Open Access
REVIEW
Breaking Barriers: Selenium and Silicon-Mediated Strategies for Mitigating Abiotic Stress in Plants
1
Department of Plant Breeding, Nuclear Agriculture Research School, Nuclear Science and Technology Research Institute (NSTRI),
Atomic Energy Organization of Iran (AEOI), Karaj, Iran
2
Department of Genetics and Plant Breeding, Bangladesh Agricultural University, Mymensingh, 2202, Bangladesh
3
Department of Energy Plant Research Laboratory, Michigan State University, East Lansing, 48824, USA
4
Department of Biochemistry and Molecular Biology, Michigan State University, East Lansing, 48824, USA
* Corresponding Authors: Mohammad Anwar Hossain. Email: ; Mohammad Golam Mostofa. Email:
Phyton-International Journal of Experimental Botany 2023, 92(9), 2713-2736. https://doi.org/10.32604/phyton.2023.030372
Received 03 April 2023; Accepted 14 June 2023; Issue published 28 July 2023
Abstract
Numerous plant species, particularly those that can accumulate selenium (Se) and silicon (Si), benefit from these essential micronutrients. Se and Si accumulation in plants profoundly affects several biochemical reactions in cells. Understanding how plants react to Se/Si enrichment is crucial for ensuring adequate dietary Se/Si intake for humans and animals and increasing plant tolerance to environmental stressors. Several studies have shown that Se/Si-enriched plants are more resistant to salinity, drought, extreme temperatures, UV radiation, and excess metalloids. The interplay between Se/Si in plants is crucial for maintaining growth and development under normal conditions while providing a critical defense mechanism against stressors like heavy metals and drought. Se and Si commonly stimulate antioxidant defense systems in plants exposed to environmental stressors, but the involved mechanisms are complex and not well understood. To ensure the positive effects of Se/Si fortification in plants, it is essential to consider the degree of accumulation, the chemical form of Se/Si used, the method of application, and the likelihood of interaction with other elements. In this review, we will discuss the effects of Se/Si bio-fortification on plants subjected to abiotic stressors. Plant responses to exogenous Se/Si will also be reviewed, emphasizing the influences of Se/Si in the modulation of enzymatic and non-enzymatic antioxidant defense mechanisms under various abiotic stress conditions.Keywords
Plants require both essential and non-essential elements to complete their life cycle successfully. While essential elements are crucial for plant growth and development, there are certain non-essential elements that, when present in optimal concentrations, can also contribute to improved plant health. Among these non-essential elements, selenium (Se), silicon (Si), cobalt (Co), aluminum (Al), and sodium (Na) are notable examples. Both Si and Se play significant roles in enhancing plant growth and development, particularly in terms of abiotic and biotic stress tolerance. Se, being a metalloid mineral nutrient, is involved in vital metabolic and functional processes in organisms. The bioavailability of Se is influenced by physicochemical properties such as pH, redox potential, and clay content. In soil, the presence of these elements is determined by geochemical processes influenced by the composition of the underlying bedrock [1]. Selenate, a form of selenium, is easily absorbed by plants thriving in oxidized and alkaline soils. On the other hand, selenite, another form of selenium, is less readily absorbed by plants, particularly in soils with high clay and iron oxide content. The soil composition, specifically the presence of clays and iron oxide surfaces, hinders the efficient uptake of selenite by plants, making it less accessible compared to selenate [2].
Plants can contain both organic and inorganic forms of selenoamino acids [3], making these micronutrients crucial for their growth. In unicellular green algae, Se promotes optimal growth, but its role in higher plants is not clear. High concentrations of Se can cause selenosis in plants, inhibiting methylation and protein sulfur substitution, resulting in symptoms such as chlorosis, necrotic patches, and wilting [4]. However, non-accumulators such as beans (Phaseolus vulgaris), broccoli (Brassica oleracea var. italica), cabbage (Brassica oleracea var. capitata), corn (Zea mays), rice (Oryza sativa), and wheat (Triticum aestivum) can tolerate up to 4000 mg.kg−1 Se [5,6]. Low concentrations of this micronutrient have a variety of beneficial effects [3], promoting plant development and physiology under harsh environmental conditions. Se is an effective phytoprotectant in recent years [7–9], mitigating the effects of abiotic stressors on plants. Abiotic and biotic stresses contribute to oxidative stress, which can cause serious physiological disturbances by creating an imbalance between reactive oxygen species (ROS) and antioxidants [10]. The presence of Se can directly or indirectly influence the effects of stress on ROS generation and scavenging, benefiting plants by mitigating abiotic stressors [8]. A recent proteomics study by Wang et al. [11] showed that plants grew better under low Se doses when their photosynthesis proteins and carbohydrates were upregulated in leaves and roots, respectively. While low Se concentrations have been associated with regulating/activating antioxidative defense systems in stressed plants, several alternative mechanisms are also responsible for Se’s ability to reduce the negative effects of abiotic stresses, which will be discussed in the following sections. With global climate change affecting a growing number of arable soils and adversely affecting plants, Se may be used to enhance crops’ resistance to abiotic stresses [9].
The perception of selenium (Se) has evolved, initially being recognized for its toxic effects in animals during the 1930s, leading to conditions like hoof and hair loss. However, its essential role in the metabolism of animals, humans, and microorganisms was later acknowledged when Se deficiency was linked to white muscle disease in livestock during the 1950s. Since then, research has uncovered a wide range of biological roles for Se, including its potential in cancer prevention and its influence on hormone production. Se deficiency in the diet has been associated with various health issues, such as thyroid dysfunction, increased cardiovascular disease risk, and impaired nerve and immune system function [12]. It is crucial to maintain an optimal balance of Se for proper growth and development. Trace amounts of Se are necessary, as they support homeostatic functions, while excessive concentrations can be toxic, as stated by Hamilton [13], It has been suggested that Se is a “double-edged sword” for human health, as both insufficient and excessive intake can have negative effects [14]. Both humans and animals primarily obtain Se from their diet. Strategies to improve suboptimal Se status include diversifying the diet, using dietary supplements, fortifying food products with Se, and biofortification techniques [15]. These approaches aim to enhance Se intake and address deficiencies in Se levels to support optimal health.
The metalloid silicon (Si) is naturally found in minerals known as silicates [16]. Within the soil, it is estimated that between 0.1 and 0.6 mM of Si exists in the form of silicic acid (Si(OH)4). Si plays a critical role in plant growth and development, although it was once believed to be a non-essential element. In recent years, Si has gained significant attention due to its ability to upregulate various physiological processes, thus earning the status of a “quasi-essential” component [17]. Plants can absorb Si in the form of soluble silicic acid, particularly at pH levels equal to or below 9 [18]. Recent research has highlighted several beneficial functions of Si in plants, especially in terms of biotic and abiotic stress tolerance. Si has been reported to have a profound impact on plant growth, yield, photosynthesis, germination, leaf turgor, and gas exchange characteristics [19]. Additionally, Si enhances water status, stomatal conductance, carbon dioxide absorption, and stability/assembly of photosystems. It also protects photosynthetic pigments from degradation and regulates photosynthesis-related proteins [20–22]. By reducing nutrient imbalance stress, Si improves plant growth, development, and yield [23]. When plants face environmental stressors like drought, salinity, ultraviolet (UV) radiation, flooding (hypoxia), heavy metal toxicity, or mineral toxicity, Si plays a crucial role in promoting embryogenesis, organogenesis, and biochemical/physiological traits. Si proteins are involved in biochemical pathways, and gene activation induced by stress enhances stress tolerance [18,24]. The aforementioned articles provide in-depth discussions on the significant roles of selenium (Se) and silicon (Si) in promoting plant growth and enhancing the plant’s ability to withstand abiotic stress, particularly at low levels. The application of exogenous Se/Si at optimal concentrations can be a viable approach to foster cultivation under suboptimal conditions, contributing to improved crop performance.
2 Uptake and Transport of Se/Si in Plants
Se metabolism in higher plants closely resembles that of sulfur (S) due to their chemical similarity. The uptake of Se from the soil is influenced by plant species and growth stages, with many plants accumulating more Se in the shoots rather than the root tissues [25]. Consequently, Se is utilized in storage organs to produce seleno-derivatives of amino acids. In the environment, Se exists in various oxidation states (+6, +4, 0, and −2), which impact its solubility and mobility. Selenate (SeO42−) and selenite (SeO32−) are highly bioavailable and tend to accumulate due to their highly oxidized states [26]. Typically, soil Se concentrations range from 0.01 to 2.0 mg.kg−1, with an overall mean of 0.4 mg.kg−1 [27]. While selenite is removed from water through various mechanisms, selenate is the primary soluble form taken up by plants [28]. Selenite is more toxic than selenate due to its rapid incorporation. However, plants with higher root growth rates selectively take up and distribute selenate more efficiently, preventing selenite-mediated toxicity [7]. Root cells convert selenite into selenomethionine (SeMet), an organic form that is non-toxic. The majority of selenate is translocated to aerial plant parts via the xylem [5,29]. Selenium shares a chemical similarity with sulfur, an essential macroelement for plants. Selenate uptake in root cells is associated with a high-affinity sulfate transporter (HAST) [30]. Arabidopsis thaliana’s SULTR1 and SULTR2 sulfate transporters are involved in selenate transport [31]. Mutant studies of SULTR1;2 have identified it as the key selenate transporter in Arabidopsis. Furthermore, Se import is accelerated when plants are phosphate-deficient, indicating the involvement of phosphate transporters in Se transport [32]. Upon entry into plants, Se is converted to selenite through the combined action of ATP sulfurylase and APS reductase enzymes. This process leads to the formation of adenosine phosphoselenate (APS), which is subsequently reduced to selenite by APR (APS reductase) [30].
Several types of silicates are present in the soil, including primary and secondary silicates, as well as biogenic and inorganic silicates. Among these, mono-silicic acid (H4SiO4) is the most abundant and soluble form of silicon (Si) [18]. Despite its high presence in soil, Si is not readily absorbed by plants due to its low solubility. However, plants employ various mechanisms for Si uptake, including active transport, passive transport, and rejection transport systems, depending on the root system. Si is absorbed as silicic acid and transported to different parts of the plant [33]. Plants can be classified into three groups based on their capacity to absorb Si: intermediate plants, high Si-accumulating plants, and non-Si-accumulating plants. For instance, wheat (Triticum spp.), barley (Hordeum vulgare), and tomato (Solanum lycopersicum) exhibit only active transport, while rice (Oryza sativa), sunflower (Helianthus annuus), and maize (Zea mays) display both active and passive transport. Silicic acid is taken up by plant roots through apoplastic and symplastic pathways (Fig. 1) [17]. Within the symplastic route, Si is transported by NIPs (Nod26-like intrinsic proteins), which are a part of the aquaporin gene family. Silicon transporters in plants play a crucial role in regulating the uptake of silicic acid, maintaining physiological functions, and ion homeostasis at the plasma membrane level [34,35]. In rice, Lsi1 and Lsi6 are identified as Si transporters belonging to the aquaporin family. Si is transported throughout the plant, while Si efflux and proton-driven transport occur through Lsi2, which functions as an anion channel and facilitates Si/H+ antiport [16,17,36]. Additionally, Lsi6 inhibits the deposition of Si in the xylem by unloading it from the xylem into the xylem parenchyma. Si remains stable in the cytoplasm and vacuole despite plant decomposition [16,17,36].
Figure 1: Uptake and assimilation of Se/Si by higher plants. The uptake and assimilation of essential minerals, such as Selenium (Se) and Silicon (Si), involve complex processes in higher plants. The initial step is the uptake of these elements from the soil by the roots. Se uptake primarily occurs through the action of sulfate transporters, whereas Si uptake relies on transporters specific to Si. Once inside the plant, Se and Si are transported to different plant tissues via the xylem and phloem. The assimilation of Se and Si follows distinct metabolic pathways. Se is first converted into selenate or selenite, which is then incorporated into the amino acid selenocysteine. Selenocysteine serves as a building block for selenoproteins, which play crucial roles in various biological processes. In contrast, Si assimilation is a passive process where amorphous silica is deposited in plant cell walls, enhancing their rigidity and resistance to biotic and abiotic stresses. Si also stimulates the activity of enzymes involved in plant metabolism and facilitates the uptake of other minerals, such as phosphorus. Overall, the uptake and assimilation of Se and Si in higher plants are intricate processes that contribute to their growth, development, and stress response mechanisms. Understanding these processes is vital for optimizing plant nutrition and enhancing its resilience to environmental challenges
3 The Beneficial Role of Se/Si in Plant Growth and Development
One intriguing aspect of Se/Si is their contrasting roles in primitive plants like algae vs. higher plants. While they are essential for primitive plants, they are considered non-essential for more advanced plant species (Fig. 2). This non-essential status in higher plants may be due to the limited availability of Se/Si on the Earth’s surface, as suggested by Schiavon and Pilon-Smits [37]. Seed germination is a critical stage in seed establishment and development, and it can be affected by various factors such as drought, salinity, and heavy metals. During germination, plant seeds absorb Se from the external medium. In certain species like bitter gourd and wheat, the application of exogenous Se compounds (priming) can break seed dormancy [38,39]. Se in the form of SeMet [40] or other Se species, such as selenourea, induces ethylene (ET) production in Stylosanthes humilis seeds, leading to the breaking of seed dormancy [41]. Low doses of Se applied through seed priming induce starch catabolism enzymes, increase sugar content and cellular respiration, and enhance growth energy, resulting in improved membrane stability, antioxidant capacity, faster germination, and more vigorous growth [42]. Germinating wheat (Triticum aestivum), alfalfa (Medicago sativa), and sunflower (Helianthus annuus) seeds can accumulate significant amounts of selenium, potentially providing a valuable selenium source for humans [43]. In the case of Si, when tomato seedlings are exposed to drought conditions induced by polyethylene glycol (PEG), Si triggers an antioxidant defense system, reduces levels of malondialdehyde (MDA) and reactive oxygen species (ROS), and enhances plant resilience [44]. Applying a low dose of Si to Glycyrrhiza uralensis during salinity stress improves germination, seedling vitality index, and seedling emergence, and induces the production of superoxide dismutase (SOD), which helps scavenge ROS and MDA [45]. Si nanoparticles (Si-NPs) applied to soybeans and rice enhance germination, increase the activity of carbonic anhydrase and nitrate reductase, improve water and fertilizer uptake, and enhance the efficiency of photosynthetic machinery and antioxidant activity [46]. These findings demonstrate the potential of Se/Si in influencing seed germination, improving seedling vigor, and enhancing the antioxidant defense system, thereby contributing to the resilience and growth of plants under various stress conditions.
Figure 2: The roles of Se/Si in higher plants under normal and stress conditions
Different plant species, including wheat (Triticum spp.), cucumber (Cucumis sativus), and tobacco (Nicotiana tabacum), exhibit enhanced shoot and root growth when exogenous Se is applied [47–49]. Se promotes the growth of mungbean (Vigna radiata) plants by up-regulating enzymes involved in carbohydrate metabolism [50]. Additionally, low concentrations of Se are beneficial for plants such as lettuce (Lactuca sativa) and ryegrass (Lolium perenne), leading to improved biomass production and regulation of antioxidants, reducing lipid peroxidation [51]. In studies with hydroponically grown Arabidopsis thaliana and Brassica juncea, selenite at a concentration of 20 µM increased root biomass production [52]. Selenite has also been found to stimulate growth and dry matter yield in Brassica juncea [7]. Arabidopsis thaliana and peas exposed to selenite exhibited increased growth, with longer hypocotyls and larger cotyledons in the case of Arabidopsis [53], and significant increases in organ biomass in peas [54]. Se application has been shown to delay senescence in Glycine max seedlings and promote their overall growth [55,56]. Regarding seed germination, bitter gourd (Momordica charantia) seeds showed improved germination when primed with selenite [38]. Se application has also been found to enhance the antioxidant machinery in seeds, making them more resilient to stress. Si application has demonstrated various positive effects on plant growth and development. It enhances morphological traits, leaf area, root morphology, mechanical strength, and firmness in plants such as Capsicum, lettuce (Lactuca sativa), ryegrass (Lolium perenne), potato (Solanum tuberosum), and green tea (Camellia sinensis) [57]. Si has been shown to increase plant height, fresh weight, and dry weight in Glycine max, as well as modulate endogenous levels of phytohormones [58]. Si reduces the toxicity of metals such as Ni, Zn, and Pb in cotton (Gossypium hirsutum) by improving morphological traits, nutrient uptake, and inhibiting metal entry into the xylem [42]. The function of Si transporters is crucial for plant growth, productivity, and development. Si accumulation in plant tissues, including leaves, roots, and shoots, forms a barrier that prevents the entry of toxic metals and ions [59]. Si application to coriander enhances biomass, height, relative water content, synthesis of cell walls, leaf tissue firmness, and flexibility, leading to improved overall plant health [60]. These findings highlight the positive impact of Se and Si application on plant growth, biomass production, nutrient uptake, stress tolerance, and various physiological processes (Table 1).
Abiotic stresses generate reactive oxygen species (ROS) in plants, which can be harmful if not promptly eliminated or neutralized by cellular processes [61]. Stress-sensitive plants often lack elaborate antioxidant mechanisms to scavenge ROS and mitigate their toxic effects [62]. Uncontrolled accumulation of ROS can lead to protein degradation, lipid peroxidation, and membrane damage [63]. When lettuce plants were exposed to low concentrations of selenate, the activities of ascorbate peroxidase and glutathione peroxidase, as well as the levels of nonenzymatic antioxidants like glutathione (GSH) and ascorbate (AsA), were increased. This resulted in reduced H2O2 production in the plants [64]. Conversely, selenite treatment was found to increase the accumulation of H2O2 and malondialdehyde (MDA) in lettuce plants [64]. In wheat plants, Se treatment increased the activities of catalase (CAT) and peroxidase (POD), but higher Se concentrations decreased the activities of these antioxidant enzymes [65]. Thus, it is crucial to optimize the application dose of Se, especially at high concentrations, to ensure agricultural benefits, considering its pro-oxidant nature. In wheat plants treated with Se, nitrate reductase activity was significantly increased during the late developmental stages. This suggests that SeCys (selenocysteine) may have been incorporated into the active site bound to NADPH, increasing the redox potential of enzymes associated with SeCys [65]. SeCys has a lower pK and greater nucleophilic power than Cys, contributing to its unique properties. Si application, on the other hand, enhances protein synthesis during stress and plays a role in inducing osmoregulation. It helps maintain water balance, metabolic activity, and cellular function in various plant species. Si increases the content of proline, carbon, nitrogen, and glycine betaine (GB), thus contributing to osmotic stress tolerance [16,59,60]. Si also reduces salt toxicity by increasing glycine levels. Proline accumulation, facilitated by Si application, acts as a molecular chaperone during stress, stabilizing and protecting enzymes and proteins, maintaining membrane integrity, quenching ROS, and modulating carbon and nitrogen pools [18,21]. Additionally, Si is essential for starch and carbohydrate production in plants [22,60] (Table 1).
The synergistic interaction between Selenium (Se) and Silicon (Si) plays beneficial roles in plant growth and development. This interaction enhances various physiological and biochemical processes, leading to improved plant performance and resilience [66]. Se/Si synergism has been found to positively impact plant nutrient uptake and assimilation. Si enhances the uptake and translocation of Se within the plant, leading to increased Se concentrations in different plant tissues. This improved uptake and distribution of Se contribute to the biosynthesis of selenoamino acids and selenoproteins, which are essential for various biological functions in plants [67]. The presence of Si also influences the chemical forms of Se in plants, potentially affecting its bioavailability and metabolic fate [68]. Furthermore, Se/Si interaction promotes plant tolerance to abiotic stresses. Both Se and Si have been shown to enhance plant resistance against environmental stressors such as salinity, drought, extreme temperatures, heavy metals, and UV radiation [69]. These micronutrients stimulate antioxidant defense systems, regulate osmotic balance, and protect cellular structures from oxidative damage. The combined effect of Se and Si results in improved stress tolerance, allowing plants to better withstand challenging environmental conditions [70]. Se/Si synergism also influences plant growth and development at various stages. It has been observed to promote seed germination, enhance root and shoot growth, increase biomass production, and improve overall plant morphology [71]. Si deposition in plant cell walls enhances their rigidity, providing structural support and protection against biotic and abiotic stresses. Se, on the other hand, contributes to essential metabolic processes and enzyme activities, which are vital for plant growth and development. In addition, the interaction between Se and Si can have positive impacts on crop productivity and quality. Se supplementation in combination with Si has been shown to increase yield parameters, such as grain yield, fruit weight, and crop biomass [72]. It also enhances the nutritional value of crops by improving the accumulation of beneficial compounds such as antioxidants, vitamins, and minerals. Overall, the synergistic relationship between Se and Si plays a crucial role in promoting plant growth, increasing stress tolerance, and improving crop productivity (Table 1). Understanding and harnessing this interaction can provide valuable insights for developing sustainable agricultural practices and enhancing food security in a changing environment [66].
4 The Role of Se/Si in the Plants’ Abiotic Stress Tolerance
Crop plants are highly susceptible to various abiotic stresses such as drought, cold, salinity, heat, and UV-B rays [83]. These stresses can impair numerous physiological, biochemical, and molecular processes throughout the plant’s lifecycle, ultimately leading to a reduction in crop yield [83,84]. However, studies have demonstrated that the application of Se/Si can help mitigate the adverse effects of these stresses, including salt, drought, cold, heat, mechanical, and metal stresses (Fig. 2). Se/Si supplementation has been shown to enhance the tolerance of plants to salt stress, enabling them to maintain proper cellular function and osmotic balance under high salinity conditions [69]. Similarly, Se/Si can improve drought tolerance by regulating water status, enhancing antioxidant defense mechanisms, and reducing oxidative damage caused by water deficit [85]. Cold stress is another abiotic factor that can be alleviated by Se/Si application. They have been found to enhance cold tolerance by improving membrane stability, regulating gene expression related to cold response, and increasing the accumulation of cryoprotective compounds [72]. Se/Si also plays a role in mitigating the harmful effects of heat stress by modulating heat shock protein expression, enhancing photosynthetic efficiency, and reducing oxidative stress [86]. Furthermore, Se/Si supplementation can improve plant resistance against mechanical stress and metal toxicity, helping to maintain plant growth and development under challenging environmental conditions [87]. By reducing the negative impact of these abiotic stresses, Se/Si application holds great potential for enhancing crop resilience and productivity, ultimately contributing to food security and sustainable agriculture (Table 2).
Crop plant productivity is significantly affected by salinity, which is considered one of the most severe environmental factors. Salinity has a greater impact on plant growth and yields than any other toxic substance [92]. Soil overfertilization in pursuit of high yields, particularly in covered cultivation systems, has contributed to increased salinity levels, exacerbated by declining precipitation rates [93,94]. High salt concentrations in the soil negatively affect crop plants, leading to reduced crop yields [95,96]. Sodium and chloride ions are the main contributors to salinity, with chloride ions being particularly hazardous [97]. Hyperosmotic or hyperionic stress caused by salinity can be fatal for plants, resulting in nutrient deficiencies, membrane damage, imbalances in growth regulators, and inhibition of metabolic processes like photosynthesis [98]. Plants respond to salt stress by increasing reactive oxygen species (ROS) levels, which can have detrimental effects. However, plants have developed various mechanisms to protect their ionic homeostasis and mitigate the negative effects of salinity. These mechanisms include the accumulation of osmolytes, scavenging of harmful radicals, and regulation of water transport to maintain intracellular balance [99]. Selenium (Se) is a protective agent that improves plant growth and enhances stress tolerance, including tolerance to excessive salinity. Se can interact with soil salinity, and its uptake by plants can be affected by sulfate salinity. However, the interaction between selenium and plants varies depending on the species [100]. Studies have demonstrated the positive effects of Se on different plant species under salt stress, such as cucumber, sorrel, melon, rapeseed, lettuce, canola, tomato, and maize [101]. Se can counteract the detrimental effects of salt on salt-stressed plants when applied at low doses, and one way by which Se exerts this stress-alleviating effect is by increasing photosynthesis and protecting photosystem II [102]. The application of Se to plants upregulates their antioxidant defense system, which helps prevent lipid peroxidation and electrolyte leakage, protecting the cells from the negative effects of salinity [56,101,103]. However, the mechanisms by which Se mediates salt tolerance are not fully understood. Low concentrations of Se have been shown to enhance proline content and photosynthetic pigments in cucumber under different salt treatments, but higher concentrations can reduce plant growth [104]. As a result of the decrease of lipid peroxidation and electrolyte leakage and the upregulation of antioxidant enzymes [105], Se was able to protect from NaCl treatment in Cucumis sativus. It is unclear, however, how Se mediates salt tolerance. Some studies report that Se induces antioxidant machinery in salt-exposed plants. Nevertheless, other studies suggest that this is not an exhaustive analysis of the metabolic effects of this element. In soybean seedlings treated with Se, proline levels increased [56], suggesting that proline signaling is directly correlated with Se signaling under stress conditions. Sulfate salinity can significantly inhibit plants’ ability to absorb Se compared to chloride salinity due to competition with Se for root absorption. In contrast, chloride-induced salt stress rarely decreases Se uptake [7]. Si has been shown to reduce the negative effects of various abiotic stresses, including salt, drought, cold, heat, metals, and mechanical stresses. The application of Si can reduce salt-induced depletion and increase various parameters related to photosynthesis, including leaf area, photosynthetic pigments, gas exchange parameters, and chlorophyll fluorescence, among others. Si also contributes to the accumulation of starch and sugar [106]. In addition to increasing salinity tolerance, Si-NPs enhance the biomass, growth, and NPK content of plants inhibited by salinity. Si increases osmolytes, such as PAs, proline, GB, GABA, and carbohydrates, and also upregulates antioxidant enzymes like POX, CAT, APX, SOD, and GR to reduce salt-induced ionic stress and other antioxidants like ascorbic acid, glutathione, flavonoids, phenolics, and carotenoid [16,107]. Higher Si accumulation at the seedling stage contributes to salt tolerance in salinity-tolerant landrace rice Pokkali [108]. Several mechanisms have been implicated in the tolerance of abiotic stress to Si either directly or indirectly, such as an increase in Fv/Fm, PSII, and Fv′/Fm′ in cucumber under salinity stress upon Si application [109] (Table 3).
Drought stress is a major factor affecting crop yield and production [8,26]. In some cases, excessive salt ions may cause drought stress due to limited water supply caused by soil drought, leading to an osmotic imbalance and water deficit in plants [9]. The resulting weight loss, reduced photosynthesis, and upregulated antioxidant defense systems lead to oxidative stress [110]. Se has been reported to protect plants under drought stress. Kuznetsov et al. [111] found that Se can mitigate drought stress in some plants by regulating water status. Se ions may also contribute to preserving an optimal water status [112]. Emam et al. [113] reported that osmoprotectants, such as inorganic (P and Ca ions) or organic (carbohydrates and proteins), accumulate in wheat tissues to improve water content. Se promotes the biosynthesis of proline and peroxidases and enhances the antioxidant defense system of plants under drought stress by enhancing CAT, SOD, POD, AsA, GSH, and alpha-tocopherol levels, according to Ahmad et al. [114] and Ibrahim [115]. However, the application of Se did not improve the growth of water-stressed plants, despite its stimulatory effect on photosynthesis and antioxidant activity [9]. Yao et al. [116] found that low doses of Se increased chlorophyll and carotenoid content and antioxidant enzyme activity in wheat under drought stress. In addition, the foliar application of Se improved turgor in wheat stressed by drought by lowering osmotic potential, reducing transpiration, and increasing antioxidant capacity [117]. When wheat plants were pretreated with Se, they accumulated fewer H2O2 and had lower levels of lipid peroxidation, while their antioxidant enzyme activity and non-enzymatic antioxidant content increased. Thus, under stress conditions, Se-pretreated plants showed enhanced root viability and membrane stability indices. Se pretreatment, however, reduced the activities of POX and proline content to some extent, which may indicate reduced oxidative stress caused by drought stress [115]. According to Nawaz et al. [118], wheat treated with Se under water deficit showed increased photosynthetic rates and stomatal conductance, which could be attributed to the positive effects of Se ions on maintaining turgor and stimulating antioxidant enzymes. Consequently, spraying selenium-selenate solutions improved the yield, fiber, and Se content of fodder. Antioxidant activity was also boosted in drought-stressed maize containing Se-selenite [119]. The highest grain yield under water deficit was obtained with Se fertilization at the grain filling stage, although micronutrients decreased antioxidant status and grain yield when used together with Se [119]. Therefore, it is not recommended to use them together in drought conditions. Using Se-selenate as a pretreatment, Emam et al. [113] demonstrated a lessening of adverse drought effects and improved rice yields. Considering the above-mentioned information, it is likely that Se plays a protective role under water deficit through several mechanisms: (i) enhancing root water uptake to regulate plant water status, (ii) protecting cells from oxidative damage, (iii) stimulating solute accumulation, and (iv) regulating the levels of certain inorganic ions in plant tissues. By increasing chlorophyll fluorescence, photosynthesis, proline content, and stomatal conductance in strawberry plants, Si is efficient in reducing water scarcity. Additionally, it reduces electrolyte leakage, transpiration, and water use efficiency [120]. There was an increase in oxidative stress and drought in Glycine max as a result of PEG, as well as a decrease in osmotic potential and plant turgor. Si supplementation enhances plant growth, turgor, photosynthetic traits, and antioxidant enzymes, as well as the production of compatible osmoregulators, reducing the toxic effect of stress on plants. Several endogenous phytohormones, including SA, GA, and JA, were upregulated [58]. During water deficit conditions, Si-NPs reduce plant yield attributes, growth traits, stomatal conductance, photosynthesis, xylem water potential, leaf water potential, and relative leaf water content. Si-NPs application energizes drought and increases plant tolerance. Further, Si mitigates water scarcity, increases cell membrane integrity, prevents electrolyte leakage, and triggers lipid peroxidation and oxidative damage. Moreover, it increases Si, Na, and P content in the soil, which induces morphological properties and mitigates drought [46].
Based on the information, it seems that selenium (Se) and silicon (Si), especially in the form of nanoparticles (Se/SiO2-NPs), can help plants cope with osmotic stress conditions like drought. Drought is a significant environmental stressor that negatively affects plant growth and yield. One key plant physiological response to drought stress is the synthesis and accumulation of compatible solutes, also known as osmolytes or osmoprotectants. These compounds lower the cell water potential and enhance the water extraction capacity in environments with limited water resources. Therefore, enhancing plant tolerance to drought and other types of osmotic stress is a crucial area of research in crop production [78]. In a study investigating the effects of Se and Si nanoparticles on strawberry plants, it was found that spraying solutions containing these nanoparticles improved growth and yield parameters under normal and drought stress conditions. Specifically, plants treated with Se/SiO2 nanoparticles preserved more of their photosynthetic pigments compared to other treated plants and exhibited higher levels of key osmolytes such as carbohydrates and proline. The treatment also increased relative water content (RWC), membrane stability index (MSI), and water use efficiency (WUE). Moreover, it increased the activity of antioxidant enzymes, which can protect cells from damage caused by reactive oxygen species during stress conditions, and decreased lipid peroxidation and hydrogen peroxide content, suggesting an improvement in the plant’s stress response mechanisms. This treatment also improved the biochemical parameters of fruits, enhancing their quality and nutritional value11https://www.nature.com/articles/s41598-020-74273-9. While Se and Si have not yet been identified as essential elements for plant growth, there have been reports on their favorable influences on plant growth and development, yield, and abiotic stress tolerance. When applied at low concentrations, these elements have been found to enhance the growth parameters and quality values of various plants under controlled conditions, indicating their importance for both stress adaptation and plant development. The application of a novel Se/SiO2 nanoparticles composition appears to have even greater influences [78]. In another study, Taha et al. [121] provided insights into the synergistic effects of Selenium (Se) and Silicon (Si) on wheat plants under salinity stress, which is a form of osmotic stress. The study tested various combinations of Se and Si in alleviating the deleterious effects of salinity stress on wheat plants’ anatomical characteristics and physio-biochemical parameters. The findings revealed that Se and Si treatments and their combinations caused a significant amelioration in growth, anatomical and physiological attributes, and grain yields of salinity-stressed wheat compared to the untreated plants. Specifically, the integrated application of Se and Si at concentrations of 30 mM each significantly increased plant growth (including plant height, number of tillers, fresh weight per plant, and dry weight per plant), membrane stability index, relative water content, total soluble sugars, proline, enzymatic antioxidants (CAT, GR, SOD, APX), non-enzymatic antioxidants (GSH, AsA), and yield components. The anatomical traits of stems and leaves also improved in wheat plants treated with 30 mM Se and 30 mM Si. These results suggest that exogenous applications of Se, Si, or their combinations can potentially help wheat plants acclimate successfully to saline soil, demonstrating the synergistic effect of Se and Si under osmotic stress conditions [121].
4.2 Se/Si and Temperature Stress
Low temperatures pose a significant threat that limits plant growth, particularly in subtropical and temperate regions, and can affect plant reproduction [128]. Cold alters the composition of lipids, affecting membrane fluidity, but no Se-related effects have been described. In wheat and sorghum plants, Se has been shown to improve photosynthetic capacity and carbohydrate metabolism by reducing chlorophyll loss under suboptimal temperatures [129]. Cold-stressed plants have higher levels of free proline, which may improve chilling tolerance but can also indicate injury. According to Abbas and Hawrylak-Nowak [104], low Se doses enhanced proline accumulation in cold-stressed cucumbers and sorghums. However, the correlation between lower Se levels and improved chilling tolerance remains unclear. Cold indirectly affect plant physiology through secondary oxidative stress induced by ROS overproduction and lipid peroxidation. Applied Se enhances antioxidant content such as anthocyanins, carotenoids, flavonoids, phenolic compounds, ascorbate, and antioxidant enzyme activity such as APX, POX, and CAT. The effect of Se-induced oxidative stress caused by chilling is ameliorated by both enzymes and non-enzymatic antioxidants [129]. The molecular mechanisms of Se-triggered cold endurance will need to be explored to clarify these relationships. Haghighi et al. [130] found that applying Se (as selenite and nano-Se) to tomato plants under short-term suboptimal and high-temperature conditions was beneficial. Free endogenous proline accumulation and cold resistance in plants have a positive correlation [131]. Similarly, most plants sensitive to cold do not become more tolerant of this stress factor by accumulating free proline, unless proline is applied exogenously. Compared to plants that are not enriched with Se, Se-biofortified plants have reduced lipid peroxidation in their roots. Applying Se to cucumber tissue increased proline levels in leaf tissues and inhibited lipid peroxidation, resulting in a different physiological response to cold stress. Plants biofortified with Se did not differ significantly from plants not biofortified with Se in biomass or photosynthetic pigment levels. A recent study conducted by Chu et al. [132] showed that supplying Se-selenite to wheat seedlings could improve both the growth rate and the antioxidant capacity of the plants. During their study, they found that fertilizing the soil with 1.0 mg of Se per kilogram of soil resulted in a reduction of ROS production as well as decreased lipid peroxidation. Different concentrations of Se induced an increase in antioxidant enzyme and compound activity (POX, CAT, anthocyanins, flavonoids, and phenolic acids). Plant growth, development, and yield can be negatively affected by cold stress caused by chilling and freezing. Cold stress can damage the structure of the cell membrane by increasing ROS and lipid peroxidation. However, Si application has been shown to have a significant positive impact on plant growth and development under cold-stress conditions. Si application has been observed to increase plant height, biomass, and relative water content. Additionally, Si has been shown to increase chlorophylls, carotenoids, anthocyanins, total protein, and amino acids. Cold stress can also deactivate reaction centers, reduce electron transport, and trap excitation energy, but Si application can help to mitigate these effects by increasing these characteristics. Si application can also reduce ROS content, enhance mineral ion homeostasis, and stimulate phytohormone synthesis, all of which can help to improve plant tolerance to cold stress [133] (Table 4).
High temperatures hurt plant growth and productivity worldwide, causing degradation of chloroplast and mitochondria ultrastructure, as well as increasing ROS accumulation. Photosynthesis can be inhibited by high temperatures before other cellular functions are impaired [134]. In wheat under heat stress, Se sprays increased chlorophyll content, photosynthetic rate, stomatal conductance, and transpiration rate [135]. Se further enhanced SOD, CAT, and POD enzyme activity while decreasing the accumulation of H2O2 and superoxide caused by heat stress. Plants grown under thermal stress commonly experience oxidative stress. Due to reduced antioxidant defense under heat stress, Djanaguiraman et al. [135] observed a lower grain yield in sorghum. Foliar application of Se (as selenate) a few days before heat stress reduced membrane injury and increased grain yield by increasing antioxidant defenses. Hasanuzzaman et al. [136] found that antioxidant defense was only upregulated when high-temperature stress was present using rapeseed. This study investigated the impact of Se supplementation and heat treatment on antioxidant enzyme activity, which revealed that monodehydroascorbate reductase GR, (MDHAR), dehydroascorbate reductase (DHAR), CAT, GPX, Gly I, and Gly II showed enhanced activity. Wheat grain yield was increased under high-temperature stress when Se-selenate was applied to foliar, according to Iqbal et al. [137]. Se application under heat stress conditions increased the levels of enzyme-bound and non-enzymatic antioxidants while decreasing the levels of oxidants (H2O2, lipid peroxidation products).
4.3 Se/Si and Heavy Metal Stress
Metal toxicity has become a significant stressor for plants due to urbanization, industrialization, and natural processes. Metal contamination in cultivated soils can be caused by atmospheric pollution, chemical fertilizers, pesticides, and poor-quality wastewater irrigation. Even though metals are essential at physiological concentrations, they can be harmful to plants when present in excess [143]. Low concentrations of Se have been found to protect plants from metal toxicity in various studies (Table 5). Se can mitigate metal-induced oxidative stress mainly by reducing metal uptake by plants, modifying their translocation, decreasing metal mobility in soils, and changing cell membrane structure [144]. Excess trace metals often cause photosynthetic disturbances leading to oxidative stress and increased ROS accumulation. Se can improve plant defenses against oxidative stress by reducing ROS generation and enhancing the antioxidant system at low concentrations. Several studies have observed Se-induced effects in this regard. For example, Cartes et al. [145] found that Se-selenite inhibited Al-induced toxicity primarily by increasing POX activation and H2O2 dismutation. Furthermore, Filek et al. [146] demonstrated that adding Se-selenate to a Cd-containing medium reduced H2O2 accumulation in rape seedlings and lipid peroxidation. Se applied alone or in conjunction with Cd did not affect antioxidative enzyme activity in the roots but reduced it in the leaves. In a similar study investigating the effect of Se-selenite on As-arsenite toxicity in rice, antioxidative phenolic compounds such as ferulic acid, gallic acid, and protocatechuic acid were detected, and Se increased enzymes related to thiol metabolisms, such as cysteine synthase (CS) and serine acetyltransferase (SAT) [147]. Plants exposed to excess trace metals showed an increase in photosynthetic efficiency under Se ions, likely due to Se’s antioxidative properties [148,149]. Se may also induce trace metal detoxification by inhibiting the uptake and translocation of metals from roots to shoots [9]. When present in a Cd-containing medium, Se-selenite significantly inhibited the translocation of Cd to the generative organs of peppers and increased the yield of Cd-exposed peppers by increasing selected productive parameters [150]. Se-selenite application to rice grains markedly decreased the concentration of Cd in the grains, but Se biofortification had no significant effect on grain Pb accumulation [151] (Table 5).
Se has been shown to have a positive impact on the membrane stability of plants under metal stress conditions [152,153]. In a study on the effect of Se on copper (Cu) toxicity in cucumber, Se-selenite application decreased Cu uptake and increased membrane stability index, electrolyte leakage, and relative water content Rehman et al. [154]. Similarly, in rice exposed to excess Cd, Se-selenite reduced membrane damage and lipid peroxidation by enhancing the activity of antioxidant enzymes such as SOD, CAT, and APX [155]. In addition to its direct impact on membrane stability, Se also modulates the expression of genes related to membrane integrity. For example, in a study on the effect of Se on cadmium (Cd) toxicity in tomatoes, Se-selenite upregulated the expression of genes related to membrane transport and integrity, such as plasma membrane H+-ATPase and aquaporin [156]. These findings suggest that Se can protect plants from metal toxicity by improving membrane stability and regulating gene expression related to membrane integrity. Si has also been found to alleviate the toxic effects of Cr stress in plants. Si application increased plant growth and reduced oxidative damage caused by Cr, as well as increased antioxidant enzyme activity and non-enzymatic antioxidant content. Si also reduced Cr uptake and translocation in different parts of the plant, resulting in decreased Cr accumulation [155]. In a study on soybean plants, Si application significantly decreased Cr-induced oxidative stress by improving antioxidant enzyme activity and reducing lipid peroxidation [156]. Furthermore, Si application increased the activity of enzymes involved in the detoxification of Cr, such as ascorbate peroxidase, catalase, and glutathione reductase. The study also found that Si decreased Cr content in soybean plants and increased the availability of essential mineral nutrients such as Fe, Mg, and Ca. Similarly, in wheat plants, Si application decreased Cr uptake and accumulation, improved plant growth and biomass, and reduced oxidative damage caused by Cr stress. Si also enhanced the activity of antioxidant enzymes and increased the content of non-enzymatic antioxidants. These findings suggest that Si application can be a useful strategy to alleviate the toxic effects of heavy metals such as Cd and Cr on plants. Excessive use of Cr impairs the physio-biochemical properties of plants, negatively affecting photosynthesis, stomatal conductance, and chloroplast ultrastructure. This toxicity induces ROS production and increases MDA content. Si application induces antioxidant enzyme activities, such as CAT, SOD, APX, GR, and POX, which reduce Cr toxicity. Additionally, Si modulates the thylakoid membrane, starch granules, and vacuolar size, improving photosynthesis attributes, gas exchange parameters, and PSII efficiency. As a result, Si is more effective than Cr’s adversary at mitigating stress. During Cr stress, Si acts as a scavenger for Cr, enhancing plant growth, development, and sustenance [157]. Silicon supplementation enhances the absorption and translocation of minerals such as potassium, magnesium, and calcium. Moreover, it improves thylakoid structure, starch content, and chloroplast ultrastructure, leading to increased photosynthesis, gas exchange parameters, and chloroplast ultrastructure. As a result, Si reduces the toxic effects of Cr [36]. The non-essential element lead (Pb) is toxic to plants even in small amounts. Pb stress negatively affects the chromosomes and cells of plants, inhibiting germination, plant growth, biomass, and photosynthetic traits. Silicon nanoparticles (Si-NPs) can mitigate Pb stress by increasing plant morphological traits and photosynthetic attributes, inducing proline content, and promoting the activity of antioxidant enzymes and non-enzymatic enzymes. As a result, electrolyte leakage is reduced, and oxidative stress is depleted in coriander [60]. The role of Si in lowering Pb translocation within plants cannot be overstated. Imtiaz et al. [36] found that Si improves photosynthesis, SPAD value, growth, and development processes by increasing antioxidant enzyme activity, reducing ROS and MDA contents, and decreasing electrolyte leakage. Bamboo plants benefit from SiO2 NPs as they increase the activity of phenylalanine ammonia-lyase (PAL), catalase, superoxide dismutase (SOD), and glutathione reductase (GR) to reduce ROS and polyphenol oxidase (PPO). Additionally, Si enhances bamboo growth, biomass, chlorophyll content, protein content, and photosynthetic machinery [155]. Arsenic (As) stress is one of the most severe problems prevailing worldwide due to its hazardous effects on soil and plant development. Increasing germination, height, biomass, photosynthesis, carbohydrate metabolism, and nutrition efficiency are all important for mitigating As toxicity. Moreover, it enhances yield and improves antioxidant and thiolic systems. Si in wheat increases morphophysiological traits, protein content, mineral absorption, and membrane integrity, detoxifying As stress. Additionally, Si enhances As cellular sequestration and homeostasis, decreases ROS levels, and reduces As translocation and accumulation in above-ground parts. Metallothionein synthase and phytochelatin synthase are also upregulated by Si, helping to mitigate As stress [156].
The synergism between selenium and silicon can potentially provide even greater protection against heavy metal stress. When used together, selenium and silicon could complement each other’s protective mechanisms, potentially offering greater protection than either one alone [72]. However, more research is needed to fully understand the mechanisms of this synergism and to develop effective strategies for using these elements to mitigate heavy metal stress in plants. It is important to note that while low levels of selenium and silicon are beneficial, high levels can be toxic to plants [66]. Therefore, the application of these elements should be carefully managed to avoid causing additional stress to plants. Also, other factors like soil pH, temperature, and the presence of other nutrients can influence the effectiveness of selenium and silicon in mitigating heavy metal stress.
5 Conclusions and Future Prospective
The use of selenium and silicon is beneficial in enhancing plant tolerance to abiotic stresses by inducing stress-related genes, proteins, and enzymes, ultimately leading to increased stress tolerance. Selenium has been shown to alleviate the negative effects of various abiotic stresses such as drought, salinity, and heavy metal toxicity, while silicon has been found to improve plant tolerance to multiple stresses including drought, salinity, heavy metals, and pathogens. In addition, these elements regulate ROS levels, reduce oxidative damage, and enhance antioxidant defense mechanisms, supporting their role in improving plant stress tolerance. Furthermore, silicon supplementation has been found to improve plant growth, yield, and quality under normal and stressful conditions.
The mechanisms underlying the role of selenium and silicon in plant stress tolerance are still not fully understood, and future research efforts should focus on unraveling the specific signaling pathways and regulatory mechanisms involved in the plant response to these elements under different stress conditions. In addition, the development of genetic engineering tools and techniques aimed at enhancing the accumulation and transport of selenium and silicon in plants holds promise as a strategy to improve crop productivity and sustainability.
Funding Statement: The authors received no specific funding for this study.
Author Contributions: The authors confirm their contribution to the paper as follows: study conception and design: Mojtaba Kordrostami and Ali Akbar Ghasemi-Soloklui; data collection: Mojtaba Kordrostami and Ali Akbar Ghasemi-Soloklui; draft manuscript preparation: Mojtaba Kordrostami and Ali Akbar Ghasemi-Soloklui; writing-review and editing: Mojtaba Kordrostami, Mohammad Anwar Hossain and Mohammad Golam Mostofa. All authors reviewed the results and approved the final version of the manuscript.
Conflicts of Interest: The authors declare that they have no conflicts of interest to report regarding the present study.
References
1. Dhillon, K., Dhillon, S. (2003). Distribution and management of seleniferous soils. Advances in Agronomy, 79(1), 119–184. [Google Scholar]
2. Mikkelsen, R., Page, A., Bingham, F. (1989). Factors affecting selenium accumulation by agricultural crops. Selenium in Agriculture and the Environment, 23, 65–94. [Google Scholar]
3. Finley, J. W., Ip, C., Lisk, D. J., Davis, C. D., Hintze, K. J. et al. (2001). Cancer-protective properties of high-selenium broccoli. Journal of Agricultural and Food Chemistry, 49(5), 2679–2683. [Google Scholar] [PubMed]
4. Mengel, K., Kirkby, E. (1987). Principles of plant nutrition, pp. 687–695. Bern: International Potash Institute. [Google Scholar]
5. Shrift, A. (1969). Aspects of selenium metabolism in higher plants. Annual Review of Plant Physiology, 20(1), 475–494. [Google Scholar]
6. Aggarwal, M., Sharma, S., Kaur, N., Pathania, D., Bhandhari, K. et al. (2011). Exogenous proline application reduces phytotoxic effects of selenium by minimising oxidative stress and improves growth in bean (Phaseolus vulgaris L.) seedlings. Biological Trace Element Research, 140, 354–367. [Google Scholar] [PubMed]
7. Hassanuzzaman, M., Hossain, M. A., Fujita, M. (2010). Selenium in higher plants: Physiological role, antioxidant metabolism and abiotic stress tolerance. Journal of Plant Sciences, 5(4), 354–375. [Google Scholar]
8. Feng, R., Wei, C., Tu, S. (2013). The roles of selenium in protecting plants against abiotic stresses. Environmental and Experimental Botany, 87, 58–68. [Google Scholar]
9. Sieprawska, A., Kornas, A., Filek, M. (2015). Involvement of selenium in protective mechanisms of plants under environmental stress conditions–review. Acta Biologica Cracoviensia, 57(1), 9–20. [Google Scholar]
10. Lin, L., Zhou, W., Dai, H., Cao, F., Zhang, G. et al. (2012). Selenium reduces cadmium uptake and mitigates cadmium toxicity in rice. Journal of Hazardous Materials, 235, 343–351. [Google Scholar] [PubMed]
11. Wang, Y. D., Wang, X., Wong, Y. S. (2012). Proteomics analysis reveals multiple regulatory mechanisms in response to selenium in rice. Journal of Proteomics, 75(6), 1849–1866. [Google Scholar] [PubMed]
12. Rayman, M. P. (2000). The importance of selenium to human health. The Lancet, 356(9225), 233–241. [Google Scholar]
13. Hamilton, S. J. (2004). Review of selenium toxicity in the aquatic food chain. Science of the Total Environment, 326(1–3), 1–31. [Google Scholar] [PubMed]
14. Hawrylak-Nowak, B., Matraszek, R., Pogorzelec, M. (2015). The dual effects of two inorganic selenium forms on the growth, selected physiological parameters and macronutrients accumulation in cucumber plants. Acta Physiologiae Plantarum, 37, 1–13. [Google Scholar]
15. Malagoli, M., Schiavon, M., dall’Acqua, S., Pilon-Smits, E. A. (2015). Effects of selenium biofortification on crop nutritional quality. Frontiers in Plant Science, 6, 280. [Google Scholar] [PubMed]
16. Khan, A., Khan, A. L., Muneer, S., Kim, Y. H., Al-Rawahi, A. et al. (2019). Silicon and salinity: Crosstalk in crop-mediated stress tolerance mechanisms. Frontiers in Plant Science, 10, 1429. [Google Scholar] [PubMed]
17. Al Murad, M., Khan, A. L., Muneer, S. (2020). Silicon in horticultural crops: Cross-talk, signaling, and tolerance mechanism under salinity stress. Plants, 9(4), 460. [Google Scholar] [PubMed]
18. Abdelaal, K. A., Mazrou, Y. S., Hafez, Y. M. (2020). Silicon foliar application mitigates salt stress in sweet pepper plants by enhancing water status, photosynthesis, antioxidant enzyme activity and fruit yield. Plants, 9(6), 733. [Google Scholar] [PubMed]
19. Souri, Z., Khanna, K., Karimi, N., Ahmad, P. (2021). Silicon and plants: Current knowledge and future prospects. Journal of Plant Growth Regulation, 40, 906–925. [Google Scholar]
20. Verma, K. K., Song, X. P., Zeng, Y., Li, D. M., Guo, D. J. et al. (2020). Characteristics of leaf stomata and their relationship with photosynthesis in Saccharum officinarum under drought and silicon application. ACS Omega, 5(37), 24145–24153. [Google Scholar] [PubMed]
21. Hassan, H., Alatawi, A., Abdulmajeed, A., Emam, M., Khattab, H. (2021). Roles of Si and SiNPs in improving thermotolerance of wheat photosynthetic machinery via upregulation of PsbH, PsbB and PsbD genes encoding PSII core proteins. Horticulturae, 7(2), 16. [Google Scholar]
22. Kafi, M., Nabati, J., Ahmadi-Lahijani, M. J., Oskoueian, A. (2021). Silicon compounds and potassium sulfate improve salinity tolerance of potato plants through instigating the defense mechanisms, cell membrane stability, and accumulation of osmolytes. Communications in Soil Science and Plant Analysis, 52(8), 843–858. [Google Scholar]
23. Fatemi, H., Esmaiel Pour, B., Rizwan, M. (2021). Foliar application of silicon nanoparticles affected the growth, vitamin C, flavonoid, and antioxidant enzyme activities of coriander (Coriandrum sativum L.) plants grown in lead (Pb)-spiked soil. Environmental Science and Pollution Research, 28, 1417–1425. [Google Scholar] [PubMed]
24. Kim, Y. H., Khan, A. L., Lee, I. J. (2016). Silicon: A duo synergy for regulating crop growth and hormonal signaling under abiotic stress conditions. Critical Reviews in Biotechnology, 36(6), 1099–1109. [Google Scholar] [PubMed]
25. Turakainen, M. (2007). Selenium and its effects on growth, yield and tuber quality in potato. Julkaisuja/Helsingin yliopisto, Soveltavan biologian laitos. [Google Scholar]
26. Saha, U., Fayiga, A., Sonon, L. (2017). Selenium in the soil-plant environment: A review. International Journal of Applied Agricultural Sciences, 3(1), 1–18. [Google Scholar]
27. Johnson, C. C., Fordyce, F. M., Rayman, M. P. (2010). Symposium on ‘Geographical and geological influences on nutrition’ Factors controlling the distribution of selenium in the environment and their impact on health and nutrition. Proceedings of the Nutrition Society, 69(1), 119–132. [Google Scholar] [PubMed]
28. Martens, D. (2003). Selenium. In: Stewart, B. A., Howel, T. A. (Eds.Encyclopedia of water sciences. New York: Marcel Dekker. [Google Scholar]
29. Zayed, A., Lytle, C. M., Terry, N. (1998). Accumulation and volatilization of different chemical species of selenium by plants. Planta, 206, 284–292. [Google Scholar]
30. Sors, T. G., Ellis, D. R., Salt, D. E. (2005). Selenium uptake, translocation, assimilation and metabolic fate in plants. Photosynthesis Research, 86, 373–389. [Google Scholar] [PubMed]
31. El Kassis, E., Cathala, N., Rouached, H., Fourcroy, P., Berthomieu, P. et al. (2007). Characterization of a selenate-resistant Arabidopsis mutant. Root growth as a potential target for selenate toxicity. Plant Physiology, 143(3), 1231–1241. [Google Scholar] [PubMed]
32. Li, H. F., McGrath, S. P., Zhao, F. J. (2008). Selenium uptake, translocation and speciation in wheat supplied with selenate or selenite. New Phytologist, 178(1), 92–102. [Google Scholar] [PubMed]
33. Tripathi, D. K., Vishwakarma, K., Singh, V. P., Prakash, V., Sharma, S. et al. (2021). Silicon crosstalk with reactive oxygen species, phytohormones and other signaling molecules. Journal of Hazardous Materials, 408, 124820. [Google Scholar] [PubMed]
34. Rawat, N., Singla-Pareek, S. L., Pareek, A. (2021). Membrane dynamics during individual and combined abiotic stresses in plants and tools to study the same. Physiologia Plantarum, 171(4), 653–676. [Google Scholar] [PubMed]
35. Subba, A., Tomar, S., Pareek, A., Singla-Pareek, S. L. (2021). The chloride channels: Silently serving the plants. Physiologia Plantarum, 171(4), 688–702. [Google Scholar] [PubMed]
36. Imtiaz, M., Rizwan, M. S., Mushtaq, M. A., Ashraf, M., Shahzad, S. M. et al. (2016). Silicon occurrence, uptake, transport and mechanisms of heavy metals, minerals and salinity enhanced tolerance in plants with future prospects: A review. Journal of Environmental Management, 183, 521–529. [Google Scholar] [PubMed]
37. Schiavon, M., Pilon-Smits, E. A. (2017). The fascinating facets of plant selenium accumulation-biochemistry, physiology, evolution and ecology. New Phytologist, 213(4), 1582–1596. [Google Scholar] [PubMed]
38. Chen, C. C., Sung, J. M. (2001). Priming bitter gourd seeds with selenium solution enhances germinability and antioxidative responses under sub-optimal temperature. Physiologia Plantarum, 111(1), 9–16. [Google Scholar]
39. Peng, A., Xu, Y., Wang, Z. J. (2001). The effect of fulvic acid on the dose effect of selenite on the growth of wheat. Biological Trace Element Research, 83, 275–279. [Google Scholar] [PubMed]
40. Barros, R. S., de Paula Freitas, A. W. (2001). Selenomethionine as a dormancy-breaking agent in seeds of Stylosanthes humilis. Acta Physiologiae Plantarum, 23, 279–284. [Google Scholar]
41. Pinheiro, F. J. A., Barros, R. S., Ribeiro, D. M., Lana Souza, D., Coelho, T. G. (2008). Efficiency of selenium compounds in breaking dormancy of Townsville stylo seeds. Seed Science and Technology, 36(2), 271–282. [Google Scholar]
42. Khaliq, A., Aslam, F., Matloob, A., Hussain, S., Geng, M. et al. (2015). Seed priming with selenium: Consequences for emergence, seedling growth, and biochemical attributes of rice. Biological Trace Element Research, 166, 236–244. [Google Scholar] [PubMed]
43. Lintschinger, J., Fuchs, N., Moser, J., Kuehnelt, D., Goessler, W. (2000). Selenium-enriched sprouts. A raw material for fortified cereal-based diets. Journal of Agricultural and Food Chemistry, 48(11), 5362–5368. [Google Scholar] [PubMed]
44. Shi, Y., Zhang, Y., Yao, H., Wu, J., Sun, H. et al. (2014). Silicon improves seed germination and alleviates oxidative stress of bud seedlings in tomato under water deficit stress. Plant Physiology and Biochemistry, 78, 27–36. [Google Scholar] [PubMed]
45. Zhang, X. H., Zhou, D., Cui, J. J., Ma, H. L., Lang, D. Y. et al. (2015). Effect of silicon on seed germination and the physiological characteristics of Glycyrrhizauralensis under different levels of salinity. The Journal of Horticultural Science and Biotechnology, 90(4), 439–443. [Google Scholar]
46. Siddiqui, H., Ahmed, K. B. M., Sami, F., Hayat, S. (2020). Silicon nanoparticles and plants: Current knowledge and future perspectives. Sustainable Agriculture Reviews: Nanotechnology for Plant Growth and Development, 41, 129–142. [Google Scholar]
47. Haghighi, M., Teixeira da Silva, J. A. (2016). Influence of selenium on cadmium toxicity in cucumber (Cucumis sativus cv. 4200) at an early growth stage in a hydroponic system. Communications in Soil Science and Plant Analysis, 47(2), 142–155. [Google Scholar]
48. Han, D., Li, X., Xiong, S., Tu, S., Chen, Z. et al. (2013). Selenium uptake, speciation and stressed response of Nicotiana tabacum L. Environmental and Experimental Botany, 95, 6–14. [Google Scholar]
49. Hawrylak-Nowak, B. (2015). Selenite is more efficient than selenate in alleviation of salt stress in lettuce plants. Acta Biologica Cracoviensia, 57(2), 49–54. [Google Scholar]
50. Malik, J. A., Kumar, S., Thakur, P., Sharma, S., Kaur, N. et al. (2011). Promotion of growth in mungbean (Phaseolus aureus Roxb.) by selenium is associated with stimulation of carbohydrate metabolism. Biological Trace Element Research, 143, 530–539. [Google Scholar] [PubMed]
51. Xue, T., Hartikainen, H., Piironen, V. (2001). Antioxidative and growth-promoting effect of selenium on senescing lettuce. Plant and Soil, 237, 55–61. [Google Scholar]
52. Molnár, Á., Feigl, G., Trifán, V., Ördög, A., Szőllősi, R. et al. (2018). The intensity of tyrosine nitration is associated with selenite and selenate toxicity in Brassica juncea L. Ecotoxicology and Environmental Safety, 147, 93–101. [Google Scholar]
53. Lehotai, N., Kolbert, Z., Pető, A., Feigl, G., Ördög, A. et al. (2012). Selenite-induced hormonal and signalling mechanisms during root growth of Arabidopsis thaliana L. Journal of Experimental Botany, 63, 5677–5687. [Google Scholar] [PubMed]
54. Lehotai, N., Lyubenova, L., Schröder, P., Feigl, G., Ördög, A. et al. (2016). Nitro-oxidative stress contributes to selenite toxicity in pea (Pisum sativum L). Plant and Soil, 400, 107–122. [Google Scholar]
55. Djanaguiraman, M., Devi, D. D., Shanker, A. K., Sheeba, J. A., Bangarusamy, U. (2004). Impact of selenium spray on monocarpic senescence of soybean (Glycine max L.). Journal of Food Agriculture and Environment, 2, 44–47. [Google Scholar]
56. Djanaguiraman, M., Devi, D. D., Shanker, A. K., Sheeba, J. A., Bangarusamy, U. (2005). Selenium-an antioxidative protectant in soybean during senescence. Plant and Soil, 272, 77–86. [Google Scholar]
57. Simojoki, A., Xue, T., Lukkari, K., Pennanen, A., Hartikainen, H. (2003). Allocation of added selenium in lettuce and its impact on roots. Agricultural and Food Science in Finland, 12(3–4), 155–164. [Google Scholar]
58. Hamayun, M., Sohn, E. Y., Khan, S. A., Shinwari, Z. K., Khan, A. L. et al. (2010). Silicon alleviates the adverse effects of salinity and drought stress on growth and endogenous plant growth hormones of soybean (Glycine max L.). Pakistan Journal of Botany, 42(3), 1713–1722. [Google Scholar]
59. Zhang, Y., Liang, Y., Zhao, X., Jin, X., Hou, L. et al. (2019). Silicon compensates phosphorus deficit-induced growth inhibition by improving photosynthetic capacity, antioxidant potential, and nutrient homeostasis in tomato. Agronomy, 9(11), 733. [Google Scholar]
60. Fatemi, H., Pour, B. E., Rizwan, M. (2020). Isolation and characterization of lead (Pb) resistant microbes and their combined use with silicon nanoparticles improved the growth, photosynthesis and antioxidant capacity of coriander (Coriandrum sativum L.) under Pb stress. Environmental Pollution, 266, 114982. [Google Scholar] [PubMed]
61. Banerjee, A., Roychoudhury, A. (2016). Group II late embryogenesis abundant (LEA) proteins: Structural and functional aspects in plant abiotic stress. Plant Growth Regulation, 79, 1–17. [Google Scholar]
62. Banerjee, A., Wani, S. H., Roychoudhury, A. (2017). Epigenetic control of plant cold responses. Frontiers in Plant Science, 8, 1643. [Google Scholar] [PubMed]
63. Banerjee, A., Roychoudhury, A. (2017). Abscisic-acid-dependent basic leucine zipper (bZIP) transcription factors in plant abiotic stress. Protoplasma, 254, 3–16. [Google Scholar] [PubMed]
64. Ríos, J. J., Blasco, B., Cervilla, L. M., Rosales, M. A., Sanchez-Rodriguez, E. et al. (2009). Production and detoxification of H2O2 in lettuce plants exposed to selenium. Annals of Applied Biology, 154(1), 107–116. [Google Scholar]
65. Nowak, J., Kaklewski, K., Ligocki, M. (2004). Influence of selenium on oxidoreductive enzymes activity in soil and in plants. Soil Biology and Biochemistry, 36(10), 1553–1558. [Google Scholar]
66. Huang, H., Li, M., Rizwan, M., Dai, Z., Yuan, Y. et al. (2021). Synergistic effect of silicon and selenium on the alleviation of cadmium toxicity in rice plants. Journal of Hazardous Materials, 401, 123393. [Google Scholar] [PubMed]
67. Wu, Z., Xu, S., Shi, H., Zhao, P., Liu, X. et al. (2018). Comparison of foliar silicon and selenium on cadmium absorption, compartmentation, translocation and the antioxidant system in Chinese flowering cabbage. Ecotoxicology and Environmental Safety, 166, 157–164. [Google Scholar] [PubMed]
68. Greger, M., Landberg, T., Vaculík, M. (2018). Silicon influences soil availability and accumulation of mineral nutrients in various plant species. Plants, 7(2), 41. [Google Scholar] [PubMed]
69. Xu, S., Zhao, N., Qin, D., Liu, S., Jiang, S. et al. (2021). The synergistic effects of silicon and selenium on enhancing salt tolerance of maize plants. Environmental and Experimental Botany, 187, 104482. [Google Scholar]
70. Luís Oliveira Cunha, M., de Mello Prado, R. (2023). Synergy of selenium and silicon to mitigate abiotic stresses: A review. Gesunde Pflanzen, 1–14. https://doi.org/10.1007/s10343-022-00826-9 [Google Scholar] [CrossRef]
71. Fu, X., Mehmood, S., Ahmed, W., Ou, W., Suo, P. et al. (2023). Reducing chromium toxicity in Chinese cabbage through synergistic effects of silicon and selenium: A study of plant growth, chromium content, and biochemical parameters. Sustainability, 15(6), 5361. [Google Scholar]
72. Kapoor, B., Kumar, P., Gill, N. S., Sharma, R., Thakur, N. et al. (2022). Molecular mechanisms underpinning the silicon-selenium (Si-Se) interactome and cross-talk in stress-induced plant responses. Plant and Soil, 486(4), 45–68. [Google Scholar]
73. Lanza, M. G. D. B., Dos Reis, A. R. (2021). Roles of selenium in mineral plant nutrition: ROS scavenging responses against abiotic stresses. Plant Physiology and Biochemistry, 164, 27–43. [Google Scholar] [PubMed]
74. Khalid, M. F., Iqbal Khan, R., Jawaid, M. Z., Shafqat, W., Hussain, S. et al. (2022). Nanoparticles: The plant saviour under abiotic stresses. Nanomaterials, 12(21), 3915. [Google Scholar] [PubMed]
75. Cheng, B., Wang, C., Yue, L., Chen, F., Cao, X. et al. (2023). Selenium nanomaterials improve the quality of lettuce (Lactuca sativa L.) by modulating root growth, nutrient availability, and photosynthesis. NanoImpact, 29, 100449. https://doi.org/10.1016/j.impact.2022.100449 [Google Scholar] [PubMed] [CrossRef]
76. Fellet, G., Pilotto, L., Marchiol, L., Braidot, E. (2021). Tools for nano-enabled agriculture: Fertilizers based on calcium phosphate, silicon, and chitosan nanostructures. Agronomy, 11(6), 1239. [Google Scholar]
77. Danso, O. P., Asante-Badu, B., Zhang, Z., Song, J., Wang, Z. et al. (2023). Selenium biofortification: Strategies, progress and challenges. Agriculture, 13(2), 416. [Google Scholar]
78. Zahedi, S. M., Moharrami, F., Sarikhani, S., Padervand, M. (2020). Selenium and silica nanostructure-based recovery of strawberry plants subjected to drought stress. Scientific Reports, 10(1), 17672. [Google Scholar] [PubMed]
79. Li, Q., Xian, L., Yuan, L., Lin, Z., Chen, X. et al. (2023). The use of selenium for controlling plant fungal diseases and insect pests. Frontiers in Plant Science, 14, 1505. [Google Scholar]
80. Islam, W., Tayyab, M., Khalil, F., Hua, Z., Huang, Z. et al. (2020). Silicon-mediated plant defense against pathogens and insect pests. Pesticide Biochemistry and Physiology, 168, 104641. [Google Scholar] [PubMed]
81. Bano, I., Skalickova, S., Sajjad, H., Skladanka, J., Horky, P. (2021). Uses of selenium nanoparticles in the plant production. Agronomy, 11(11), 2229. [Google Scholar]
82. Abdullah, E. H. E., Misran, A., Yaapar, M. N., Yusop, M. R., Ramli, A. (2021). The potential of silicon in improving rice yield, grain quality, and minimising chalkiness: A review. Pertanika Journal of Tropical Agricultural Science, 44(3), 655–672. [Google Scholar]
83. Pareek, A., Sopory, S. K., Bohnert, H. J. (2009). Abiotic stress adaptation in plants. Dordrecht, The Netherlands: Springer. [Google Scholar]
84. Singh, A. K., Sopory, S. K., Wu, R., Singla-Pareek, S. L. (2010). Transgenic approaches. In: Abiotic stress adaptation in plants: Physiological, molecular and genomic foundation, pp. 417–450. Netherlands: Springer. [Google Scholar]
85. Sattar, A., Cheema, M. A., Sher, A., Ijaz, M., Ul-Allah, S. et al. (2019). Physiological and biochemical attributes of bread wheat (Triticum aestivum L.) seedlings are influenced by foliar application of silicon and selenium under water deficit. Acta Physiologiae Plantarum, 41, 1–11. [Google Scholar]
86. Sita, K., Sehgal, A., Kumar, S., Nayyar, H. (2022). Individual and combined effects of selenium and silica on enhancing the heat tolerance of lentil (Lens culinaris Medik.) genotypes. Plant Physiology Reports, 27(3), 481–497. [Google Scholar]
87. Tang, H., Liu, Y., Gong, X., Zeng, G., Zheng, B. et al. (2015). Effects of selenium and silicon on enhancing antioxidative capacity in ramie (Boehmeria nivea (L.) Gaud.) under cadmium stress. Environmental Science and Pollution Research, 22, 9999–10008. [Google Scholar] [PubMed]
88. Pavlovic, J., Kostic, L., Bosnic, P., Kirkby, E. A., Nikolic, M. (2021). Interactions of silicon with essential and beneficial elements in plants. Frontiers in Plant Science, 12, 697592. [Google Scholar] [PubMed]
89. González-García, Y., Cárdenas-Álvarez, C., Cadenas-Pliego, G., Benavides-Mendoza, A., Cabrera-de-la-Fuente, M. et al. (2021). Effect of three nanoparticles (Se, Si and Cu) on the bioactive compounds of bell pepper fruits under saline stress. Plants, 10(2), 217. [Google Scholar]
90. Xiang, J., Rao, S., Chen, Q., Zhang, W., Cheng, S. et al. (2022). Research progress on the effects of selenium on the growth and quality of tea plants. Plants, 11(19), 2491. [Google Scholar] [PubMed]
91. Golubkina, N., Zayachkovsky, V., Sheshnitsan, S., Skrypnik, L., Antoshkina, M. et al. (2022). Prospects of the application of garlic extracts and selenium and silicon compounds for plant protection against herbivorous pests: A review. Agriculture, 12(1), 64. [Google Scholar]
92. Aslam, R., Bostan, N., Nabgha-e-Amen, M. M., Safdar, W. (2011). A critical review on halophytes: Salt tolerant plants. Journal of Medicinal Plants Research, 5(33), 7108–7118. [Google Scholar]
93. Kronzucker, H. J., Britto, D. T. (2011). Sodium transport in plants: A critical review. New Phytologist, 189(1), 54–81. [Google Scholar] [PubMed]
94. Munns, R., Tester, M. (2008). Mechanisms of salinity tolerance. Annual Review of Plant Biology, 59, 651–681. [Google Scholar] [PubMed]
95. Ahmad, P., Prasad, M. N. V. (2011). Abiotic stress responses in plants: Metabolism, productivity and sustainability. USA: Springer Science & Business Media. [Google Scholar]
96. Bhatnagar-Mathur, P., Vadez, V., Sharma, K. K. (2008). Transgenic approaches for abiotic stress tolerance in plants: Retrospect and prospects. Plant Cell Reports, 27, 411–424. [Google Scholar] [PubMed]
97. Maathuis, F. J., Ahmad, I., Patishtan, J. (2014). Regulation of Na+ fluxes in plants. Frontiers in Plant Science, 5, 467. [Google Scholar] [PubMed]
98. Hasanuzzaman, M., Nahar, K., Fujita, M. (2013). Plant response to salt stress and role of exogenous protectants to mitigate salt-induced damages. In: Ecophysiology and Responses of Plants under Salt Stress, vol. 25–87. NY, USA: Springer. [Google Scholar]
99. Ahmad, P. (2011). Antioxidants: Oxidative stress management in plants. New Delhi, India: Studium Press. [Google Scholar]
100. Terry, N., Zayed, A. M., de Souza, M. P., Tarun, A. S. (2000). Selenium in higher plants. Annual Review of Plant Biology, 51(1), 401–432. [Google Scholar]
101. Kong, L., Wang, M., Bi, D. (2005). Selenium modulates the activities of antioxidant enzymes, osmotic homeostasis and promotes the growth of sorrel seedlings under salt stress. Plant Growth Regulation, 45, 155–163. [Google Scholar]
102. Diao, M., Ma, L., Wang, J., Cui, J., Fu, A. et al. (2014). Selenium promotes the growth and photosynthesis of tomato seedlings under salt stress by enhancing chloroplast antioxidant defense system. Journal of Plant Growth Regulation, 33, 671–682. [Google Scholar]
103. Habibi, G. (2014). Role of trace elements in alleviating environmental stress. In: Emerging technologies and management of crop stress tolerance, pp. 313–342. USA: Academic Press. [Google Scholar]
104. Hawrylak-Nowak, B. (2009). Beneficial effects of exogenous selenium in cucumber seedlings subjected to salt stress. Biological Trace Element Research, 132, 259–269. [Google Scholar] [PubMed]
105. Walaa, A. E., Shatlah, M. A., Atteia, M. H., Sror, H. A. M. (2010). Selenium induces antioxidant defensive enzymes and promotes tolerance against salinity stress in cucumber seedlings (Cucumis sativus). Arab Universities Journal of Agricultural Sciences, 18(1), 65–76. [Google Scholar]
106. Qin, L., Kang, W. H., Qi, Y. L., Zhang, Z. W., Wang, N. (2016). The influence of silicon application on growth and photosynthesis response of salt stressed grapevines (Vitis vinifera L.). Acta Physiologiae Plantarum, 38, 1–9. [Google Scholar]
107. Khan, W. U. D., Aziz, T., Maqsood, M. A., Farooq, M., Abdullah, Y. et al. (2018). Silicon nutrition mitigates salinity stress in maize by modulating ion accumulation, photosynthesis, and antioxidants. Photosynthetica, 56, 1047–1057. [Google Scholar]
108. Mishra, M., Wungrampha, S., Kumar, G., Singla-Pareek, S. L., Pareek, A. (2021). How do rice seedlings of landrace Pokkali survive in saline fields after transplantation? Physiology, biochemistry, and photosynthesis. Photosynthesis Research, 150, 117–135. [Google Scholar] [PubMed]
109. Zhu, Y. X., Xu, X. B., Hu, Y. H., Han, W. H., Yin, J. L. et al. (2015). Silicon improves salt tolerance by increasing root water uptake in Cucumis sativus L. Plant Cell Reports, 34, 1629–1646. [Google Scholar] [PubMed]
110. Filek, M., Łabanowska, M., Kościelniak, J., Biesaga-Kościelniak, J., Kurdziel, M. et al. (2015). Characterization of barley leaf tolerance to drought stress by chlorophyll fluorescence and electron paramagnetic resonance studies. Journal of Agronomy and Crop Science, 201(3), 228–240. [Google Scholar]
111. Kuznetsov, V. V., Kholodova, V. P., Kuznetsov, V. V., Yagodin, B. A. (2003). Selenium regulates the water status of plants exposed to drought. In: Doklady biological sciences, pp. c1965–c1992. New York: Consultants Bureau. [Google Scholar]
112. Proietti, P., Nasini, L., Del Buono, D., D’Amato, R., Tedeschini, E. et al. (2013). Selenium protects olive (Olea europaea L.) from drought stress. Scientia Horticulturae, 164, 165–171. [Google Scholar]
113. Emam, M. M., Khattab, H. E., Helal, N. M., Deraz, A. E. (2014). Effect of selenium and silicon on yield quality of rice plant grown under drought stress. Australian Journal of Crop Science, 8(4), 596–605. [Google Scholar]
114. Ahmad, R., Waraich, E. A., Nawaz, F., Ashraf, M. Y., Khalid, M. (2016). Selenium (Se) improves drought tolerance in crop plants-a myth or fact? Journal of the Science of Food and Agriculture, 96(2), 372–380. [Google Scholar] [PubMed]
115. Ibrahim, H. M. (2014). Selenium pretreatment regulates the antioxidant defense system and reduces oxidative stress on drought-stressed wheat (Triticum aestivum L.) plants. Asian Journal of Plant Sciences, 13(3), 120–128. [Google Scholar]
116. Yao, X., Chu, J., Wang, G. (2009). Effects of selenium on wheat seedlings under drought stress. Biological Trace Element Research, 130, 283–290. [Google Scholar] [PubMed]
117. Nawaz, F., Ahmad, R., Ashraf, M. Y., Waraich, E. A., Khan, S. Z. (2015). Effect of selenium foliar spray on physiological and biochemical processes and chemical constituents of wheat under drought stress. Ecotoxicology and Environmental Safety, 113, 191–200. [Google Scholar] [PubMed]
118. Nawaz, F., Naeem, M., Ashraf, M. Y., Tahir, M. N., Zulfiqar, B. et al. (2016). Selenium supplementation affects physiological and biochemical processes to improve fodder yield and quality of maize (Zea mays L.) under water deficit conditions. Frontiers in Plant Science, 7, 1438. [Google Scholar] [PubMed]
119. Sajedi, N. A., Ardakani, M. R., Madani, H., Naderi, A., Miransari, M. (2011). The effects of selenium and other micronutrients on the antioxidant activities and yield of corn (Zea mays L.) under drought stress. Physiology and Molecular Biology of Plants, 17, 215–222. [Google Scholar] [PubMed]
120. Dehghanipoodeh, S., Ghobadi, C., Baninasab, B., Gheysari, M., Shiranibidabadi, S. (2018). Effect of silicon on growth and development of strawberry under water deficit conditions. Horticultural Plant Journal, 4(6), 226–232. [Google Scholar]
121. Taha, R. S., Seleiman, M. F., Shami, A., Alhammad, B. A., Mahdi, A. H. (2021). Integrated application of selenium and silicon enhances growth and anatomical structure, antioxidant defense system and yield of wheat grown in salt-stressed soil. Plants, 10(6), 1040. [Google Scholar] [PubMed]
122. Rahman, M., Rahman, K., Sathi, K. S., Alam, M. M., Nahar, K. et al. (2021). Supplemental selenium and boron mitigate salt-induced oxidative damages in Glycine max L. Plants, 10(10), 2224. [Google Scholar] [PubMed]
123. Abideen, Z., Hanif, M., Munir, N., Nielsen, B. L. (2022). Impact of nanomaterials on the regulation of gene expression and metabolomics of plants under salt stress. Plants, 11(5), 691. [Google Scholar] [PubMed]
124. Semida, W. M., Abd El-Mageed, T. A., Abdelkhalik, A., Hemida, K. A., Abdurrahman, H. A. et al. (2021). Selenium modulates antioxidant activity, osmoprotectants, and photosynthetic efficiency of onion under saline soil conditions. Agronomy, 11(5), 855. [Google Scholar]
125. Wang, D., Hou, L., Zhang, L., Liu, P. (2021). The mechanisms of silicon on maintaining water balance under water deficit stress. Physiologia Plantarum, 173(3), 1253–1262. [Google Scholar] [PubMed]
126. Avila, R. G., Magalhães, P. C., da Silva, E. M., Gomes Júnior, C. C., de Paula Lana, U. G. et al. (2020). Silicon supplementation improves tolerance to water deficiency in sorghum plants by increasing root system growth and improving photosynthesis. Silicon, 12(11), 2545–2554. [Google Scholar]
127. Zhu, Y. X., Gong, H. J., Yin, J. L. (2019). Role of silicon in mediating salt tolerance in plants: A review. Plants, 8(6), 147. [Google Scholar] [PubMed]
128. Yadav, S. K. (2010). Cold stress tolerance mechanisms in plants. A review. Agronomy for Sustainable Development, 30(3), 515–527. [Google Scholar]
129. Abbas, S. M. (2012). Effects of low temperature and selenium application on growth and the physiological changes in sorghum seedlings. Journal of Stress Physiology & Biochemistry, 8(1), 268–286. [Google Scholar]
130. Haghighi, M., Abolghasemi, R., da Silva, J. A. T. (2014). Low and high temperature stress affect the growth characteristics of tomato in hydroponic culture with Se and nano-Se amendment. Scientia Horticulturae, 178(16), 231–240. [Google Scholar]
131. Kishor, P. K., Sangam, S., Amrutha, R. N., Laxmi, P. S., Naidu, K. R. et al. (2005). Regulation of proline biosynthesis, degradation, uptake and transport in higher plants: Its implications in plant growth and abiotic stress tolerance. Current Science, 88(3), 424–438. [Google Scholar]
132. Chu, J., Yao, X., Zhang, Z. (2010). Responses of wheat seedlings to exogenous selenium supply under cold stress. Biological Trace Element Research, 136(3), 355–363. [Google Scholar] [PubMed]
133. Habibi, G. (2016). Effect of foliar-applied silicon on photochemistry, antioxidant capacity and growth in maize plants subjected to chilling stress. Acta Agriculturae Slovenica, 107(1), 33–43. [Google Scholar]
134. Mathur, S., Agrawal, D., Jajoo, A. (2014). Photosynthesis: Response to high temperature stress. Journal of Photochemistry and Photobiology B: Biology, 137, 116–126. [Google Scholar] [PubMed]
135. Djanaguiraman, M., Prasad, P. V., Seppanen, M. (2010). Selenium protects sorghum leaves from oxidative damage under high temperature stress by enhancing antioxidant defense system. Plant Physiology and Biochemistry, 48(12), 999–1007. [Google Scholar] [PubMed]
136. Hasanuzzaman, M., Nahar, K., Alam, M. M., Fujita, M. (2014). Modulation of antioxidant machinery and the methylglyoxal detoxification system in selenium-supplemented Brassica napus seedlings confers tolerance to high temperature stress. Biological Trace Element Research, 161, 297–307. [Google Scholar] [PubMed]
137. Iqbal, M., Hussain, I., Liaqat, H., Ashraf, M. A., Rasheed, R. et al. (2015). Exogenously applied selenium reduces oxidative stress and induces heat tolerance in spring wheat. Plant Physiology and Biochemistry, 94, 95–103. [Google Scholar] [PubMed]
138. Jóźwiak, W., Politycka, B. (2019). Effect of selenium on alleviating oxidative stress caused by a water deficit in cucumber roots. Plants, 8(7), 217. [Google Scholar] [PubMed]
139. Huang, C. P., Huang, W. J., Liao, J. L. (2022). Selenium-and nano-selenium-mediated cold-stress tolerance in crop plants. In: Selenium and nano-selenium in environmental stress management and crop quality improvement. Cham: Springer International Publishing. [Google Scholar]
140. Kumar, R. R., Rai, G. K., Kota, S., Watts, A., Sakhare, A. et al. (2022). Fascinating dynamics of silicon in alleviation of heat stress induced oxidative damage in plants. Plant Growth Regulation, 45, 1–15. [Google Scholar]
141. Saha, G., Mostofa, M. G., Rahman, M. M., Tran, L. S. P. (2021). Silicon-mediated heat tolerance in higher plants: A mechanistic outlook. Plant Physiology and Biochemistry, 166, 341–347. [Google Scholar] [PubMed]
142. Khan, I., Awan, S. A., Rizwan, M., Brestic, M., Xie, W. (2022). Silicon: An essential element for plant nutrition and phytohormones signaling mechanism under stressful conditions. Plant Growth Regulation, 46, 1–19. [Google Scholar]
143. Hasanuzzaman, M., Fujita, M. (2013). Heavy metals in the environment: Current status, toxic effects on plants and phytoremediation. In: Anjum, N. A., Pereira, M. E., Ahmad, I., Duarte, A. C., Umar, S., Khan, N. A. (Eds.Phytotechnologies—remediation of environmental contaminants, pp. 7–73. Boca Raton, USA: CRC Press. [Google Scholar]
144. Gupta, M., Gupta, S. (2017). An overview of selenium uptake, metabolism, and toxicity in plants. Frontiers in Plant Science, 7, 2074. [Google Scholar] [PubMed]
145. Cartes, P., Jara, A. A., Pinilla, L., Rosas, A., Mora, M. L. (2010). Selenium improves the antioxidant ability against aluminium-induced oxidative stress in ryegrass roots. Annals of Applied Biology, 156(2), 297–307. [Google Scholar]
146. Filek, M., Zembala, M., Hartikainen, H., Miszalski, Z., Kornaś, A. et al. (2009). Changes in wheat plastid membrane properties induced by cadmium and selenium in presence/absence of 2,4-dichlorophenoxyacetic acid. Plant Cell, Tissue and Organ Culture, 96, 19–28. [Google Scholar]
147. Chauhan, R., Awasthi, S., Tripathi, P., Mishra, S., Dwivedi, S. et al. (2017). Selenite modulates the level of phenolics and nutrient element to alleviate the toxicity of arsenite in rice (Oryza sativa L.). Ecotoxicology and Environmental Safety, 138, 47–55. [Google Scholar] [PubMed]
148. Łabanowska, M., Bidzińska, E., Filek, M. (2010). Influence of cadmium and selenium on photosynthesis activity of rape and wheat plants studied by EPR. Current Topics in Biophysics, 33, 141–146. [Google Scholar]
149. Filek, M., Kościelniak, J., Łabanowska, M., Bednarska, E., Bidzińska, E. (2010). Selenium-induced protection of photosynthesis activity in rape (Brassica napus) seedlings subjected to cadmium stress. Fluorescence and EPR measurements. Photosynthesis Research, 105, 27–37. [Google Scholar] [PubMed]
150. Mozafariyan, M., Shekari, L., Hawrylak-Nowak, B., Kamelmanesh, M. M. (2014). Protective role of selenium on pepper exposed to cadmium stress during reproductive stage. Biological Trace Element Research, 160, 97–107. [Google Scholar] [PubMed]
151. Hu, Y., Norton, G. J., Duan, G., Huang, Y., Liu, Y. (2014). Effect of selenium fertilization on the accumulation of cadmium and lead in rice plants. Plant and Soil, 384, 131–140. [Google Scholar]
152. Pandey, C., Raghuram, B., Sinha, A. K., Gupta, M. (2015). miRNA plays a role in the antagonistic effect of selenium on arsenic stress in rice seedlings. Metallomics, 7(5), 857–866. [Google Scholar] [PubMed]
153. Sun, H., Dai, H., Wang, X., Wang, G. (2016). Physiological and proteomic analysis of selenium-mediated tolerance to Cd stress in cucumber (Cucumis sativus L.). Ecotoxicology and Environmental Safety, 133, 114–126. [Google Scholar] [PubMed]
154. Rehman, M. Z., Rizwan, M., Rauf, A., Ayub, M. A., Ali, S. et al. (2019). Split application of silicon in cadmium (Cd) spiked alkaline soil plays a vital role in decreasing Cd accumulation in rice (Oryza sativa L.) grains. Chemosphere, 226, 454–462. [Google Scholar] [PubMed]
155. Emamverdian, A., Ding, Y., Mokhberdoran, F., Xie, Y., Zheng, X. et al. (2020). Silicon dioxide nanoparticles improve plant growth by enhancing antioxidant enzyme capacity in bamboo (Pleioblastus pygmaeus) under lead toxicity. Trees, 34, 469–481. [Google Scholar]
156. Hossain, M. M., Khatun, M. A., Haque, M. N., Bari, M. A., Alam, M. F. et al. (2018). Silicon alleviates arsenic-induced toxicity in wheat through vacuolar sequestration and ROS scavenging. International Journal of Phytoremediation, 20(8), 796–804. [Google Scholar] [PubMed]
157. Ali, S., Farooq, M. A., Yasmeen, T., Hussain, S., Arif, M. S. et al. (2013). The influence of silicon on barley growth, photosynthesis and ultra-structure under chromium stress. Ecotoxicology and Environmental Safety, 89, 66–72. [Google Scholar] [PubMed]
158. Zhu, Y., Dong, Y., Zhu, N., Jin, H. (2022). Foliar application of biosynthetic nano-selenium alleviates the toxicity of Cd, Pb, and Hg in Brassica chinensis by inhibiting heavy metal adsorption and improving antioxidant system in plant. Ecotoxicology and Environmental Safety, 240, 113681. [Google Scholar] [PubMed]
159. Lai, X., Yang, X., Rao, S., Zhu, Z., Cong, X. et al. (2022). Advances in physiological mechanisms of selenium to improve heavy metal stress tolerance in plants. Plant Biology, 24(6), 913–919. [Google Scholar] [PubMed]
160. Sheng, H., Chen, S. (2020). Plant silicon-cell wall complexes: Identification, model of covalent bond formation and biofunction. Plant Physiology and Biochemistry, 155, 13–19. [Google Scholar] [PubMed]
161. Khan, I., Awan, S. A., Rizwan, M., Ali, S., Hassan, M. J. et al. (2021). Effects of silicon on heavy metal uptake at the soil-plant interphase: A review. Ecotoxicology and Environmental Safety, 222, 112510. [Google Scholar] [PubMed]
162. Flora, C., Khandekar, S., Boldt, J., Leisner, S. (2019). Silicon alleviates long-term copper toxicity and influences gene expression in Nicotiana tabacum. Journal of Plant Nutrition, 42(8), 864–878. [Google Scholar]
Cite This Article
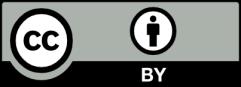
This work is licensed under a Creative Commons Attribution 4.0 International License , which permits unrestricted use, distribution, and reproduction in any medium, provided the original work is properly cited.