinity stress application). Soil was amended with 3 g kg−1 biochar; pepper seedlings were exposed to 150 mM NaCl salinity stress for 7 days. PH (plant height); RL (root length); SFW (shoot fresh weight); RFW (root fresh weight); Chl. a (chlorophyll a); Chl. b (chlorophyll b); Car (carotenoids); MDA (malondialdehyde); H2O2 (hydrogen peroxide); PPO (polyphenols); FLAV (flavonoids); POD (peroxidase); CAT (catalase); GST (glutathione-S-transferase); APX (ascorbate peroxidase)
Advanced technologies can be used to combat salinity-induced soil degradation and alleviate its negative effects on the growth of plants and soil quality [37]. Biochar has gained widespread attention owing to its great potential for improving soil fertility and quality and for the role it has played in the immobilization of, and increase in bioavailability of, toxic elements [38].
As compared to the seedlings of the control group, pepper seedlings that experienced salt stress had hugely reduced growth and fresh weight. Based on this study, the toxicity of NaCl had a significant impact on the growth of peppers, resulting in decreased biomass yield for both roots and shoots. Salt stress is becoming the primary abiotic stress factor limiting crop productivity. Indeed, this reduction in growth may stem from salt’s impact on metabolic processes and molecular changes. Salt can affect water availability to plants by hindering water uptake through the roots and potentially exerting toxicity, thereby impacting plant growth. Salt stress significantly affects plant growth and biomass accumulation, primarily through the induction of oxidative stress [39]. This stress is characterized by the increased production of reactive oxygen species (ROS), notably hydrogen peroxide (H2O2) and malondialdehyde (MDA), which are indicative of cellular damage [40]. It also considerably reduces growth and yield by lowering chlorophyll levels, membrane stability and photosynthetic activity. Numerous studies have reported the toxic effects of salts on crop growth [41–43]. Their findings indicated that salt stress adversely affects cell division. Moreover, salt stress in soil has an effect on the growth of a plant for three reasons. In the first case, the quantity of salt available in the soil solution results in decreases in the potential of the plant to obtain water, consequently leading to a decrease in the growth rate of the plant. This can be linked or attributed to the osmotic effect of the salt stress. Secondly, elevated levels of Na+ ions in the soil solution increase osmotic pressure, which inhibits the uptake of essential nutrients, particularly nitrogen (N), phosphorus (P), and potassium (K), affecting their availability to plants. Thirdly, the excess salt that enters the plant’s transpiration system can disrupt leaf transpiration, potentially stunting growth. Growth and development are essential processes for the continued survival and multiplication of any plant species. Based on the findings of this study, NaCl toxicity severely affected pepper growth in terms of loss of biomass yield (roots and shoots), whereas the addition of Biochar significantly improved seedling growth (Fig. 5). Our findings results align with those reported by Semida et al. [44] revealed that biochar application at high rates can reduce the harmful impact of salt stress, which decreases pepper plant growth. Furthermore, chemical modification of biochar has led to its widespread use to improve agricultural and environmental remediation strategies [25,45]. Such biochar modifications/acidification provide more substantial benefits in both physical characteristics (e.g., surface area, pore structure, molecular weight, cation exchange capacity (CEC)) and chemical properties (introduction of specific functional groups) [45]. The pores in biochar enhance the physical properties of salt-affected soils, hence promoting plant growth under salinity stress [46]. Presence of numerous functional groups within Biochar makes it an appropriate choice for adsorption of different salts that are present within the soils, hence mitigating the salinity of soil [47]. In salt-affected soils, the biochar releases K+, Ca2+, Mg2+, and other elements into the soil for crop growth and reduces Na+ uptake or excludes Na+ from the root cells [48], because of this, nutrient absorption, carbon assimilation, and antioxidant responses may improve, resulting in better plant growth promotion [49].
Photosynthesis is a basic, primary biochemical mechanism supporting life in plants and the carrying out of various activities related to organic compounds synthesized from light energy. Chlorophyll, the pigment allowing plants to gain energy from light, is essential for photosynthesis [18]. Therefore, this photosynthetic efficiency determined the carbohydrate and protein content of plant tissues, mostly under stressful conditions [50]. These data clearly show that NaCl has a negative effect on the rate of photosynthesis and chlorophyll pigment contents, which results in reduced plant growth and biomass. The salt-stress-induced decline in growth may be a result of senescence and reduced photosynthetic activity. It may further be aggravated by a decrease in chlorophyll content [51]. Our findings are corroborated with Dubey [52], who reported that salinity reduced gas exchange, stomatal conductance, and electron transport chain, which eventually creates a disturbance in the synthesis of chlorophyll in plants. This reduction is caused by degradation in the structure of chlorophyll because of the generation of reactive oxygen species and the replacement of Cl- and Na+ ions by essential elements. Pigment content was lowered in NaCl-stressed plants, probably due to the enhanced production of chlorophyll degrading enzymes like chlorophyllase [53]. The photosynthetic activities of the pepper seedlings were improved with the addition of biochar during this research work, probably due to a decrease in Na+ content, followed by an increase in chloroplast ultrastructure. Helaoui et al. [54] demonstrated that biochar supplementation to plants under salt stress reduces sodium accumulation. The reason could be due to enhanced CO2 uptake since a higher chlorophyll content was observed in pepper shoots with enhanced salinity in combination with biochar addition [55].
Moreover, it has been identified that salinity induces the overproduction of H2O2 and MDA content [56]. As a result, many biomarkers have been fixed in recent times past to evaluate the responses of plants against abiotic stress [18]. In contrast, the content of MDA in pepper shoots was reduced under combined treatment with high doses of biochar and salinity compared to without biochar. In agreement with our results, several researchers have reported that salinity stress enhances MDA production in Zea mays L. and Triticum aestivum L. compared to their respective control seedlings. However, the addition of biochar reduces the MDA content compared with seedlings without biochar. Numerous studies have demonstrated that biochar reduces the levels of H2O2 and MDA, thereby enhancing membrane stability [57].
Plants have a ROS-scavenging system that defends them from oxidative damage [58]. Biochar might induce oxidative stress by antioxidant enzymes and also improve salt tolerance in plants. The impact of salt stress on the oxidative status of pepper seedlings was evaluated by analyzing GST, CAT, and MDA content. Salt stress significantly increased enzyme activities compared with the control, with similar results for Zea mays L. and Oryza sativa L. under salt stress. Biochar enhances plant tolerance to salt stress by augmenting antioxidant enzymes and regulating enzyme activities. Indeed, the supplementation of biochar plays a crucial role in regulating enzyme activities and metabolic processes, consequently enhancing plant tolerance to salt stress [49,54].
Total polyphenols and flavonoids express antioxidant properties by: Direct scavenging of reactive oxygen species (ROS), Suppression of ROS formation by inhibition of certain enzymes or by chelation of the metal ions involved in their production, Protection of the body’s antioxidant defence systems [59]. Polyphenols have phenolic hydroxyl groups in their structures, and their antioxidant properties are attributed in part to the ability of these natural compounds to trap free radicals [60]. In this study, pepper seedlings treated with NaCl and/or BC showed a significant improvement in terms of total polyphenols and flavonoids. Biochar amendment significantly improved the phenolic composition and antioxidant capacity of pepper plants. Similar studies reported that seedlings of pepper treated with biochar have produced more total polyphenols and flavonoids than unamended plants [61,62]. Thus, it is believed that the existence of such an improved phenolic content in the biochar-amended plants is accounted for by improved nutrient availability and reduced stress levels in the plants [62].
The enhanced biomass in peppers was increased by the modified biochar, improving its growth. Chlorophyll content was reduced in salt-stressed pepper seedlings. In addition, the induction of salt-induced oxidative stress was alleviated due to the enhanced activities of ascorbic peroxidase, catalase, and glutathione-S-transferase under treatment by BC. Biochar increased chlorophyll and flavonoid content in salt-treated seedlings. In summary, the use of BM350E derived from date palm waste was found to be effective in mitigating the harmful effects of salinity on crops, supporting our hypothesis that this new product can improve plant resistance to saline conditions, thus being particularly important for regions facing salinization challenges. We believe that this work presents significant contributions to the fields of environmental science and sustainable agriculture; however, more investigations at the field level, is suggested for confirmation of 3% BM350E as a high-quality amendment for optimization of growth and yield in different cultivated horticultural crops under salinity conditions. This research not only promotes environmental sustainability but also provides a pathway for improving agricultural productivity in saline soils, particularly in regions where date palm waste is abundantly available.
5.1 Future Research Directions
Future research should focus on optimizing the pyrolysis process duration and exploring the use of various agricultural wastes from Oasis regions, including date palm residues. To address soil degradation, researchers must prioritize the usage of biochar made out of pyrolysis of date palm wastes in arid regions. Additional research endeavours should consider the long-term sustainability and environmental impact of date palm biochar on the microbial community in the soil, especially in the plant rhizosphere, its adaptability to specific crop varieties, and its performance below various abiotic conditions. Furthermore, exploring eco-friendly alternatives to fertilization can contribute to resource efficiency and environmental conservation in arid and semiarid regions. By taking these steps in arid and semiarid regions, we can not only bolster food security but also encourage industries to create valuable products from date palm waste for future use.
Acknowledgement: The authors thank the staff from the laboratory of the Faculty of Science at the University Yahia Fares in Medea (Algeria) for assistance in executing the experiment and carrying out the plant analyses. Adil Mihoub is thankful to the Centre for Scientific and Technical Research on Arid Regions (CRSTRA of Algeria) for facilities during the experiment. This work is a part of the national research project PNR entitled « Développement et diffusion des solutions durables de gestion des sols et de l’eau dans les régions arides », so the General Directorate for Scientific Research and Technological Development (DGRSDT of Algeria) is gratefully acknowledged for the providing the bench facilities for this research programs.
Funding Statement: This research was financially supported by the National Agency of Agricultural Research of the Czech Republic (Project No. QK22020008), the Technological Agency of the Czech Republic (Project No. TQ03000234), and the Ministry of Agriculture (CR), institutional support MZE-RO0123.
Author Contributions: Conceptualization, Adil Mihoub, Mohammed Mesnoua, and Nabil Touzout; methodology, Adil Mihoub, and Nabil Touzout; software, Adil Mihoub, and Nabil Touzout; validation, Adil Mihoub; formal analysis, Adil Mihoub, and Nabil Touzout; investigation, Adil Mihoub, Mohammed Mesnoua, Nourelislm Siabdallah, Chawqi Benchikh, and Nabil Touzout; resources, Adil Mihoub, Nourelislm Siabdallah, and Chawqi Benchikh; data curation, Adil Mihoub, Mohammed Mesnoua, Reguia Zeguerrou, Saliha Benaoune, Jakub Černý, and Nabil Touzout; writing—original draft preparation, Adil Mihoub, and Aftab Jamal; writing—review and editing, Adil Mihoub, Domenico Ronga, Jakub Černý, and Aftab Jamal; visualization, Adil Mihoub, and Aftab Jamal; supervision, Adil Mihoub, and Jakub Černý; project administration, Adil Mihoub; funding acquisition, Jakub Černý, and Aftab Jamal. All authors reviewed the results and approved the final version of the manuscript.
Availability of Data and Materials: The data presented in this study are available on request from the first corresponding author.
Ethics Approval: Not applicable.
Conflicts of Interest: The authors declare that they have no conflicts of interest to report regarding the present study.
References
1. Naorem A, Jayaraman S, Dang YP, Dalal RC, Sinha NK, Rao CS, et al. Soil constraints in an arid environment challenges, prospects, and implications. Agronomy. 2023;13:220. doi:10.3390/agronomy13010220. [Google Scholar] [CrossRef]
2. Mihoub A, Boukhalfa-Deraoui N. Performance of different phosphorus fertilizer types on wheat grown in calcareous sandy soil of El-Menia, southern Algeria. Asian J Crop Sci. 2014;6:383–91. doi:10.3923/ajcs.2014.383.391. [Google Scholar] [CrossRef]
3. Khan I, Amanullah, Jamal A, Farooq O, Farhan Saeed M, Roberto M, et al. Partial substitution of chemical fertilizers with organic supplements increased wheat productivity and profitability under limited and assured irrigation regimes. Agriculture. 2022;12:1754. doi:10.3390/agriculture12111754. [Google Scholar] [CrossRef]
4. Ullah J, Shah S, Mihoub A, Jamal A, Saeed MF, Székely Á, et al. Assessing the effect of combining phosphorus fertilizers with crop residues on maize (Zea mays L.) productivity and financial benefits. Gesunde Pflanz. 2023;75:1995–2008. doi:10.1007/s10343-023-00829-0. [Google Scholar] [CrossRef]
5. Salman M, Inamullah, Jamal A, Mihoub A, Saeed MF, Radicetti E, et al. Composting sugarcane filter mud with different sources differently benefits sweet maize. Agronomy. 2023;13:748. doi:10.3390/agronomy13030748. [Google Scholar] [CrossRef]
6. Ashraf F, Chen Y. Synergistic effects of biochar and arbuscular mycorrhizal fungi on enhancing Elymus elymoides growth in saline coastal soil. Pak J Bot. 2023;55:119–26. doi:10.30848/PJB2023-SI(14). [Google Scholar] [CrossRef]
7. Zia A, Munsif F, Jamal A, Mihoub A, Saeed MF, Fawad M, et al. Morpho-physiological attributes of different maize (Zea mays L.) genotypes under varying salt stress conditions. Gesunde Pflanz. 2022;74:661–73. doi:10.1007/s10343-022-00641-2. [Google Scholar] [CrossRef]
8. Thomas B. Encyclopedia of applied plant sciences. Cambridge: Academic Press; 2016. [Google Scholar]
9. Elkelish AA, Alnusaire TS, Soliman MH, Gowayed S, Senousy HH, Fahad S. Calcium availability regulates antioxidant system, physio-biochemical activities and alleviates salinity stress mediated oxidative damage in soybean seedlings. J Appl Bot Food Qual. 2019;92. doi:10.5073/jabfq.2019.092.036. [Google Scholar] [CrossRef]
10. Abdelaal KA, El-Maghraby LM, Elansary H, Hafez YM, Ibrahim EI, El-Banna M, et al. Treatment of sweet pepper with stress tolerance-inducing compounds alleviates salinity stress oxidative damage by mediating the physio-biochemical activities and antioxidant systems. Agronomy. 2019;10:26. doi:10.3390/agronomy10010026. [Google Scholar] [CrossRef]
11. Swamy K. Origin, distribution, taxonomy, botanical description, genetic diversity and breeding of capsicum (Capsicum annuum L.). Int J Dev Res. 2023;13:61956–77. [Google Scholar]
12. López-Serrano L, Calatayud Á, López-Galarza S, Serrano R, Bueso E. Uncovering salt tolerance mechanisms in pepper plants: a physiological and transcriptomic approach. BMC Plant Biol. 2021;21:1–17. doi:10.1186/s12870-021-02938-2 [Google Scholar] [PubMed] [CrossRef]
13. Richards LA. Diagnosis and improvement of saline and alkali soils. Washington: US Government Printing Office; 1954. [Google Scholar]
14. Shalaby OA, Ramadan ME-S. Mycorrhizal colonization and calcium spraying modulate physiological and antioxidant responses to improve pepper growth and yield under salinity stress. Rhizosphere. 2024;29:100852. doi:10.1016/j.rhisph.2024.100852. [Google Scholar] [CrossRef]
15. Waśkiewicz A, Muzolf-Panek M, Goliński P. Phenolic content changes in plants under salt stress. In: Ecophysiology and responses of plants under salt stress. New York: Springer; 2013. p. 283–314. [Google Scholar]
16. Gupta B, Huang B. Mechanism of salinity tolerance in plants: physiological, biochemical, and molecular characterization. Int J Genom. 2014;2014:701596. [Google Scholar]
17. Balasubramaniam T, Shen G, Esmaeili N, Zhang H. Plants’ response mechanisms to salinity stress. Plants. 2023;12:2253. doi:10.3390/plants12122253 [Google Scholar] [PubMed] [CrossRef]
18. Touzout N. Efficacy of silicon in mitigating the combined phytotoxic effects of salt and insecticide in Solanum lycopersicum L. J Soil Sci Plant Nutr. 2023;23:5048–59. doi:10.1007/s42729-023-01381-6. [Google Scholar] [CrossRef]
19. Liang X, Li J, Yang Y, Jiang C, Guo Y. Designing salt stress-resilient crops: current progress and future challenges. J Integr Plant Biol. 2024;66:303–29. doi:10.1111/jipb.13599 [Google Scholar] [PubMed] [CrossRef]
20. Ahmad I, Munsif F, Mihoub A, Jamal A, Saeed MF, Babar S, et al. Beneficial effect of melatonin on growth and chlorophyll content in wheat (Triticum aestivum L.) grown under salt stress conditions. Gesunde Pflanz. 2022;74:997–1009. doi:10.1007/s10343-022-00684-5. [Google Scholar] [CrossRef]
21. Adil M, Samia H, Sakher M, El-Hafed K, Naima K, Kawther L, et al. Date palm (Phoenix dactylifera L.) irrigation water requirements as affected by salinity in Oued Righ conditions, North Eastern Sahara, Algeria. Asian J Crop Sci. 2015;7:174–85. doi:10.3923/ajcs.2015.174.185. [Google Scholar] [CrossRef]
22. Djaafri M, Salem F, Kalloum S, Desideri U, Bartocci P, Khelafi M, et al. A route for bioenergy in the sahara region: date palm waste valorization through updraft gasification. Energies. 2024;17:2520. doi:10.3390/en17112520. [Google Scholar] [CrossRef]
23. Kavvadias V, Le Guyader E, El Mazlouzi M, Gommeaux M, Boumaraf B, Moussa M, et al. Using date palm residues to improve soil properties: the case of compost and biochar. Soil Syst. 2024;8:69. doi:10.3390/soilsystems8030069. [Google Scholar] [CrossRef]
24. Abid W, Ammar E. Date palm (Phoenix dactylifera L.) wastes valorization: a circular economy approach. In: Mediterranean fruits bio-wastes: chemistry, functionality and technological applications. Cham, Switzerland: Springer; 2022. p. 403–30. [Google Scholar]
25. Visser ED, Seroka NS, Khotseng L. Recent advances in biochar: synthesis techniques, properties, applications, and hydrogen production. Processes. 2024;12:1111. doi:10.3390/pr12061111. [Google Scholar] [CrossRef]
26. Kul R, Arjumend T, Ekinci M, Yildirim E, Turan M, Argin S. Biochar as an organic soil conditioner for mitigating salinity stress in tomato. Soil Sci Plant Nutr. 2021;67:693–706. doi:10.1080/00380768.2021.1998924. [Google Scholar] [CrossRef]
27. Qin L, Wu Y, Hou Z, Jiang E. Influence of biomass components, temperature and pressure on the pyrolysis behavior and biochar properties of pine nut shells. Bioresour Technol. 2020;313:123682. doi:10.1016/j.biortech.2020.123682 [Google Scholar] [PubMed] [CrossRef]
28. Setter C, Borges F, Cardoso C, Mendes R, Oliveira T. Energy quality of pellets produced from coffee residue: characterization of the products obtained via slow pyrolysis. Ind Crops Prod. 2020;154:112731. doi:10.1016/j.indcrop.2020.112731. [Google Scholar] [CrossRef]
29. Arnon DI. Copper enzymes in isolated chloroplasts. Polyphenoloxidase in Beta vulgaris. Plant Physiol. 1949;24:1 [Google Scholar] [PubMed]
30. Davis BH. Carotenoids. In: Goodwin TW, editor. Chemistry and biochemistry of plant pigments. London: Academic Press; 1976. p. 38–166. [Google Scholar]
31. Velikova V, Yordanov I, Edreva A. Oxidative stress and some antioxidant systems in acid rain-treated bean plants: protective role of exogenous polyamines. Plant Sci. 2000;151:59–66. doi:10.1016/S0168-9452(99)00197-1. [Google Scholar] [CrossRef]
32. Ouzounidou G, Giannakoula A, Ilias I, Zamanidis P. Alleviation of drought and salinity stresses on growth, physiology, biochemistry and quality of two Cucumis sativus L. cultivars by Si application. Braz J Bot. 2016;39:531–9. doi:10.1007/s40415-016-0274-y. [Google Scholar] [CrossRef]
33. Nakano Y, Asada K. Hydrogen peroxide is scavenged by ascorbate-specific peroxidase in spinach chloroplasts. Plant Cell Physiol. 1981;22:867–80. doi:10.1093/oxfordjournals.pcp.a076232. [Google Scholar] [CrossRef]
34. Aebi H. Catalase in vitro. Method Enzymol. 1984;105:121–6. doi:10.1016/S0076-6879(84)05016-3. [Google Scholar] [CrossRef]
35. Cakmak I, Marschner H. Magnesium deficiency and high light intensity enhance activities of superoxide dismutase, ascorbate peroxidase, and glutathione reductase in bean leaves. Plant Physiol. 1992;98:1222–7. doi:10.1104/pp.98.4.1222 [Google Scholar] [PubMed] [CrossRef]
36. Habig WH, Jakoby WB. Assays for differentiation of glutathione S-Transferases. Method Enzymol. 1981;77:398–405. doi:10.1016/S0076-6879(81)77053-8. [Google Scholar] [CrossRef]
37. Gorji T, Tanik A, Sertel E. Soil salinity prediction, monitoring and mapping using modern technologies. Proc Earth Planet Sci. 2015;15:507–12. doi:10.1016/j.proeps.2015.08.062. [Google Scholar] [CrossRef]
38. Alkharabsheh HM, Seleiman MF, Battaglia ML, Shami A, Jalal RS, Alhammad BA, et al. Biochar and its broad impacts in soil quality and fertility, nutrient leaching and crop productivity: a review. Agronomy. 2021;11:993. doi:10.3390/agronomy11050993. [Google Scholar] [CrossRef]
39. Lamsaadi N, Farssi O, El Moukhtari A, Farissi M. Different approaches to improve the tolerance of aromatic and medicinal plants to salt stressed conditions. J Appl Res Med Aromat Plants. 2024;100532. doi:10.1016/j.jarmap.2024.100532. [Google Scholar] [CrossRef]
40. Lamsaadi N, Ellouzi H, Zorrig W, El Moukhtari A, Abdelly C, Savouré A, et al. Enhancing fenugreek (Trigonella foenum-graecum L.) productivity and seed quality through silicon-based seed priming under salt-stressed conditions. Russ J Plant Physl. 2024;71:70. doi:10.1134/s1021443723602562. [Google Scholar] [CrossRef]
41. Kamran M, Parveen A, Ahmar S, Malik Z, Hussain S, Chattha MS, et al. An overview of hazardous impacts of soil salinity in crops, tolerance mechanisms, and amelioration through selenium supplementation. Int J Mol Sci. 2019;21:148. doi:10.3390/ijms21010148 [Google Scholar] [PubMed] [CrossRef]
42. Atta K, Mondal S, Gorai S, Singh AP, Kumari A, Ghosh T, et al. Impacts of salinity stress on crop plants: improving salt tolerance through genetic and molecular dissection. Front Plant Sci. 2023;14:1241736. doi:10.3389/fpls.2023.1241736 [Google Scholar] [PubMed] [CrossRef]
43. Ahmed M, Tóth Z, Decsi K. The impact of salinity on crop yields and the confrontational behavior of transcriptional regulators, nanoparticles, and antioxidant defensive mechanisms under stressful conditions: a review. Int J Mol Sci. 2024;25:2654. doi:10.3390/ijms25052654 [Google Scholar] [PubMed] [CrossRef]
44. Semida WM, Beheiry HR, Sétamou M, Simpson CR, Abd El-Mageed TA, Rady MM, et al. Biochar implications for sustainable agriculture and environment: a review. S Afr J Bot. 2019;127:333–47. [Google Scholar]
45. Wang J, Wang S. Preparation, modification and environmental application of biochar: a review. J Clean Prod. 2019;227:1002–22. doi:10.1016/j.jclepro.2019.04.282. [Google Scholar] [CrossRef]
46. Agbna GH, Ali AB, Bashir AK, Eltoum F, Hassan MM. Influence of biochar amendment on soil water characteristics and crop growth enhancement under salinity stress. Int J Eng Works. 2017;4:49–54. [Google Scholar]
47. Rajapaksha AU, Chen SS, Tsang DC, Zhang M, Vithanage M, Mandal S, et al. Engineered/designer biochar for contaminant removal/immobilization from soil and water: potential and implication of biochar modification. Chemosphere. 2016;148:276–91. doi:10.1016/j.chemosphere.2016.01.043 [Google Scholar] [PubMed] [CrossRef]
48. Thomas SC, Frye S, Gale N, Garmon M, Launchbury R, Machado N, et al. Biochar mitigates negative effects of salt additions on two herbaceous plant species. J Environ Manage. 2013;129:62–8. doi:10.1016/j.jenvman.2013.05.057 [Google Scholar] [PubMed] [CrossRef]
49. Wu Y, Wang X, Zhang L, Zheng Y, Liu X, Zhang Y. The critical role of biochar to mitigate the adverse impacts of drought and salinity stress in plants. Front Plant Sci. 2023;14:1163451. doi:10.3389/fpls.2023.1163451 [Google Scholar] [PubMed] [CrossRef]
50. Simkin AJ, Kapoor L, Doss CGP, Hofmann TA, Lawson T, Ramamoorthy S. The role of photosynthesis related pigments in light harvesting, photoprotection and enhancement of photosynthetic yield in planta. Photosynth Res. 2022;152:23–42. doi:10.1007/s11120-021-00892-6 [Google Scholar] [PubMed] [CrossRef]
51. Pecherina A, Dimitrieva A, Mudrilov M, Ladeynova M, Zanegina D, Brilkina A, et al. Salt-induced early changes in photosynthesis activity caused by root-to-shoot signaling in potato. Int J Mol Sci. 2024;25:1229. doi:10.3390/ijms25021229 [Google Scholar] [PubMed] [CrossRef]
52. Dubey RS. Photosynthesis in plants under stressful conditions. In: Handbook of photosynthesis. New York: CRC Press; 2018. p. 629–49. [Google Scholar]
53. Sabater B, Rodrguez MT. Control of chlorophyll degradation in detached leaves of barley and oat through effect of kinetin on chlorophyllase levels. Physiol Plant. 1978;43:274–6. [Google Scholar]
54. Helaoui S, Boughattas I, Mkhinini M, Ghazouani H, Jabnouni H, El Kribi-Boukhris S, et al. Biochar application mitigates salt stress on maize plant: study of the agronomic parameters, photosynthetic activities and biochemical attributes. Plant Stress. 2023;9(11):100182. doi:10.1016/j.stress.2023.100182. [Google Scholar] [CrossRef]
55. Singh A, Agrawal M. Effects of ambient and elevated CO2 on growth, chlorophyll fluorescence, photosynthetic pigments, antioxidants, and secondary metabolites of Catharanthus roseus (L.) G Don. grown under three different soil N levels. Environ Sci Pollut Res. 2015;22:3936–46. doi:10.1007/s11356-014-3661-6 [Google Scholar] [PubMed] [CrossRef]
56. Shams M, Ekinci M, Ors S, Turan M, Agar G, Kul R, et al. Nitric oxide mitigates salt stress effects of pepper seedlings by altering nutrient uptake, enzyme activity and osmolyte accumulation. Physiol Mol Biol Plants. 2019;25:1149–61. doi:10.1007/s12298-019-00692-2 [Google Scholar] [PubMed] [CrossRef]
57. Lalarukh I, Amjad SF, Mansoora N, Al-Dhumri SA, Alshahri AH, Almutari MM, et al. Integral effects of brassinosteroids and timber waste biochar enhances the drought tolerance capacity of wheat plant. Sci Rep. 2022;12:12842. doi:10.1038/s41598-022-16866-0 [Google Scholar] [PubMed] [CrossRef]
58. Hasanuzzaman M, Bhuyan MB, Zulfiqar F, Raza A, Mohsin SM, Mahmud JA, et al. Reactive oxygen species and antioxidant defense in plants under abiotic stress: revisiting the crucial role of a universal defense regulator. Antioxidants. 2020;9:681. doi:10.3390/antiox9080681 [Google Scholar] [PubMed] [CrossRef]
59. Hassanpour SH, Doroudi A. Review of the antioxidant potential of flavonoids as a subgroup of polyphenols and partial substitute for synthetic antioxidants. Avicenna J Phytomed. 2023;13:354. doi:10.22038/ajp.2023.21774 [Google Scholar] [PubMed] [CrossRef]
60. Pandey KB, Rizvi SI. Plant polyphenols as dietary antioxidants in human health and disease. Oxid Med Cell Longev. 2009;2:270–8. doi:10.4161/oxim.2.5.9498 [Google Scholar] [PubMed] [CrossRef]
61. Quartacci MF, Sgherri C, Frisenda S. Biochar amendment affects phenolic composition and antioxidant capacity restoring the nutraceutical value of lettuce grown in a copper-contaminated soil. Sci Hortic. 2017;215:9–14. doi:10.1016/j.scienta.2016.12.002. [Google Scholar] [CrossRef]
62. Antón-Herrero R, Vega-Jara L, García-Delgado C, Mayans B, Camacho-Arévalo R, Moreno-Jiménez E, et al. Synergistic effects of biochar and biostimulants on nutrient and toxic element uptake by pepper in contaminated soils. J Sci Food Agric. 2022;102:167–74. doi:10.1002/jsfa.11343 [Google Scholar] [PubMed] [CrossRef]
Cite This Article
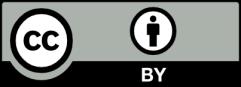
This work is licensed under a Creative Commons Attribution 4.0 International License , which permits unrestricted use, distribution, and reproduction in any medium, provided the original work is properly cited.