Open Access
REVIEW
Exploring Metal Based Nanoparticles for Boosting Plant Tolerance to Heavy Metals and Trace Element Contamination
1 Water and Environmental Study Centre, King Faisal University, Al-Ahsa, 31982, Saudi Arabia
2 Department of Environmental Sciences, Government College University Faisalabad, Faisalabad, 38000, Pakistan
3 Department of Biological Sciences and Technology, China Medical University, Taichung, 40402, Taiwan
4 Date Palm Research Center of Excellence, King Faisal University, Al-Ahsa, 31982, Saudi Arabia
5 Central Laboratories, King Faisal University, Al-Ahsa, 31982, Saudi Arabia
6 Department of Biology, Faculty of Science, University of Tabuk, Tabuk, 71491, Saudi Arabia
* Corresponding Authors: Shafaqat Ali. Email: ,
; Muhammad Munir. Email:
(This article belongs to the Special Issue: Abiotic Stress Impacts on Plant Physiology and Their Alleviation)
Phyton-International Journal of Experimental Botany 2024, 93(11), 2683-2705. https://doi.org/10.32604/phyton.2024.055898
Received 09 July 2024; Accepted 08 October 2024; Issue published 30 November 2024
Abstract
Heavy metal pollution in agricultural soils is a significant challenge for global food production and human health with the increasing industrialization and urbanization. There is a concern about introducing innovative techniques that are eco-friendly, cost-effective, and have the potential to alleviate metals, enhance crop growth, and protect plants against various environmental threats. For this, nanotechnology is one of the promising solutions having various applications in almost every field of life. This review explores various nano-based strategies that use nanoparticles (NPs) to lessen the harmful effects that heavy metals have on plants. Incorporated literature including published research and review papers from the year 2015 to 2023. This review paper gives a thorough review of the current situation regarding heavy metal contamination in agricultural soils and how it affects plant health. The necessity of finding practical and eco-friendly ways to address these issues is emphasized, paving the way for the introduction of NPs. Then, it highlighted the mechanistic route of heavy metal toxicity alleviation in plants by their application as well as their long-term efficiency and prospects. This review also elaborated on various synthesis methods (physical, chemical, and green), but the emphasis on the green synthesis of NPs by utilizing plant extract offers dependable and sustainable benefits over traditional physicochemical techniques. Under trace element stress, NPs application enhances plant antioxidant defense system, ameliorating structural changes, immobilizing trace elements in growth media, and improving the physio-chemical properties of soil as well. However, there are still numerous limitations present on how these materials are synthesized, applied, and appropriately absorbed by plant cells. It is recommended to promote and fund long-term research to assess the long-term effects of using NPs on plant development, soil health, and possible environmental repercussions.Keywords
In recent years, due to the fast industrial and urban expansion, there has been rising concern about the potential buildup of heavy metals (HMs) in agricultural soil [1,2]. Mainly HMs are the polluting agents [3] present in different environmental components. They also have deleterious effects on vegetation, animals, and humans because of their persistent, non-biodegradable, and poisonous nature [4–9]. In soil, HMs can be found in many different forms with a wide range in solubility and bioavailability [10,11]. Their excessive buildup in plant tissue interferes with several biochemical, physiological, and morphological functions either directly or indirectly, which affects crop productivity [12,13]. It depends on plant type, HM content, and exposure duration, which directly has a significant effect on the bioavailability of HMs. In various plants, they also can cause oxidative stress and decreased chlorophyll content, which results in photosynthesis reduction [14]. Abiotic variables and climate change are creating uncertainty in agriculture productivity by altering growing conditions such as temperature and water availability that affect crop yield. MTEs are beneficial in trace amounts, but their elevated concentrations during germination are dangerous because they can cause seed death and subsequently lessen plant germination overall [15,16]. Hence, environmental cleanup of these toxic HMs is of the utmost importance [17], such as the application of nanotechnology [18], particularly green technology, which holds great promise for resolving these issues.
Nanotechnology refers to the application of materials generally at the nanometer scale, typically between 1 and 100 nanometers, to attain distinctive properties. Recent nanotechnology advancements have facilitated the widespread use of nanoparticles (NPs) in a variety of science fields, industrial firms, and research. NPs have been identified in agricultural soil, including metals and their oxides, carbon compounds, zeolites, and bimetallic NPs [19,20]. The plants may be able to produce NPs, which are natural substances used to enhance plant morphology without having any adverse consequences. Recently, the application of NPs for abiotic stress conditions has obtained more attention [21]. According to studies, NPs can be applied to decrease the MTEs toxic effects in plants [22,23]. They can enhance morphogenesis of plants like metallic ones, including zinc-oxide (ZnO), and copper-oxide (CuO), the most prevalent NPs used in agriculture and industry. However, due to a lack of mechanisms that have not been clarified or further researched, some of the potential of NPs remains still hidden [9]. This comprehensive review investigates how nanotechnology can be applied to treat plants that harbor HMs in comparison to traditional methods. This article extensively analyzes and deliberates upon the benefits, hurdles, opportunities, and limitations linked to utilizing NPs to extract HMs from plants. Its objective is to furnish environmentalists with an enriched understanding of nanomaterial capabilities and their potential application in studies ranging from laboratory-scale to large practical scales. This review stands as an invaluable asset for researchers and practitioners interested in exploring nanotechnology-driven remedies for the effective and sustainable remediation of heavy metals, all while carefully considering the wider environmental impacts.
It is hypothesized that the use of NPs, which are distinguished by their special physicochemical qualities, is thought to be able to lessen the harmful effects of HMs toxicity and stress on plants via several different processes. These strategies could involve the production of defensive biochemical responses, modification of metal absorption and translocation pathways, and manipulation of plant physiological processes using NPs.
The objectives of this review are to thoroughly examine the effects of HMs on plants and the role of ROS in influencing plant health and growth with a specific focus on their interactions within soil. Additionally, aims to investigate nano-based approaches especially, the use of green synthesized NPs to improve plant resistance and mitigate stress and adverse effects on plant development. This study is significant because it has the potential to address a pressing environmental issue, HMs toxicity of agricultural soils. The results of this review could guide sustainable agriculture practices since HMs pollution seriously jeopardizes ecosystem health and food security. The work aids in the creation of environmentally friendly techniques for soil remediation and crop improvement by clarifying the processes of NPs action in plants. It also highlights the necessity of conducting additional studies to investigate the combined use of NPs in agricultural systems, as this could improve crop resilience in contaminated settings and support sustainable agricultural productivity.
2 Applications of Nanotechnology for Environmental Remediation
Anthropogenic and natural activities are the two main causes of the complex problem of HMs pollution in the environment [24]. High concentrations of HMs in the air, water, and soil are a result of the fast expansion of industry and metropolitan areas, which has also amplified emissions, released pollutants, and raised resource demand [25]. During industrial activities, HMs are discharged into the atmosphere and can come back to the ground via depositions causing adverse effects as shown in Fig. 1. Wastewater discharges, including home sewage and industrial effluents are also an alarming environmental source of HMs [26–30]. Soils are the major repository of HMs in terrestrial ecosystems. Additionally, HMs are notorious for their significant toxicity in flora and fauna [2,31]. Lead (Pb), copper (Cu), and zinc (Zn) contaminated soils, all individually limit microbial biomass and enzymatic activities. These metals primarily concentrate in plants, where they transform into harmful forms that can infect people and other animals with deadly diseases. In addition to affecting plant growth, toxic HMs can alter the physiological and biochemical processes, resulting in structural damage and deformation [32–34]. The presence of HMs in the food web results ultimately in food chains has been the subject of numerous research in developed countries.
Figure 1: Anthropogenic sources of heavy metals and their key consequences on environment (air, water & soil)
Nanotechnology is also one of the innovative technologies that have promise for raising agricultural output using effective nano-pesticides and nano-herbicides to enhance soil properties and pathogen mitigation. The unique qualities and characteristics of NPs provide a huge potential for their various applications in therapeutic [35] and agriculture [36,37]. However, to address the safety and health issues posed by their application, more research/investigation is necessary. NPs play a pivotal role in alleviating HMs toxicity in plants through several mechanisms. Primarily, NPs can bind and immobilize HMs in the soil, reducing their availability for plant uptake. They also facilitate the breakdown or transformation of toxic metals into less harmful forms. Additionally, NPs can enhance the plant’s defense mechanisms, boosting its ability to tolerate and detoxify HMs by triggering antioxidant production or stimulating stress-response pathways. Overall, NPs are contributed to mitigate HMs toxicity in plants by reducing uptake, transforming toxic forms, and enhancing the plant’s natural defense mechanisms [38,39].
Metals and metal-oxides NPs are also the most explored NPs for environmental remediation [40]. Cerium oxide (CeO) NPs have been applied in a variety of soil remediation and agricultural applications as they are cost-effective and maintain their catalytic characteristics under stressful environments. The ability of CeO NPs to switch between valence states allows them to mimic the functions of certain enzymes like superoxide dismutase (SOD), catalase (CAT), phosphatase, and phosphotriesterase (PTE) to reduce the oxidative stress harmful effects [41]. The NPs could be used in the agricultural sector to treat plant diseases and enhance growth and nutrient contents [42–45]. Novel nanotechnological techniques are being developed to improve the characteristics of NPs that are being applied in agriculture for sustainable growth (Fig. 2). These methods should be environmentally friendly and can boost plant and crop growth without affecting the surrounding environment.
Figure 2: Eco-friendly applications of metal based nanoparticles for sustainable agriculture
3 Synthesis of Nanoparticles: Green vs. Physio-Chemical Methods
Physical and chemical NPs synthesis methods use materials and innovative equipment, which can harm the environment. To support the expanding use of NPs across a variety of industrial facilities, the field of nanotechnology have thus switched towards environmentally sustainable and economically feasible “green” synthesis over the past ten years. In contrast to conventional synthetic procedures, green synthesis offers dependable and sustainable ways to produce NPs [46]. The biological method of synthesizing NPs includes the utilization of microorganisms such as fungi, yeast, and plant parts extract as reducing agents of the metal ions [47,48]. Traditional methods have been utilized for a long time, but studies have shown that green method by plant extract seems more efficient because they have fewer failure risks and are less expensive [49]. Plant-based synthesis of NPs was quite simple as a metal salt is made with plant extract, and the process takes only a few minutes to a few hours at standard room temperature [50].
In general, top-down and bottom-up approaches are the two main ways to synthesize NPs [51–53]. The top-down approach incorporated the change of bulk materials to thinner crystallites through a physical pathway including milling and ionic sputtering (enormous mechanical energy sources) [54]. However, this strategy results in some drawbacks, causing secondary impressions, surface chemistry, and physicochemical properties alteration of synthesized NPs [55]. More notably by this approach, NPs are mostly unfeasible. Meanwhile, the bottom-down strategy involves particle formation through building block creation from ultra-small particles followed by assembling them [56,57].
The NPs synthesis using plant extracts is an environmentally friendly method as chemical synthesis might have unfavorable negative consequences on the environment. A wide range of vegetal species from different families have been used for the synthesis of NPs, such as Garcinia cambogia extract [58] and flower extract of Japanese honeysuckle (Lonicera Japonica) [59] in Ag & gold (Au) NPs synthesizing, respectively. Cacumen platycladi leaf extract can also be employed for Au NPs production [60]. Synthesized NPs by this method have been used leaf extracts as biological reducing agents and isolated their active ingredients. Plant extracts also have been widely used to produce copper oxide (CuO) NPs by using Punica granatum peels extract [61], Azadirachta indica plant extract [62,63], Abutilon indicum leaf extract [64], Rheum palmatum L. root extract [65] and aqueous extract of Hyptis suaveolens L [66]. Consequently, extracts of plants showed an ideal source for NPs production. Plant-based fabrication processes show less energy consumption and improved NPs stability [67].
4 Mechanistic Approach behind Metal Based Nanoparticles Interaction with Soil and Plants
The mechanisms by which NPs interact with soil and plants are complex and depend on their physicochemical properties and environmental conditions. In soil, NPs can interact with soil particles, organic matter, and microorganisms, which can affect their mobility, bioavailability, and toxicity to plants. For example, the surface charge and hydrophobicity of NPs can influence their adsorption and aggregation on soil particles.
Inside plants, NPs can enter plant tissues through various pathways, such as root uptake and foliar application. Once inside, NPs can interact with cell membranes, organelles, and metabolic pathways, which can affect their uptake, translocation, and toxicity to plants. For example, the size, shape, and surface chemistry of NPs can influence their penetration and translocation in plant tissues [68]. As smaller NPs tend to penetrate plant tissues more easily, surface alteration can increase or inhibit their uptake. The mechanism of mode of action of NPs also depends on the target organism and exposure route. In general, NMs can interact with biological membranes and proteins, disrupt cellular functions, generate ROS, and induce inflammation or other immune responses. For example, AgNPs can interact with bacterial cell membranes and disrupt their functions, while carbon nanotubes can induce oxidative stress and inflammation in lung cells.
5 Effects of NPs on Metals Uptake and Metalloids Reduction in Food Crops
Reducing the uptake of toxic metals and metalloids by food crops is crucial for ensuring food safety and preventing potential health risks. Preventing the uptake of metals by NPs is also important for ensuring food safety and preventing potential health risks. To achieve this, it is important to carefully evaluate the physicochemical properties of NPs and their interactions with plants and soil.
The interaction between NPs and HMs in plants is complex and can have both positive and negative effects on plant growth and physiology. Under metal stress, NPs can help to mitigate their toxic effects on plants by reducing their uptake and accumulation in plant tissues while enhancing photosynthesis and nutrient uptake, as shown in Table 1. For example, zinc oxide nanoparticles (ZnO NPs) have been shown to increase the accumulation of zinc in plants, which can help alleviate the toxic effects of HMs such as cadmium (Cd) and lead.
Regarding antioxidant enzymes, NPs can potentially alter the plant defense system under metal stress. For instance, rice seedlings were grown in hydroponic experiments under Cd stress, TiO2 NPs treatment boosted SOD, CAT, and peroxidase (POD) activity. The antioxidant enzyme activities in a variety of plants under Cd stress, including wheat [69] and rice [29] were dramatically increased with a foliar spray of ZnO NPs [22]. On the other hand, researchers also demonstrated the potential of TiO2 NPs negative impacts on the physiology and growth of maize. Increased TiO2 NP treatments dramatically decreased the chlorophyll contents of maize crops.
CeO2 NPs can promote root growth, activate the antioxidant enzymes [70–72] and can persuade oxidative stress. They also improve agronomic features, photosynthetic pigment content, and antioxidant enzymes in plants exposed to salinity stress and enhanced parameters of growth. Various plants react in different ways towards the presence of CeO2 NPs. For instance, wheat (Triticum aestivum L.) treated plants with approximately 500 mg kg−1 CeO2 NPs in the soil results in increased growth, more grain yield, and shoot biomass [73–75]. Contrarywise, it was observed that CeO2 NPs suppressed photosynthesis in barley by lowering transpiration and stomatal conductance at 1000 mg kg−1 [76].
The uptake of TEs by plants depends merely on their chemical form as well as NPs effectiveness. For instance, in cucumber plants, selenium (Se) application can reduce the Cd concentration in roots. Researchers have demonstrated that for reducing plants’ TEs uptake levels, selenite has shown more effectiveness than selenate. The Cd concentration reduction by using ZnO, Fe and Si NPs on wheat has also been studied [22,29,69]. These NPs have significantly increased wheat growth, biomass, and photosynthesis rate.
The effect of NPs on metal bioavailability in the environment is largely dependent on their speciation. Metal sorption and desorption can be impacted by the surface chemistry of NPs, including their coating and functional groups [77–79]. The binding or release of metals may be facilitated by functional groups on NPs, which could impact the metals’ bioavailability. The size and surface area of NPs can change when they aggregate, which can affect their reactivity with metals. Due to their limited mobility, aggregated NPs may lower the bioavailability of metals. However, the release of metal ions during NPs dissolution can boost bioavailability [80,81]. NPs and metal ions in the surrounding environment have the potential to form complexes [15]. These complexes’ characteristics may affect the speciation of metals and their consequent bioavailability. Metal binding and release can also be influenced by ligand exchange events that occur between NPs and the surrounding ligands.
6 Effects of Nanoparticles Seed Priming and Foliar Application on Plant Growth
Nano-priming can be applied to seeds to protect them during storage, enhance germination, boost plant growth, as well as to increase crops’ resistance to biotic or abiotic stress conditions. It is an efficient procedure that can modify the signaling pathways of seeds and the whole plant lifespan. Seed priming is often classified as either abiotic or biotic priming, depending on the priming agent used. Abiotic priming, which covers both traditional and advanced methods of seed priming, is described as the use of non-living chemical and physical agents. Additionally, biofortification of seeds by nano-priming can be employed to encourage an improvement in food quality and production. Using these metal-based NPs for seed nano-priming has significant promise for use in agriculture. For instance, Kumar et al. [82] discovered that ZnO NPs promote germination and seedling development (shoot and root length). At high concentrations, seed priming with ZnO and FeO NPs promoted plant development, boosting spike length, plant biomass, leaves chlorophyll levels, and photosynthetic parameters [19]. Maswada et al. [83] demonstrated that seed priming with iron oxide nanoparticles (Fe2O3 NPs) of sorghum plants increased seed germination and plant growth. Photosynthetic pigments and biomass increased after treatment at 500 mg/mL.
Despite significant advancements, the application of physical techniques for NPs seed priming still requires extensive research in terms of priming protocols, appropriate dose, dose rate, and exposed period. On the other hand, several studies showed that foliar application of NPs was better than seed priming treatment [84,85]. This is further demonstrated by Abdel-Aziz et al. [86], who investigated effects of applying either carbon nanotubes (CNTs) or nanochitosan (Cs) unaccompanied or combined with NPK as fertilizers to french bean plants. Foliar treatment reduced the time to harvest as 80 days (37.5%) without affecting yield when related to seed priming treatment & control as 110 days. The NPs applications for foliar and seed priming have both been shown in various studies to have multiple benefits for plant growth. Raj et al. [87] has conducted an experiment on the growth, yield, and economics of Bt cotton using nano-zinc seed treatment and foliar application. Nano ZnO foliar application (@1000 ppm) showed sophisticated productivity as 2718 kg ha−1 in seed cotton, an increase in plant height as 190.1 cm, and soil plant analysis development (SPAD) chlorophyll meter value than other concentrations. Various studies have shown positive effects of seed nano-priming and foliar applications like enhancing the growth of plants and development, improving yield, and nutritional food quality but still limitations present for their use. Under some conditions, foliar application of NPs alleviates metal toxicity under metal stress as compared to seed priming. NPs, when combined with additional amendments like farmyard manure (FYM), charcoal, and bio-nanocomposites, also play a key role in reducing metal toxicity, promoting plant development, and affecting the bioavailability of TEs. For instance, Ali et al. [29] assessed the effectiveness of ZnO NPs at various concentrations in Cd-contaminated soil to test Cd alleviation on rice plants alone and with biochar (1.0 w/w). The findings demonstrated that either independently or in combination with biochar, rice plant biomass and photosynthesis were enhanced by Zn NPs. In general, both seed priming and foliar application of metal-based NPs have the potential to improve plant growth and productivity. However, further research is needed to determine the optimal concentration and timing of NPs application for different plant species and growth stages, as well as the potential long-term effects on soil health and the environment.
7 Mechanisms of Trace Elements Tolerance in Plants
7.1 Nanoparticles’ Influence on Plant Attributes Focuses on the Tes Absorption and Translocation
Plants that are exposed to an environment polluted with metals suffer significant effects on their vegetative and reproductive development, which ultimately affects agricultural productivity and performance [88,89]. The complicated physiological characteristic of TE absorption and subsequent accumulation in plants is primarily controlled by the element transporters and metal chelators present in the plant system [80]. When present in optimal quantities, these advantageous components raise the nutritional content of plants and have an impact on several processes vital to healthy plant development and productivity. When they are present in excess, though, they become poisonous to plants and cause them to become less able to absorb and accumulate other non-essential elements [90,91]. Several strategies were used to reduce these emerging pollutants’ phytotoxicity in the soil plant system [92,13]. The nexus of nanotechnology and plant science has attracted a lot of attention lately, especially when it comes to comprehending how NPs affect plants’ ability to tolerate TE toxicity [93]. Because of their distinct physicochemical characteristics, NPs provide opportunities as well as problems to the field of plant biology.
7.2 Antioxidant Defense Systems Enhancement
In plants, mostly HMs can cause oxidative stress that results mainly in reactive oxygen species (ROS) production [94,95]. In such a situation, the application of NPs was observed to be more effective on plants in enhancing the antioxidant defense system and reducing ROS. The ROS have been shown in recent years to be signals that control a variety of developmental ways and responses to the environment [96,97]. Additionally, an increase of TEs in plants might result in oxidative bursts due to enhancement in ROS generation and lipid peroxidation. In this case, NPs reduced the toxicity that TE induced in the plants. To enhance plant development in wheat and lower oxidative stress and Cd content, an experiment was conducted by Latif et al. [19] using Zn and Fe NPs. Both NPs enhanced Fe and Zn concentrations in wheat while reducing oxidative stress and Cd concentrations. Arsenic toxicity in plants can result in the production of ROS, which can harm cells oxidatively and disrupt metabolism. For instance, the MDA concentration (17.5%–30.8%) of rice under arsenic stress was decreased by 10−100 mg L−1 ZnO NPs amendment [98]. Similar research has demonstrated that Fe3O4 NPs have good impacts on root growth and integrity of membranes by lowering the MDA contents in maize grown in soil amended with NPs (50, and 500 mg/kg) for the duration of 28 days compared with control [99]. The growth, chlorophyll content, and antioxidant activity of basil plants were enhanced by the foliar application of ZnO NPs alone or in combination with Cu and Mn NPs [100]. Foliar application effects of Si and TiO2 NPs on oxidative burst and Cd uptake by rice was observed by [19]. Nanoparticles lessened EL, and MDA and improved SOD, POD, CAT, and ascorbate peroxidase over the treatment. Thus, findings showed that foliar application of NPs was successful in lowering oxidative burst and enhancing antioxidant defense. Jalil et al. [101] examined the mitigating effects of SiO NPs as well as the physiological and molecular responses of rice genotype “9311” to Cd stress. The study highlights that rice plants’ physiological functions are enhanced by the SiO NPs applications and their tolerance to Cd stress as well. Foliar application under abiotic stress can enhance chlorophyll content, increase K+ absorption, modify Na+ levels, and lessen damage in cell wall compared to untreated plants. The effective strain response of tomato subjected to CuO NPs depends on hydrogen sulphide (H2S)-mediated persulfidation of antioxidize [102]. And show that it controls CAT, APX, and POD activity, improving the plant’s reaction to oxidative stress. On the other hand, Se may protect plants against various hazardous TEs by regulating their intake and transport in plant parts and can be beneficial to promote plant growth and photosynthesis and control ROS at low-concentration applications. But the reverse scenario can also be observed at high quantities that do not show benefits of reducing oxidative stress [103].
7.3 Plants Morphological and Physiological Alterations by TEs
Plant structural changes brought on by TEs stress result in decreased growth and yield. For example, in the presence of manganese (Mn), Cu, nickel (Ni), leaves turn pale in color, pale pink between veins and leaf chlorosis happens respectively in plants [104,105]. By modifying gene transcription, enhancing antioxidant defense mechanisms, and generating structural alterations, NPs prevent TEs from moving from roots to shoots in plants.
Pérez Velasco et al. [106] assessed the impacts of ZnO NPs application practices, surface modification, and morphology on the growth and biomass on tomato plants. The findings have shown that ZnO NPs treated tomato plants meaningfully boosted in height, stem diameter, and its dry weight. Maraei et al. [107] assessed the impacts of treated TiO2 NPs on the nutritional worth and broccoli’s growth. The results have shown that TiO2 foliar treatments had a satisfactory impact on vegetative growth as compared to the control. Hussain et al. [108] results demonstrated that Ti NPs application has a considerable effect on the plant’s growth, and significantly increases the soybean diameter. Lower TiO2 NP concentrations (2 and 10 ppm) have also been revealed to be advantageous as enhance the shoots length of wheat seedlings, though with the higher TiO2 NP concentrations exposed, neutral effects and the repressive effects have also been detected at bulk/greater concentrations of TiO2 NPs. As 53% shoot length, 33% root length, 44% dry weight, and 48% fresh weight of wheat have also enhanced when calcium phosphate and TiO2 NPs (both 40 ppm) are used together in contrast to the controls [109]. An experiment was carried out to induce Cd stress tolerance in maize by the exogenous application of silicon nanoparticles (Si NPs). Three levels of Cd (0, 15, and 30 ppm) and five dosages of Si NPs (0, 100, 200, 300, and 400 ppm) were used for the maize hybrid (SF-9515). The biochemical measurements and morphological characteristics of maize were among the response factors. The findings showed that, for maize plants, a Cd level of 30 ppm remained the most severe, as measured by minimal characteristics such as shoot length (39.35 cm), shoot fresh weight (9.52 g), and shoot dry weight (3.20 g) [110].
Fe NPs were administered in a variety of ways to strengthen the wheat crop. Fe NPs seed priming, however, was found to be more effective in increasing the contents of proline, chlorophyll a, b, and total chlorophyll in wheat plants when applied topically at concentrations of 10, 20, and 30 mg L−1 [131]. CeO2 NPs meaningfully enhanced fruit weight at doses of 10 mg L−1, whereas increasing the stem elongation at doses of 1 to 10 mg L−1 [132,133]. Thus, fewer studies have compared the functional variations in plants in the response towards metals and NPs, therefore, more comprehensive research would be crucial to understand the metals tolerance mechanism.
8 Interaction between NPs and Trace Elements
The interaction between NPs and soil TEs is a complex and dynamic process that can affect the mobility, bioavailability, and toxicity of both NPs and TEs in soil. Through adsorption and desorption, NPs can absorb soil particles, organic matter and TEs. This can affect the mobility and bioavailability of them in soil, as well as the potential for uptake by plants. NPs with high surface reactivity can interact more strongly with TEs in soil, leading to increased adsorption and reduced bioavailability. However, this can also increase the potential for the release of TEs under certain conditions, such as changes in pH or ionic strength.
8.1 TE Bioavailability Alteration in Soil
NPs due to their high sorption capacity, may diminish and/or limit the bioavailability TEs in plants. Depending on the concentration and NPs used, as well as how long NPs and TEs interacted, NPs may immobilize TEs in growth media as shown in Fig. 3. However, soil characteristics are essential for NP dispersion, aggregation, stability, transport, and bioavailability as well as their release into the soil. Labile TE fractions can easily be changed into stable TE fractions using NPs. Due to their nanoscale properties, the mobility, bioavailability, and toxicity of co-existing contaminants like TMs and hazardous organics in the soil environment could all be affected by NPs. The bioavailability of TEs in the soil is influenced by both metallic and organic NPs. ZnO NPs impact on soil TEs concentration has been studied by Baysal et al. [134]. According to the study, ZnO NPs exposure caused a decrease in the amount of Al, Mg, Ca, and Cu in the soil as well as the adsorption of TEs into soil. However, the exposure of ZnO NPs enhanced the Fe content while not affecting Cu or Ni. The impact of nanoscale zerovalent iron (nZVI) and ferrous sulphide (FeS NPs) supported by biochar on the availability of Pb and Fe, as well as the micro-ecology in lead-contaminated soil was examined [135]. In excessive Pb-contaminated alkaline soil, nZVI @ BC exhibited the best immobilization effect on Pb, although Fe3O4-NPs toxic consequences on soil micro-ecology was only marginal. According to Rossi et al. [136], on soybean CeO2 NPs did not affect Cd accumulation, but it markedly boosted Ce accumulation within tissues of plants, particularly in older leaves & roots.
Figure 3: Mechanism and interaction of nanoparticles with the plants and soil and its positive effects on plant growth and soil properties
8.2 Soil Physicochemical Properties Enhancement
On soils, limited data showed that metal oxide nanoparticles affect biochemical parameters. By changing the speciation of TEs and altering the physiological, biochemical, and biological characteristics of soil, NPs successfully immobilized TEs in soil solution. If kinetic limitations are removed, NPs, particularly ZnO NPs, dissolve chemically in the soil’s aqueous phase can either directly or indirectly change the soil’s properties. In the same way, using maize as the test crop, Verma et al. [137] carried out a model experiment in a greenhouse to investigate how ZnO NPs interact with soil. When ZnO NPs were applied, soil pH and organic carbon levels significantly decreased, EC significantly increased, and P, Zn, and Fe availability significantly increased. Through the consumption of reductive material and increased quantity of NPs, Zhang et al. [138] detected an upsurge in soil redox potential after the use of ZnO NPs and CuO NPs. The detrimental effects of TEs on soil lead to a decline in soil quality.
8.3 Effect on Biological Properties of Soil
The biological characteristics of the soil are influenced by the presence of HMs. Pb, Cu, Cr, and Cd concentrations in soil have an impact on biomass, soil respiration, and enzyme activity. They have been shown to alter microbial colonies and biomass, may have a positive impact on rhizospheric microorganisms and biomass content, and can increase soil fertility. Rajput et al. [139] studied both CuO and ZnO NPs applications and have shown that they promoted bacterial and fungal communities in soil through increasing bacterial and fungus groups. However, at higher concentrations than 250 mg/kg soil, the beneficial effects of NPs on soil microorganisms were hampered [71]. On the sunflower plant cultivated under Cr stress, the administration of CeO2 NPs greatly increased the enzymatic activities [140]. So, based on previous research findings, it can be demonstrated that NPs, because of their distinctive characteristics and useful applications in various agricultural soils have drawn a lot of interest, but limited studies highlight their role in improving biological properties of soil. So, more work has to been done in future regarding that.
9 Limitations of Using Metal based NPs
The presence of metallic NPs in soil is a serious problem because they can cause secondary contamination, which increases the hazards to the environment and public health. Due to their small size and larger surface area, they are more mobile and can easily leach into groundwater or travel through the air, causing accidental dispersion beyond the original location of pollution [141,142].
Metal-based NPs effectiveness is often concentration-dependent as their dosages may not result in substantial benefits, in contrast, high dosages can be toxic to plants. This may complicate NPs application, in order to determine their optimal dosage for improving plant growth without causing remains a challenge [143]. The mechanisms through which NPs alleviate HMs stress are still not fully understood. While some research indicated that NPs could lower oxidative stress and increase antioxidant enzyme activity, further research is needed to determine the precise molecular interaction between NPs, HMs, and plant physiology [144]. This ambiguity may make it more difficult to develop NPs formulations that work well for agricultural applications. The efficacy of NPs can be affected with their interaction with soil and environmental conditions. Factors including soil chemistry, pH and organic matter can change NPs behavior, result in different outcomes in various soil types [23]. In the food chain, concerns exist with respect to metal-based NPs bioaccumulation. While NPs can assist plants in tolerating HMs, their own potential toxicity and the risk of entering the food supply necessitate careful evaluation to ensure food safety [41]. The damage can be lessened by creating substitute, eco-friendly NPs, but it is crucial to fund studies on remediation strategies. Advanced remediation techniques integrate biological and nanotechnological remediation techniques, whereby the manipulation of nanoscale processes facilitates the adsorption and degradation of contaminants.
10 Conclusions and Future Perspectives
Heavy metal contamination of agricultural soils has recently been a difficult problem for global food security. Nano-remediation strategies, including various metal-based NPs applications, are promising solutions to enhance plant growth and reduce metal uptake. The review highlighted how green synthesized NPs increased agricultural crop yields in several ways, with significant improvements shown in plant height and chlorophyll content, as well as reduced oxidative stress, raising specific enzyme activity under metal stress. Due to their lower cost, eco-effectiveness, and increased plant tolerance to a variety of biotic and abiotic stimuli, it is anticipated that their usage in agriculture will continue to increase. In TEs condition, NPs application at their best treatment level enhances the plant antioxidant defense system, ameliorating the structural changes, immobilizing TE in growth media, and improving the physio-chemical properties of soil as well. There is a significant research gap in understanding the molecular mechanisms underlying the interaction between green-synthesized NPs and plants, hindering the optimization of strategies for mitigating metal stress and enhancing plant growth. While existing studies show positive effects, a comprehensive understanding of the specific physiological processes activated by NPs is lacking. Investigating these mechanisms is crucial for establishing a more robust foundation for the practical application of green-synthesized NPs in sustainable agriculture. For this, it is recommended to promote and fund long-term research to assess the long-term effects of using NPs on plant development, soil health, and possible environmental repercussions.
Acknowledgement: The authors would like to extend their sincere appreciation to the financial support from the Government College University, Faisalabad, as well as Researchers Supporting Project at King Faisal University, Saudi Arabia (Project No. GRANTA513), for their invaluable support, which has significantly contributed to the development of this review paper.
Funding Statement: This work has been financially supported by the Deanship of Scientific Research, Vice Presidency for Graduate Studies and Scientific Research, King Faisal University, Saudi Arabia (Project No. GRANTA513).
Author Contributions: Abdula Ghafoor: Project administration, Supervision, Maria Latif: Conceptualization, Writing—review & editing, Shafaqat Ali: Methodology, Writing—review & editing, Muhammad Munir: Writing, Manuscript drafting, Muhammad Naeem Sattar: Review, Editing, Mohammed Ali Alshehri: Draft manuscript preparation, Editing. All authors reviewed the results and approved the final version of the manuscript.
Availability of Data and Materials: Data sharing is not applicable to this article as no datasets were analyzed during the current study.
Ethics Approval: Not applicable.
Conflicts of Interest: The authors declare that they have no known competing financial interests or personal relationships that could have appeared to influence the work reported in this paper.
References
1. Jayakumar M, Surendran U, Raja P, Kumar A, Senapathi V. A review of heavy metals accumulation pathways, sources and management in soils. Arab J Geosci. 2021;14(20):1–19. doi:10.1007/s12517-021-08543-9. [Google Scholar] [CrossRef]
2. Ahmad W, Alharthy RD, Zubair M, Ahmed M, Hameed A, Rafique S. Toxic and heavy metals contamination assessment in soil and water to evaluate human health risk. Sci Rep. 2021;11(1):1–12. doi:10.1038/s41598-021-94616-4. [Google Scholar] [PubMed] [CrossRef]
3. Sodhi KK, Mishra LC, Singh CK, Kumar M. Perspective on the heavy metal pollution and recent remediation strategies. Microbiol Curr Res. 2022;3(1):100166. doi:10.1016/j.crmicr.2022.100166. [Google Scholar] [PubMed] [CrossRef]
4. Jin M, Yuan H, Liu B, Peng J, Xu L, Yang D. Review of the distribution and detection methods of heavy metals in the environment. Anal Methods. 2020;12(48):5747–66. doi:10.1039/D0AY01577F. [Google Scholar] [PubMed] [CrossRef]
5. Manisalidis I, Stavropoulou E, Stavropoulos A, Bezirtzoglou E. Environmental and health impacts of air pollution: a review. Front Public Health. 2020;14:43. doi:10.3389/fpubh.2020.00014. [Google Scholar] [PubMed] [CrossRef]
6. Inyinbor AA, Adebesin Babatunde O, Oluyori Abimbola P, Adelani Akande Tabitha A, Dada Adewumi O, Oreofe Toyin A. Water pollution: effects, prevention, and climatic impact. Water Chall Urban World. 2018;33:33–47. [Google Scholar]
7. Xie R, Sun D, Sun L, Zhang N, Zhang J, Zang S. Sediment record of heavy metals over the last 150 years in Northeast China: implications for regional anthropogenic activities. Ecotoxicol. 2021;30(7):1354–65. doi:10.1007/s10646-021-02350-4. [Google Scholar] [PubMed] [CrossRef]
8. Latif M, Nasir N, Nawaz R, Nasim I, Sultan K, Irshad MA, et al. Assessment of drinking water quality using water quality index and synthetic pollution index in urban areas of mega city Lahore: a GIS-based approach. Sci Rep. 2024;14(1):13416. doi:10.1038/s41598-024-63296-1. [Google Scholar] [PubMed] [CrossRef]
9. Rizwan M, Ali S, Ali B, Adrees M, Arshad M, Hussain A, et al. Zinc and iron oxide nanoparticles improved the plant growth and reduced the oxidative stress and cadmium concentration in wheat. Chemosphere. 2019;214:269–77. doi:10.1016/j.chemosphere.2018.09.120. [Google Scholar] [PubMed] [CrossRef]
10. Kaninga BK, Chishala BH, Maseka KK, Sakala GM, Lark MR, Tye A. Mine tailings in an African tropical environment—mechanisms for the bioavailability of heavy metals in soils. Environ Geochem Health. 2020;42(4):1069–94. doi:10.1007/s10653-019-00326-2. [Google Scholar] [PubMed] [CrossRef]
11. Rashid A, Schutte BJ, Ulery A, Deyholos MK, Sanogo S, Lehnhoff EA. Heavy metal contamination in agricultural soil: environmental pollutants affecting crop health. Agronomy. 2023;13(6):1521. doi:10.3390/agronomy13061521. [Google Scholar] [CrossRef]
12. Manwani S, Vanisree CR, Jaiman V, Awasthi KK, Yadav CS, Sankhla MS, et al. Heavy metal contamination in vegetables and their toxic effects on human health. J Sustain Crop Prod. 2022;181. [Google Scholar]
13. Zhang Z, Cui Q, Chen L, Zhu X, Zhao S, Duan C. A critical review of microplastics in the soil-plant system: distribution, uptake, phytotoxicity and prevention. J Hazard Mater. 2022;424:127750. doi:10.1016/j.jhazmat.2021.127750. [Google Scholar] [PubMed] [CrossRef]
14. Miras-Moreno B, Senizza B, Regni L, Tolisano C, Proietti P, Trevisan M, et al. Biochemical insights into the ability of Lemna minor L. extract to counteract copper toxicity in maize. Plants. 2022;11(19):2613. doi:10.3390/plants11192613. [Google Scholar] [PubMed] [CrossRef]
15. Hussain I, Afzal S, Ashraf MA, Rasheed R, Saleem MH, Alatawi A. Effect of metals or trace elements on wheat growth and its remediation in contaminated soil. J Plant Growth Regul. 2023;42(4):2258–82. doi:10.1007/s00344-022-10700-7. [Google Scholar] [CrossRef]
16. Malik A, Mor VS, Punia H, Duhan DS, Bhuker A, Tokas J. Investigating mineral accumulation and seed vigor potential in bottle gourd (Lagenaria siceraria) through crossbreeding timing. Plants. 2023;12(23):3998. doi:10.3390/plants12233998. [Google Scholar] [PubMed] [CrossRef]
17. Hasan MK, Shopan J, Ahammed GJ. Nanomaterials and soil health for agricultural crop production: current status and future prospects. Nanomater Agric For Appl. 2020:289–312. [Google Scholar]
18. Shang Y, Hasan MK, Ahammed GJ, Li M, Yin H, Zhou J. Applications of nanotechnology in plant growth and crop protection: a review. Molecules. 2019;24(14):2558. doi:10.3390/molecules24142558. [Google Scholar] [PubMed] [CrossRef]
19. Latif A, Sheng D, Sun K, Si Y, Azeem M, Abbas A, et al. Remediation of heavy metals polluted environment using Fe-based nanoparticles: mechanisms, influencing factors, and environmental implications. Environ Pollut. 2020;264:114728. doi:10.1016/j.envpol.2020.114728. [Google Scholar] [PubMed] [CrossRef]
20. Hong J, Wang C, Wagner DC, Gardea-Torresdey JL, He F, Rico CM. Foliar application of nanoparticles: mechanisms of absorption, transfer, and multiple impacts. Environ Sci: Nano. 2021;8(5):1196–210. [Google Scholar]
21. Hassanisaadi M, Barani M, Rahdar A, Heidary M, Thysiadou A, Kyzas GZ. Role of agrochemical-based nanomaterials in plants: biotic and abiotic stress with germination improvement of seeds. J Plant Growth Regul. 2022;97(2):375–418. doi:10.1007/s10725-021-00782-w. [Google Scholar] [CrossRef]
22. Rizwan M, Ali S, ur Rehman MZ, Malik S, Adrees M, Qayyum MF, et al. Effect of foliar applications of silicon and titanium dioxide nanoparticles on growth, oxidative stress, and cadmium accumulation by rice (Oryza sativa). Acta Physiol Plant. 2019;41(3):1–12. [Google Scholar]
23. Zhou P, Adeel M, Shakoor N, Guo M, Hao Y, Azeem I, et al. Application of nanoparticles alleviates heavy metals stress and promotes plant growth: an overview. Nanomater. 2020;11(1):26. doi:10.3390/nano11010026. [Google Scholar] [PubMed] [CrossRef]
24. Chawla S, Kerna NA, Flores JV, Ngwu DC, Carsrud NDV, Holets HM. Environmental heavy metals: adverse effects on the human skeletal system. EC Orthop. 2023;14:01–17. [Google Scholar]
25. Goswami R, Neog N. Heavy metal pollution in the environment: impact on air quality and human health implications. In: Heavy metal toxicity: Environmental concerns, remediation and opportunities. Singapore: Springer Nature Singapore; 2023. p. 75–103. [Google Scholar]
26. Luo X, Wu C, Lin Y, Li W, Deng M, Tan J, et al. Soil heavy metal pollution from Pb/Zn smelting regions in China and the remediation potential of biomineralization. J Environ Sci. 2023;125:662–77. doi:10.1016/j.jes.2022.01.029. [Google Scholar] [PubMed] [CrossRef]
27. Jeong H, Choi JY, Ra K. Heavy metal pollution assessment in stream sediments from urban and different types of industrial areas in South Korea. Soil Sediment Contam: Int J. 2021;30(7):804–18. doi:10.1080/15320383.2021.1893646. [Google Scholar] [CrossRef]
28. Pujari M, Kapoor D. Heavy metals in the ecosystem: sources and their effects. In: Heavy metals in the environment. Amsterdam, The Netherlands: Elsevier; 2021. p. 1–7. [Google Scholar]
29. Ali H, Khan E, Ilahi I. Environmental chemistry and ecotoxicology of hazardous heavy metals: environmental persistence, toxicity, and bioaccumulation. J Chem. 2019;2019(1):6730305. [Google Scholar]
30. Said I. Nickel pollution pathways in small ecosystem. Egypt Arab J Geosci. 2022;15(10):1–8. [Google Scholar]
31. Alengebawy A, Abdelkhalek ST, Qureshi SR, Wang MQ. Heavy metals and pesticides toxicity in agricultural soil and plants: ecological risks and human health implications. Toxics. 2021;9(3):42. doi:10.3390/toxics9030042. [Google Scholar] [PubMed] [CrossRef]
32. Al-Dhabi NA, Esmail GA, Mohammed Ghilan AK, Valan Arasu M. Composting of vegetable waste using microbial consortium and biocontrol efficacy of Streptomyces sp. isolated from the Saudi Arabian environment for sustainable agriculture. Sustainability. 2019;11(23):6845. doi:10.3390/su11236845. [Google Scholar] [CrossRef]
33. Alghobar MA, Suresha S. Evaluation of metal accumulation in soil and tomatoes irrigated with sewage water from Mysore city, Karnataka, India. J Saudi Soc Agric Sci. 2017;16(1):49–59. [Google Scholar]
34. Ashraf I, Ahmad F, Sharif A, Altaf AR, Teng H. Heavy metals assessment in water, soil, vegetables, and their associated health risks via consumption of vegetables, District Kasur, Pakistan. SN Appl Sci. 2021;3(5):1–16. [Google Scholar]
35. Atale N, Saxena S, Nirmala JG, Narendhirakannan RT, Mohanty S, Rani V. Synthesis and characterization of Sygyzium cumini nanoparticles for their protective potential in high glucose-induced cardiac stress: a green approach. Appl Biochem Biotechnol. 2017;181(3):1140–54. doi:10.1007/s12010-016-2274-6. [Google Scholar] [PubMed] [CrossRef]
36. Tuga B, O’Keefe T, Deng C, Ligocki AT, White JC, Haynes CL. Designing nanoparticles for sustainable agricultural applications. Trends Chem. 2023;5(11):814–826 doi:10.1016/j.trechm.2023.07.004. [Google Scholar] [CrossRef]
37. Trukhanov AV, Thakur P, Thakur A. Nanotechnological applications in food and agriculture. In: Integrated nanomaterials and their applications. Singapore: Springer Nature Singapore; 2023. p. 393–417. [Google Scholar]
38. Charagh S, Hui S, Wang J, Raza A, Zhou L, Xu B, et al. Unveiling innovative approaches to mitigate metals/metalloids toxicity for sustainable agriculture. Physiol Plant. 2024;176(2):e14226. doi:10.1111/ppl.v176.2. [Google Scholar] [CrossRef]
39. Alhaithloul HAS, Ali B, Alghanem SMS, Zulfiqar F, Al-Robai SA, Ercisli S. Effect of green-synthesized copper oxide nanoparticles on growth, physiology, nutrient uptake, and cadmium accumulation in Triticum aestivum (L.). Ecotoxicol Environ Saf. 2023;268:115701. doi:10.1016/j.ecoenv.2023.115701. [Google Scholar] [PubMed] [CrossRef]
40. Latif M, Ali S, Ansari MA, Zahoor AF, Nafees M. Exploring the potential of green synthesized cerium oxide nanoparticles in mitigating chromium toxicity in maize. J King Saud Univ Sci. 2024;36(8):103323. doi:10.1016/j.jksus.2024.103323. [Google Scholar] [CrossRef]
41. Sarraf M, Vishwakarma K, Kumar V, Arif N, Das S, Johnson R, et al. Metal/metalloid-based nanomaterials for plant abiotic stress tolerance: an overview of the mechanisms. Plants. 2022;11(3):316. doi:10.3390/plants11030316. [Google Scholar] [PubMed] [CrossRef]
42. Chen T, Chang QR, Liu J, Clevers JGPW, Kooistra L. Identification of soil heavy metal sources and improvement in spatial mapping based on soil spectral information: a case study in northwest China. Sci Total Environ. 2016;565:155–64. doi:10.1016/j.scitotenv.2016.04.163. [Google Scholar] [PubMed] [CrossRef]
43. Alabdallah NM, Al-Shammari AS, Saleem K, AlZahrani SS, Raza A, Asghar MA, et al. Unveiling the mechanisms of silicon-induced salinity stress tolerance in Panicum turgidum: insights from antioxidant defense system and comprehensive metabolic and nutritional profiling. S Afr J Bot. 2024;168:328–39. doi:10.1016/j.sajb.2024.03.006. [Google Scholar] [CrossRef]
44. Rossi L, Zhang W, Ma X. Cerium oxide nanoparticles alter the salt stress tolerance of Brassica napus L. by modifying the formation of root apoplastic barriers. Environ Pollut. 2016;229:132–8. [Google Scholar]
45. Adisa IO, Pullagurala VLR, Peralta-Videa JR, Dimkpa CO, Elmer WH, Gardea-Torresdey JL, et al. Recent advances in nano-enabled fertilizers and pesticides: a critical review of mechanisms of action. Environ Sci Nano. 2019;6(7):2002–30. doi:10.1039/C9EN00265K. [Google Scholar] [CrossRef]
46. Velsankar K, Suganya S, Muthumari P, Mohandoss S, Sudhahar S. Ecofriendly green synthesis, characterization and biomedical applications of CuO nanoparticles synthesized using leaf extract of Capsicum frutescens. J Environ Chem Eng. 2021;9(5):106299. doi:10.1016/j.jece.2021.106299. [Google Scholar] [CrossRef]
47. Hosseini-Koupaei M, Shareghi B, Saboury AA, Davar F, Sirotkin VA, Hosseini-Koupaei MH, et al. Catalytic activity, structure and stability of proteinase K in the presence of biosynthesized CuO nanoparticles. Int J Biol Macromol. 2019;122:732–44. doi:10.1016/j.ijbiomac.2018.11.001. [Google Scholar] [PubMed] [CrossRef]
48. Samuel MS, Jose S, Selvarajan E, Mathimani T, Pugazhendhi A. Biosynthesized silver nanoparticles using Bacillus amyloliquefaciens; Application for cytotoxicity effect on A549 cell line and photocatalytic degradation of p-nitrophenol. J Photochem Photobiol B. 2020;202:111642. doi:10.1016/j.jphotobiol.2019.111642. [Google Scholar] [PubMed] [CrossRef]
49. Abdelghany TM, Al-Rajhi AM, Al Abboud MA, Alawlaqi MM, Ganash Magdah A, Helmy EA, et al. Recent advances in green synthesis of silver nanoparticles and their applications: about future directions. A review. Bio Nano Sci. 2018;8(1):5–16. [Google Scholar]
50. Gour A, Jain NK. Advances in green synthesis of nanoparticles. Artif Cells Nanomed Biotechnol. 2019;47(1):844–51. doi:10.1080/21691401.2019.1577878. [Google Scholar] [PubMed] [CrossRef]
51. Abid N, Khan AM, Shujait S, Chaudhary K, Ikram M, Imran M, et al. Synthesis of nanomaterials using various top-down and bottom-up approaches, influencing factors, advantages, and disadvantages: a review. Adv Colloid Interface Sci. 2022;300:102597. doi:10.1016/j.cis.2021.102597. [Google Scholar] [PubMed] [CrossRef]
52. Dang-Bao T, Favier I, Gómez M. Metal nanoparticles in polyols: bottom-up and top-down syntheses and catalytic applications. In: Nanoparticles in catalysis: advances in synthesis and applications; 2021. vol. 5, p. 99–122. [Google Scholar]
53. Krishna RH, Chandraprabha MN, Monika P, Br T, Chaudhary V, Manjunatha C. Biomolecule conjugated inorganic nanoparticles for biomedical applications: a review. Biotechnol Genet Eng. 2022:40(4):3611–52. [Google Scholar]
54. Shrimal P, Jadeja G, Patel S. A review on novel methodologies for drug nanoparticle preparation: microfluidic approach. Chem Eng Res Des. 2020;153:728–56. doi:10.1016/j.cherd.2019.11.031. [Google Scholar] [CrossRef]
55. Indiarto R, Indriana LPA, Andoyo R, Subroto E, Nurhadi B. Bottom-up nanoparticle synthesis: a review of techniques, polyphenol-based core materials, and their properties. Eur Food Res Technol. 2022;248(1):1–24. doi:10.1007/s00217-021-03867-y. [Google Scholar] [CrossRef]
56. Rana A, Yadav K, Jagadevan S. A comprehensive review on green synthesis of nature-inspired metal nanoparticles: mechanism, application and toxicity. J Clean Prod. 2020;272:122880. doi:10.1016/j.jclepro.2020.122880. [Google Scholar] [CrossRef]
57. Tran TV, Nguyen D.T. C, Kumar PS, Din A.T. M, Jalil AA, Vo DVN. Green synthesis of ZrO2 nanoparticles and nanocomposites for biomedical and environmental applications: a review. Environ Chem Lett. 2022;20:1309–31. doi:10.1007/s10311-021-01367-9. [Google Scholar] [PubMed] [CrossRef]
58. Sarip NA, Aminudin NI, Danial WH. Green synthesis of metal nanoparticles using Garcinia extracts: a review. Environ Chem Lett. 2022:20(1):469–93. doi:10.1007/s10311-021-01319-3. [Google Scholar] [CrossRef]
59. Patil MP, Bayaraa E, Subedi P, Piad LLA, Tarte NH, Kim GD. Biogenic synthesis, characterization of gold nanoparticles using Lonicera japonica and their anticancer activity on HeLa cells. J Drug Deliv Sci Technol. 2019;51:83–90. doi:10.1016/j.jddst.2019.02.021. [Google Scholar] [CrossRef]
60. Azmi L, Reddy DS, Pal S. Plant-derived synthesis of bionanomaterials. In: Synthesis of bionanomaterials for biomedical applications. Amsterdam, The Netherlands: Elsevier; 2023. p. 131–50. [Google Scholar]
61. Ghidan AY, Al-Antary TM, Awwad AM. Green synthesis of copper oxide nanoparticles using Punica granatum peels extract: effect on green peach Aphid. Environ Nanotechnol Monit Manag. 2016;6:95–8. [Google Scholar]
62. Sharma BK, Shah DV, Roy DR. Green synthesis of CuO nanoparticles using Azadirachta indica and its antibacterial activity for medicinal applications. Mater Res Express. 2018;5(9):095033. doi:10.1088/2053-1591/aad91d. [Google Scholar] [CrossRef]
63. Goutam SP, Sharma KK, Chauhan A, Bajpai D, Verma S, Singh G. Synthesis of copper oxide nanoparticles using green leaves extract of azadirachta indica. Invertis J Renew Energy. 2020;10(1):7. doi:10.5958/2454-7611.2020.00002.8. [Google Scholar] [CrossRef]
64. Ijaz F, Shahid S, Khan SA, Ahmad W, Zaman S. Green synthesis of copper oxide nanoparticles using Abutilon indicum leaf extract: antimicrobial, antioxidant and photocatalytic dye degradation activities. Trop J Pharm Res. 2017;16(4):743–53. doi:10.4314/tjpr.v16i4.2. [Google Scholar] [CrossRef]
65. Bordbar M, Sharifi-Zarchi Z, Khodadadi B. Green synthesis of copper oxide nanoparticles/clinoptilolite using Rheum palmatum L. root extract: high catalytic activity for reduction of 4-nitro phenol, rhodamine B, and methylene blue. J. Sol-Gel Sci Technol. 2017;81(3):724–33. doi:10.1007/s10971-016-4239-1. [Google Scholar] [CrossRef]
66. Shubhashree KR, Reddy R, Gangula AK, Nagananda GS, Badiya PK, Ramamurthy SS, et al. Green synthesis of copper nanoparticles using aqueous extracts from Hyptis suaveolens (L.). Mater Chem Phys. 2022;280:125795. doi:10.1016/j.matchemphys.2022.125795. [Google Scholar] [CrossRef]
67. Muthamil SS, Vijai AK, Govindaraju K, Tamilselvan S, Kumar VG, Subramanian KS, et al. Green synthesis of copper oxide nanoparticles and mosquito larvicidal activity against dengue, zika and chikungunya causing vector Aedes aegypti. IET Nanobiotechnology. 2018;12(8):1042–6. doi:10.1049/nbt2.v12.8. [Google Scholar] [CrossRef]
68. Pramanik P, Krishnan P, Maity A, Mridha N, Mukherjee A, Rai V. Application of nanotechnology in agriculture. In: Environ nanotechnol. Cham: Springer; 2020. vol. 4, p. 317–48. [Google Scholar]
69. Hussain A, Ali S, Rizwan M, ur Rehman MZ, Hameed A, Hafeez F, et al. Role of zinc-lysine on growth and chromium uptake in rice plants under Cr stress. J Plant Growth Regul. 2018;37(4):1413–22. doi:10.1007/s00344-018-9831-x. [Google Scholar] [CrossRef]
70. Cao Z, Rossi L, Stowers C, Zhang W, Lombardini L, Ma X. The impact of cerium oxide nanoparticles on the physiology of soybean (Glycine max (L.) Merr.) under different soil moisture conditions. Environ Sci Pollut Res. 2018;25(1):930–9. doi:10.1007/s11356-017-0501-5. [Google Scholar] [PubMed] [CrossRef]
71. Liu R, Lal R. Potentials of engineered nanoparticles as fertilizers for increasing agronomic productions. Sci Total Environ. 2015;514:131–9. doi:10.1016/j.scitotenv.2015.01.104. [Google Scholar] [PubMed] [CrossRef]
72. Wojcieszek J, Jiménez-Lamana J, Bierła K, Ruzik L, Asztemborska M, Jarosz M, et al. Uptake, translocation, size characterization and localization of cerium oxide nanoparticles in radish (Raphanus sativus L.). Sci Total Environ. 2019;683:284–92. doi:10.1016/j.scitotenv.2019.05.265. [Google Scholar] [PubMed] [CrossRef]
73. Ma X, Yan J. Plant uptake and accumulation of engineered metallic nanoparticles from lab to field conditions. Curr Opin Environ Sci Health. 2018;6:16–20. doi:10.1016/j.coesh.2018.07.008. [Google Scholar] [CrossRef]
74. Rico CM, Abolade OM, Wagner D, Lottes B, Rodriguez J, Biagioni R, et al. Wheat exposure to cerium oxide nanoparticles over three generations reveals transmissible changes in nutrition, biochemical pools, and response to soil N. J Hazard Mater. 2020;384:121364. doi:10.1016/j.jhazmat.2019.121364. [Google Scholar] [PubMed] [CrossRef]
75. Wang X, Sun W, Zhang S, Sharifan H, Ma X. Elucidating the effects of cerium oxide nanoparticles and zinc oxide nanoparticles on arsenic uptake and speciation in rice (Oryza sativa) in a hydroponic system. Environ Sci Technol. 2018;52(17):10040–47. doi:10.1021/acs.est.8b01664. [Google Scholar] [PubMed] [CrossRef]
76. Marchiol L, Mattiello A, Pošćić F, Fellet G, Zavalloni C, Carlino E, et al. Changes in physiological and agronomical parameters of barley (Hordeum vulgare) exposed to cerium and titanium dioxide nanoparticles. Int J Environ Res Public Health. 2016;13(3):332. doi:10.3390/ijerph13030332. [Google Scholar] [PubMed] [CrossRef]
77. Amde M, Liu JF, Tan ZQ, Bekana D. Transformation and bioavailability of metal oxide nanoparticles in aquatic and terrestrial environments. a review. Environ Pollut. 2017;230:250–67. doi:10.1016/j.envpol.2017.06.064. [Google Scholar] [PubMed] [CrossRef]
78. O.’Keefe TL, Haynes CL. Review of oxidative dissolution and sulfidation of select nanoparticles in the environment: impact on applications. ACS Appl Nano Mater. 2024;7(8):8392–406. doi:10.1021/acsanm.3c06227. [Google Scholar] [CrossRef]
79. Keller AA, Zheng Y, Praetorius A, Quik JT, Nowack B. Predicting environmental concentrations of nanomaterials for exposure assessment—a review. NanoImpact. 2024;33:100496. doi:10.1016/j.impact.2024.100496. [Google Scholar] [PubMed] [CrossRef]
80. Rahman SU, Wang X, Shahzad M, Bashir O, Li Y, Cheng H. A review of the influence of nanoparticles on the physiological and biochemical attributes of plants with a focus on the absorption and translocation of toxic trace elements. Environ Pollut. 2022;310:119916. doi:10.1016/j.envpol.2022.119916. [Google Scholar] [PubMed] [CrossRef]
81. Khepar V, Sidhu A, Chandel S. Sustained release of Zn from zinc sulfide nanoparticles (ZnS NPs) amplified the bioaccessibility of Zn in soil: adsorption dynamics and dissolution kinetics. Environ Res. 2024;251:118624. doi:10.1016/j.envres.2024.118624. [Google Scholar] [PubMed] [CrossRef]
82. Kumar A, Singh IK, Mishra R, Singh A, Ramawat N, Singh A. The role of zinc oxide nanoparticles in plants: a critical appraisal. In: Nanomaterial biointeractions at the cellular, organismal and system levels. Cham: Springer; 2021. p. 249–67. [Google Scholar]
83. Maswada HF, Djanaguiraman M, Prasad PVV. Seed treatment with nano-iron (III) oxide enhances germination, seeding growth and salinity tolerance of sorghum. J Agron Crop Sci. 2018;204(6):577–87. doi:10.1111/jac.2018.204.issue-6. [Google Scholar] [CrossRef]
84. Parveen A, Siddiqui ZA. Foliar spray and seed priming of titanium dioxide nanoparticles and their impact on the growth of tomato, defense enzymes and some bacterial and fungal diseases. Arch Phytopathol Plant Prot. 2022;55(5):527–48. doi:10.1080/03235408.2022.2035535. [Google Scholar] [CrossRef]
85. Khan I, Awan SA, Rizwan M, Hassan ZU, Akram MA, Tariq R, et al. Nanoparticle’s uptake and translocation mechanisms in plants via seed priming, foliar treatment, and root exposure: a review. Environ Sci Pollut Res. 2022;29(60):89823–33. doi:10.1007/s11356-022-23945-2. [Google Scholar] [PubMed] [CrossRef]
86. Abdel-Aziz HMM, Hasaneen MNA, Omer AM. Impact of engineered nanomaterials either alone or loaded with NPK on growth and productivity of French bean plants: seed priming vs foliar application. S Afr J Bot. 2019;125:102–8. doi:10.1016/j.sajb.2019.07.005. [Google Scholar] [CrossRef]
87. Raj NP, Chandrashekara CP. Nano zinc seed treatment and foliar application on growth, yield and economics of Bt cotton (Gossypium hirsutum L.). Int J Curr Microbiol Appl Sci. 2019;8(8):1624–30. doi:10.20546/ijcmas. [Google Scholar] [CrossRef]
88. Yadav M, George N, Dwibedi V. Emergence of toxic trace elements in plant environments: insights into the potential of silica nanoparticles for mitigating metal toxicity in plants. Environ Pollut. 2023;333:122112. doi:10.1016/j.envpol.2023.122112. [Google Scholar] [PubMed] [CrossRef]
89. Zandalinas SI, Peláez-Vico MÁ, Sinha R, Pascual LS, Mittler R. The impact of multifactorial stress combination on plants, crops, and ecosystems: how should we prepare for what comes next? Plant J. 2024;117(6):1800–14. doi:10.1111/tpj.v117.6. [Google Scholar] [CrossRef]
90. Sethi S. Phytochelatins: heavy metal detoxifiers in plants. In: Advanced and innovative approaches of environmental biotechnology in industrial wastewater treatment. Singapore: Springer Nature Singapore; 2023. p. 361–79. [Google Scholar]
91. Mathur P, Tripathi DK, Baluška F, Mukherjee S. Auxin-mediated molecular mechanisms of heavy metal and metalloid stress regulation in plants. Environ Exp Bot. 2022;196:104796. doi:10.1016/j.envexpbot.2022.104796. [Google Scholar] [CrossRef]
92. El-Saadony MT, Saad AM, Soliman SM, Salem HM, Desoky ESM, Babalghith AO. Role of nanoparticles in enhancing crop tolerance to abiotic stress: a comprehensive review. Front Plant Sci. 2022;13:946717. doi:10.3389/fpls.2022.946717. [Google Scholar] [PubMed] [CrossRef]
93. Kumari A, Rana V, Yadav SK, Kumar V. Nanotechnology as a powerful tool in plant sciences: recent developments, challenges and perspectives. Plant Nano Biology. 2023;5:100046. doi:10.1016/j.plana.2023.100046. [Google Scholar] [CrossRef]
94. Gomez R, Vicino P, Carrillo N, Lodeyro AF. Manipulation of oxidative stress responses as a strategy to generate stress-tolerant crops. From damage to signaling to tolerance. Crit Rev Biotechnol. 2019;39(5):693–708. doi:10.1080/07388551.2019.1597829. [Google Scholar] [PubMed] [CrossRef]
95. Petrov V, Hille J, Mueller-Roeber B, Gechev TS. ROS-mediated abiotic stress-induced programmed cell death in plants. Front Plant Sci. 2015;6:69. [Google Scholar] [PubMed]
96. Mhamdi A, Van Breusegem F. Reactive oxygen species in plant development. J Dev. 2018;145(15):dev164376. doi:10.1242/dev.164376. [Google Scholar] [PubMed] [CrossRef]
97. Kerchev P, van der Meer T, Sujeeth N, Verlee A, Stevens CV, Van Breusegem F, et al. Molecular priming as an approach to induce tolerance against abiotic and oxidative stresses in crop plants. Biotechnol Adv. 2020;40:107503. doi:10.1016/j.biotechadv.2019.107503. [Google Scholar] [PubMed] [CrossRef]
98. Wu F, Fang Q, Yan S, Pan L, Tang X, Ye W. Effects of zinc oxide nanoparticles on arsenic stress in rice (Oryza sativa L.germination, early growth, and arsenic uptake. Environ Sci Pollut Res. 2020;27(21):26974–81. doi:10.1007/s11356-020-08965-0. [Google Scholar] [PubMed] [CrossRef]
99. Yan L, Li P, Zhao X, Ji R, Zhao L. Physiological and metabolic responses of maize (Zea mays) plants to Fe3O4 nanoparticles. Sci Total Environ. 2020;718:137400. doi:10.1016/j.scitotenv.2020.137400. [Google Scholar] [PubMed] [CrossRef]
100. Abbasifar A, Shahrabadi F, ValizadehKaji B. Effects of green synthesized zinc and copper nano-fertilizers on the morphological and biochemical attributes of basil plant. J Plant Nutr. 2020;43(8):1104–18. doi:10.1080/01904167.2020.1724305. [Google Scholar] [CrossRef]
101. Jalil S, Nazir MM, Al-Huqail AA, Ali B, Al-Qthanin RN, Asad MA. Silicon nanoparticles alleviate cadmium toxicity in rice (Oryza sativa L.) by modulating the nutritional profile and triggering stress-responsive genetic mechanisms. Ecotoxicol Environ Saf. 2023;268:115699. doi:10.1016/j.ecoenv.2023.115699. [Google Scholar] [PubMed] [CrossRef]
102. Li J, Shi C, Wang X, Liu C, Ding X, Ma P, et al. Hydrogen sulfide regulates the activity of antioxidant enzymes through per-sulfidation and improves the resistance of tomato seedling to copper oxide nanoparticles (CuO NPs)-induced oxidative stress. J Physiol Biochem. 2020;156:257–66. [Google Scholar]
103. Rizwan M, Ali S, Rehman MZ, Rinklebe U, Tsang J, Tack DC, et al. Effects of selenium on the uptake of toxic trace elements by crop plants: a review. Crit Rev Environ Sci Technol. 2021;51(21):2531–66. doi:10.1080/10643389.2020.1796566. [Google Scholar] [CrossRef]
104. Rizwan M, Ali S, ur Rehman MZ, Riaz M, Adrees M, Hussain A, et al. Effects of nanoparticles on trace element uptake and toxicity in plants: a review. Ecotoxicol Environ Saf. 2021;221:112437. doi:10.1016/j.ecoenv.2021.112437. [Google Scholar] [PubMed] [CrossRef]
105. Raveau R, Fontaine J, Hijri M, Lounes-Hadj Sahraoui A. The aromatic plant clary sage shaped bacterial communities in the roots and in the trace element-contaminated soil more than mycorrhizal inoculation—a two-year monitoring field trial. Front Microbiol. 2020;11:586050. doi:10.3389/fmicb.2020.586050. [Google Scholar] [PubMed] [CrossRef]
106. Pérez Velasco EA, Betancourt GR, Valdez ALA, González FJA, Puente UBA, Lozano MSA, et al. Effects of the morphology, surface modification and application methods of ZnO-NPs on the growth and biomass of tomato plants. Molecules. 2020;25(6):1282. doi:10.3390/molecules25061282. [Google Scholar] [PubMed] [CrossRef]
107. Maraei RW, Louis YA, Safwat G. Assessment of irradiated TiO2 nanoparticles on the growth and nutritional components of broccoli. Not Bot Horti Agrobo. 2021;49(3):12397. doi:10.15835/nbha49312397. [Google Scholar] [CrossRef]
108. Hussain S, Shafiq I, Chattha MS, Mumtaz M, Brestic M, Rastogi A, et al. Effect of Ti treatments on growth, photosynthesis, phosphorus uptake and yield of soybean (Glycine max L.) in maize-soybean relay strip intercropping. Environ Exp Bot. 2021;187:104476. doi:10.1016/j.envexpbot.2021.104476. [Google Scholar] [CrossRef]
109. Mustafa H, Ilyas N, Akhtar N, Raja NI, Zainab T, Shah T, et al. Biosynthesis and characterization of titanium dioxide nanoparticles and its effects along with calcium phosphate on physicochemical attributes of wheat under drought stress. Ecotoxicol Environ Saf. 2021;223:112519. doi:10.1016/j.ecoenv.2021.112519. [Google Scholar] [PubMed] [CrossRef]
110. Ahmed S, Iqbal M, Ahmad Z, Iqbal MA, Artyszak A, Sabagh AE. Foliar application of silicon-based nanoparticles improve the adaptability of maize (Zea mays L.) in cadmium contaminated soils. Environ Sci Pollut Res. 2023;30(14):41002–13. doi:10.1007/s11356-023-25189-0. [Google Scholar] [PubMed] [CrossRef]
111. Kiany T, Pishkar L, Sartipnia N, Iranbakhsh A, Barzin G. Effects of silicon and titanium dioxide nanoparticles on arsenic accumulation, phytochelatin metabolism, and antioxidant system by rice under arsenic toxicity. Environ Sci Pollut Res. 2022;29(23):34725–37. doi:10.1007/s11356-021-17927-z. [Google Scholar] [PubMed] [CrossRef]
112. Ma X, Sharifan H, Dou F, Sun W. Simultaneous reduction of arsenic (As) and cadmium (Cd) accumulation in rice by zinc oxide nanoparticles. Chem Eng J. 2020;384:123802. doi:10.1016/j.cej.2019.123802. [Google Scholar] [CrossRef]
113. Tripathi DK, Singh S, Singh S, Chauhan DK, Dubey NK, Prasad R. Silicon as a beneficial element to combat the adverse effect of drought in agricultural crops: capabilities and future possibilities. Water Stress Crop Plants: Sustain Approach. 2016;2:682–94. [Google Scholar]
114. Lian J, Zhao L, Wu J, Xiong H, Bao Y, Zeb A, et al. Foliar spray of TiO2 nanoparticles prevails over root application in reducing Cd accumulation and mitigating Cd-induced phytotoxicity in maize (Zea mays L.). Chemosphere. 2020;239:124794. doi:10.1016/j.chemosphere.2019.124794. [Google Scholar] [PubMed] [CrossRef]
115. Fox JP, Capen JD, Zhang W, Ma X, Rossi L. Effects of cerium oxide nanoparticles and cadmium on corn (Zea mays L.) seedlings physiology and root anatomy. Nano Impact. 2020;20:100264. [Google Scholar]
116. Daghan H. Effects of TiO2 nanoparticles on maize (Zea mays L.) growth, chlorophyll content and nutrient uptake. Appl Eco Environ Res. 2018;16. [Google Scholar]
117. Chen F, Bashir A, Zia ur Rehman M, Adrees M, Jing Ma, Ali S, et al. Combined effects of green manure and zinc oxide nanoparticles on cadmium uptake by wheat (Triticum aestivum L.). Chemosphere. 2022;298:134348. doi:10.1016/j.chemosphere.2022.134348. [Google Scholar] [PubMed] [CrossRef]
118. Guan X, Gao X, Avellan A, Spielman-Sun E, Xu J, Laughton S, et al. CuO nanoparticles alter the rhizospheric bacterial community and local nitrogen cycling for wheat grown in a calcareous soil. Environ Sci Tech. 2020;54(14):8699–709. doi:10.1021/acs.est.0c00036. [Google Scholar] [PubMed] [CrossRef]
119. Zakharova O, Kolesnikov E, Shatrova N, Gusev A. The effects of CuO nanoparticles on wheat seeds and seedlings and Alternaria solani fungi: in vitro study. IOP Conf Series: Earth Environ Sci. 2019;226:012036. [Google Scholar]
120. Ortega-Ortiz H, Gaucin-Delgado JM, Preciado-Rangel P, Fortis-Hernandez M, Hernandez-Montiel LG, La Cruz-lazaro ED, et al. Copper oxide nanoparticles biosynthetized improve germination and bioactive compounds in wheat sprouts. Not Bot Horti Agrobo. 2022;50(1):12657. doi:10.15835/nbha50112657. [Google Scholar] [CrossRef]
121. Petrova A, Plaksenkova I, Kokina I, Jermaļonoka M. Effect of Fe3O4 and CuO Nanoparticles on morphology, genotoxicity, and miRNA expression on different barley (Hordeum vulgare L.) genotypes. Sci World J. 2021;2021(1):6644689. [Google Scholar]
122. Huang G, Zuverza-Mena N, White JC, Hu H, Xing B, Dhankher OP. Simultaneous exposure of wheat (Triticum aestivum L.) to CuO and S nanoparticles alleviates toxicity by reducing Cu accumulation and modulating antioxidant response. Sci Total Environ. 2022;839:156285. doi:10.1016/j.scitotenv.2022.156285. [Google Scholar] [PubMed] [CrossRef]
123. Manzoor N, Ahmed T, Noman M, Shahid M, Nazir MM, Ali L, et al. Iron oxide nanoparticles ameliorated the cadmium and salinity stresses in wheat plants, facilitating photosynthetic pigments and restricting cadmium uptake. Sci Total Environ. 2021;769:145221. doi:10.1016/j.scitotenv.2021.145221. [Google Scholar] [PubMed] [CrossRef]
124. Rodrigues ES, Montanha GS, de Almeida E, Fantucci H, Santos RM, de Carvalho H W. Effect of nano cerium oxide on soybean (Glycine max L. Merrill) crop exposed to environmentally relevant concentrations. Chemosphere. 2021;273:128492. doi:10.1016/j.chemosphere.2020.128492. [Google Scholar] [PubMed] [CrossRef]
125. Zeeshan M, Hu YX, Iqbal A, Salam A, Liu YX, Muhammad I, et al. Amelioration of AsV toxicity by concurrent application of ZnO-NPs and Se-NPs is associated with differential regulation of photosynthetic indexes, antioxidant pool and osmolytes content in soybean seedling. Ecotoxicol Environ Saf. 2021;225:112738. doi:10.1016/j.ecoenv.2021.112738. [Google Scholar] [PubMed] [CrossRef]
126. Siddiqui ZA, Parveen A, Ahmad L, Hashem A. Effects of graphene oxide and zinc oxide nanoparticles on growth, chlorophyll, carotenoids, proline contents and diseases of carrot. Sci Hortic. 2019;249:374–82. doi:10.1016/j.scienta.2019.01.054. [Google Scholar] [CrossRef]
127. Fatemi H, Esmaiel Pour B, Rizwan M. Foliar application of silicon nanoparticles affected the growth, vitamin C, flavonoid, and antioxidant enzyme activities of coriander (Coriandrum sativum L.) plants grown in lead (Pb)-spiked soil. Environ Sci Pollut Res. 2021;28(2):1417–25. doi:10.1007/s11356-020-10549-x. [Google Scholar] [PubMed] [CrossRef]
128. Babashpour-Asl M, Farajzadeh-Memari-Tabrizi E, Yousefpour-Dokhanieh A. Foliar-applied selenium nanoparticles alleviate cadmium stress through changes in physio-biochemical status and essential oil profile of coriander (Coriandrum sativum L.) leaves. Environ Sci Pollut Res. 2022;29(53):80021–31. doi:10.1007/s11356-022-19941-1. [Google Scholar] [PubMed] [CrossRef]
129. Memari-Tabrizi EF, Yousefpour-Dokhanieh A, Babashpour-Asl M. Foliar-applied silicon nanoparticles mitigate cadmium stress through physio-chemical changes to improve growth, antioxidant capacity, and essential oil profile of summer savory (Satureja hortensis L.). Plant Physiol Biochem. 2021;165:71–9. doi:10.1016/j.plaphy.2021.04.040. [Google Scholar] [PubMed] [CrossRef]
130. B. DAS, Yonzone R, Saha S, Murmu DK, Kundu S. Comprehensive assessment of ZnO, P and TiO2 nanoparticles sustaining environment in response to seed germination, antioxidants activity, nutritional quality, and yield of Spinach Beet (Beta vulgaris var. bengalensis). Res Square. 2022;1–16. [Google Scholar]
131. Hassan UU, Khan M, Younas Z, Raja NI, Mashwani ZUR, Sohail. Effect of phytogenic iron nanoparticles on the bio-fortification of wheat varieties. Green Process Synth. 2023;12(1):20238002. doi:10.1515/gps-2023-8002. [Google Scholar] [CrossRef]
132. Khan N, Bano A. Exopolysaccharide producing rhizobacteria and their impact on growth and drought tolerance of wheat grown under rainfed conditions. PLoS One. 2019;14(9):e0222302. doi:10.1371/journal.pone.0222302. [Google Scholar] [PubMed] [CrossRef]
133. Ali S, Mehmood A, Khan N. Uptake, translocation, and consequences of nanomaterials on plant growth and stress adaptation. J Nanomater. 2021;1–17. [Google Scholar]
134. Baysal A, Saygın H. Effect of zinc oxide nanoparticles on the trace element contents of soils. Chem Eco. 2018;34(8):713–26. doi:10.1080/02757540.2018.1491556. [Google Scholar] [CrossRef]
135. Peng D, Wu B, Tan H, Hou S, Liu M, Tang H, et al. Effect of multiple iron-based nanoparticles on availability of lead and iron, and micro-ecology in lead contaminated soil. Chemosphere. 2019;228:44–53. doi:10.1016/j.chemosphere.2019.04.106. [Google Scholar] [PubMed] [CrossRef]
136. Rossi L, Zhang W, Schwab AP, Uptake Ma X. Accumulation, and in planta distribution of coexisting cerium oxide nanoparticles and cadmium in Glycine max (L.). Merr Environ Sci Tech. 2017;51(21):12815–24. doi:10.1021/acs.est.7b03363. [Google Scholar] [PubMed] [CrossRef]
137. Verma Y, Singh SK, Jatav HS, Rajput VD, Minkina T. Interaction of zinc oxide nanoparticles with soil: insights into the chemical and biological properties. Environ Geochem Health. 2022;44:221–34. doi:10.1007/s10653-021-00929-8. [Google Scholar] [PubMed] [CrossRef]
138. Zhang W, Long J, Li J, Zhang M, Ye X, Chang W, et al. Effect of metal oxide nanoparticles on the chemical speciation of heavy metals and micronutrient bioavailability in paddy soil. Int J Environ Res Public Health. 2020;17(7):2482. doi:10.3390/ijerph17072482. [Google Scholar] [PubMed] [CrossRef]
139. Rajput VD, Minkina T, Kumari A, Harish, Singh VK, Verma KK. Coping with the challenges of abiotic stress in plants: new dimensions in the field application of nanoparticles. Plants. 2021;10(6):1221. doi:10.3390/plants10061221. [Google Scholar] [PubMed] [CrossRef]
140. Ma J, Alshaya H, Okla MK, Alwasel YA, Chen F, Adrees M, et al. Application of cerium dioxide nanoparticles and chromium-resistant bacteria reduced chromium toxicity in sunflower plants. Front Plant Sci. 2022;13:876119. doi:10.3389/fpls.2022.876119. [Google Scholar] [PubMed] [CrossRef]
141. Loureiro S, Tourinho PS, Cornelis G, Van Den Brink NW, Díez-Ortiz M, Vázquez-Campos S. Nanomaterials as soil pollutants. In: Soil pollution. Cambridge, MA, USA: Academic Press; 2018. p. 161–90. [Google Scholar]
142. Pathan SI, Arfaioli P, Bardelli T, Ceccherini MT, Nannipieri P, Pietramellara G. Soil pollution from micro-and nanoplastic debris: a hidden and unknown biohazard. Sustainability. 2020;12(18):7255. doi:10.3390/su12187255. [Google Scholar] [CrossRef]
143. Faizan M, Alam P, Rajput VD, Faraz A, Afzal S, Ahmed SM, et al. Nanoparticle mediated plant tolerance to heavy metal stress: what we know? Sustainability. 2023;15(2):1446. doi:10.3390/su15021446. [Google Scholar] [CrossRef]
144. Ghorbani A, Emamverdian A, Pehlivan N, Zargar M, Razavi SM, Chen M. Nano-enabled agrochemicals: mitigating heavy metal toxicity and enhancing crop adaptability for sustainable crop production. J Nanobiotechnol. 2024;22(1):91. doi:10.1186/s12951-024-02371-1. [Google Scholar] [PubMed] [CrossRef]
Cite This Article
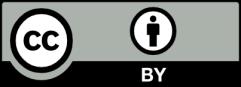
This work is licensed under a Creative Commons Attribution 4.0 International License , which permits unrestricted use, distribution, and reproduction in any medium, provided the original work is properly cited.