Open Access
REVIEW
The Mechanisms of Trichoderma Species to Reduce Drought and Salinity Stress in Plants
College of Environment and Surveying and Mapping Engineering, Suzhou University, Suzhou, 234000, China
* Corresponding Author: Haiyang Zhang. Email:
(This article belongs to the Special Issue: New Approaches to Mitigate Abiotic and Biotic Stresses for Improving Crop Productivity and Quality)
Phyton-International Journal of Experimental Botany 2023, 92(8), 2261-2281. https://doi.org/10.32604/phyton.2023.029486
Received 22 February 2023; Accepted 06 May 2023; Issue published 25 June 2023
Abstract
Environmental stresses caused by climate change have severely affected agriculture in the present century; Salinity and drought have challenged most forecasts for increased agricultural production in the past few decades, therefore, different methods that reduce the effect of these stresses on plants have attracted scientists’ attention. The effect of beneficial soil microorganisms on soil health and increasing plants’ resistance to stresses is one of the solutions that researchers have paid attention to. This study investigated how Trichoderma species can be affected by the molecular and morphophysiological mechanisms of plants and improve their salt and drought resistance. This study also studied the different Trichoderma species’ functions to get a better understanding of how they reduce salt and drought stresses. Furthermore, the findings of this study provide a clear path for future research to focus on the unknown aspects of Trichoderma species and find effective ways to boost crop production under environmental stresses.Keywords
Trichoderma species are saprophytic or endophytic fungi that are found in almost all natural ecosystems, including forests, agricultural soils, and desert soils [1–3]. Trichodermaspp. which grow on plants’ roots and the rhizosphere, are non-pathogenic fungi that colonize a variety of monocotyledonous and dicotyledonous plants [3–5]; Besides secreting secondary metabolites, they also produce hydrolytic enzymes that improve plant structure [6]. There are a number of commercial products based on Trichodermaspp., called plant protection products (PPPs), which are used in a wide range of crops (agricultural, horticultural, ornamental, as well as vegetables and fruits during post-harvest storage) [7]. The fungi, also known as opportunistic plant symbionts, colonize plant roots and improve soil fertility and texture, water-holding capacity, and plant growth, as well as plants’ systemic resistance to pathogens [8,9]. Additionally, Trichodermaspp. interacts with a wide range of soil microorganisms and inhibits many diseases of plants without being pathogenic to plants or humans [3,10,11].
The hyphae of Trichodermaspp. penetrate the root cortex and form appressoria-like structures, colonizing the root intercellular space of the root vascular tissue; Eventually, force the surrounding plant cells to precipitate the cell wall material and establish a chemical relationship with plant compounds [3,4,11]. At the beginning of the third seed germination stage, Trichodermaspp. establish a symbiotic relationship with the host plant, and this relationship continues until the plant matures [5]. When Trichodermaspp. colonizes plant roots, they release viral-like genes products, enzymes, and low molecular weight compounds, reprogramming plant genes and proteomes and altering plant responses to the environment [5,12]; Besides colonizing plant roots, fungi also cause the expression of genes in leaves and stems [5].
As a plant growth promoter, Trichodermaspp. has become increasingly popular in recent years, and numerous studies have demonstrated that it increases plant growth throughout all growth stages [5,13]. Studies have shown that Trichoderma species improves root length, plant height, leaf surface, and dry weight of cucumber, millet, Luffa, bitter gourd (Momordica charantia L.), and Arabidopsis thaliana [5]. With Trichodermaspp. as biofertilizers, chemicals can be reduced and sustainable farming can be improved [14,15]; In this way, they can maintain soil conditions, rehabilitate land, and protect rare seeds while enhancing the health of humans and preserve the environment [13,16] (Fig. 1).
Figure 1: Involved effectors in the interaction between Trichoderma and plants. Nomenclature was proposed by Ramírez-Valdespino et al. [33]. SSCPs: Small Secreted Cysteine-Rich Proteins
In recent decades, it has been demonstrated that Trichodermaspp. improves plant growth and development by increasing its ability to absorb nutrients and water, and regulating phytohormones like ethylene, abscisic acid (ABA), cytokinin (CK), Indole-3-acetic acid (IAA, 3-IAA), Gibberellin (GA), and Zeatin [1,5,11,17–21]. As well, Trichodermaspp. activates nutrients in the soil by increasing the soil-rhizosphere contact zone and secreting extracellular enzymes including phosphatase, urease, sucrase, and organic acids [3,14,22]. Ramrez-Pimentel et al. found that Trichodermaspp. improved soil phosphorus bioavailability and plant availability of phosphorus [23], and additionally, Harman’s study demonstrated that Trichoderma spp. reduced nitrogen (N) fertilizer use by about 40% to 50% [24]. In general, seed coating with Trichodermaspp. increases seed germination, plant growth, and seedling establishment significantly [13] (Fig. 2).
Figure 2: Positive effects of inoculation of plant roots with Trichodermaspp.
Trichodermaspp. is the most useful fungi for protecting plant products in organic systems due to its antagonistic properties [12,25]. It has recently been discovered that Trichodermaspp. is an effective biological control agent that helps plants resist both biotic (pests and pathogens) and abiotic stresses (salinity, high and low temperatures, droughts, heavy metals, etc.) [26–29]. Studies have also shown that Trichoderma species are effective at controlling plant pathogens, including Pythium, Fusarium, Rhizoctonia, Phytophthora, Sclerotium, Botrytis, Colletotrichum, and Armillaria [6,15,23,30,31]. In general, Trichodermaspp. play a crucial role in increasing the resistance of plants to various stresses by increasing photosynthetic efficiency, stimulating root growth, improving water and nutrient absorption, removing reactive oxygen species (ROSs), using chemical mutagens or T-DNA mutagenesis, producing alamethicins, producing metabolites, induce Hydrolytic enzymes genes expression including the endochitinase gene (ech33), and producing a trichodermin-trichothecene-type terpene toxin [7,12,13,23,27,32].
When plants are exposed to abiotic stresses, ROSs (including H2O2, OH−, and O2−) are increased in different organs, and plants attempt to reduce ROS by implementing different techniques, including increasing the non-enzymatic and enzymatic antioxidants in plant organs [5,34]. It was found that Trichodermaspp. inoculation increased levels of glutathione reductases (GR), glutathione S-transferases (GST), superoxide dismutases (SOD), peroxidases (POD), and catalases (CAT) [5]. In response to diverse abiotic and biotic stresses, plants also regulate the opening and closing of their stomata with plant hormones such as Ethylene (ET), Jasmonic acid (JA), Salicylic acid (SA), and ABA [12]. Researchers have found that ABA preferentially signals abiotic stresses, while SA, JA, and ET signal pathogen and pest responses [12,35,36]. Studies have also shown that inoculating plant roots with Trichodermaspp. stimulates the expression of defense response genes, including Pathogenesis-related (PR) genes and proteinase inhibitor II gene [12,36,37] (Fig. 2; Table 1).
In recent years, specific genes from Trichodermaspp. have been identified and transferred to plants, increasing their resistance to biotic and abiotic stresses, among them, genes coding for aquaporins (AQPs), which play a crucial role in physiological processes and responses to a wide range of stresses [38–40]. AQPs belong to the major intrinsic protein (MIP) family and are essential for maintaining Cell Turgor, repairing xylem embolisms, regulating transpiration, regulating water balance, cell elongation, hydraulic conductance of root and leaf, and stomatal movements [6,41,42]. Research on transgenic Arabidopsis, tobacco, and rice with overexpressed aquaporin genes indicates that these plants are more resistant to various stresses [6,39].
Nomenclature was proposed by Pacheco-Trejo et al. [43].
Many studies have been conducted on Trichoderma species in recent years to understand how they increase plant resistance to biotic and abiotic stresses [44]; The most important types of Trichoderma are T. atroviride, T. coningii, T. reesei, T. ghanense, T. viride, and T. harzianum [45]. As an antagonistic mycobacterium, T. harzianum colonizes both monocotyledonous and dicotyledonous roots [46]; T. Harzianu is widely used as a biological fertilizer due to its beneficial effects on plants, including improving their structures as well as increasing their resistance to pathogens such as Fusarium solani, Rhizoctonia solani and Botrytis cinerea [47–50]. In addition to improving seed germination and reducing adverse effects of abiotic stresses on seeds, T. harzianum facilitates the absorption of nutritional compounds such as iron (Fe), manganese (Mn), phosphorus (P), copper (Cu), nitrogen (N), and sodium (Na), by improving the ability to dissolve them [5,23,29] (Fig. 2; Table 2).
It has been found that the colonization of roots by T. harzianum regulates hormones, antioxidants, phytoalexins, enzymes, and phenolic compounds (such as IAA, ABA, POD, β-1,3-Glucanases, Chitinases, and Lipoxygenases) [5,21,23,51]. According to Afifi et al., colonization of wheat roots by T. virnes, T. hariziunm, and T. viridie improves photosynthetic pigments and, consequently, total photosynthesis and proline production [13]; Moreover, Sekmen Cetinel et al. reported that T. virens and T. harzianum increased antioxidant enzymes such as CAT, SOD, and POX in various plant organs [52]. Research shows that T. asperellum colonization of tomato roots results in improved plant growth, organic fertilizer replacement, and biotic stress resistance [53] (Table 2).
Nomenclature was proposed by [54–72].
Meanwhile, several studies have been conducted on the effects of simultaneous inoculation of soil microorganisms on plants in recent years [22,32,73,74]; Li et al. reported in their study that simultaneous inoculation of Glomusspp., Trichodermaspp., and Bacillusspp. not only protected plants more effectively against pathogenic microbes but also stimulated plant defense mechanisms in adverse environments [22]. Study results showed that the simultaneous application of arbuscular mycorrhizal fungi (AMF) and Trichodermaspp. increased the antioxidant capacity of Pinus sylvestris [75]. Researchers have also found that the simultaneous application of AMF and Trichodermaspp. improves plant biomass, biochemical parameters, nutrient levels, and soil-borne disease resistance [76–78]. This study aims to determine investigation the action mechanism of Trichodermaspp. in plants to obtain a general understanding of how Trichoderma species affects plant performance under salt and drought conditions.
Drought is one of the major abiotic stresses that can reduce crop yield more than all plant diseases combined worldwide [79,80]. As the global population has grown rapidly in recent decades, and crops need to be produced at about 60% more by 2050 to maintain human nutrition standards, the production of crops must be increased by using new technologies; On the other hand, it is also important to investigate the role of environmental stresses, particularly drought stress, in reducing crops production [5,29,81,82]. In general, drought is considered a stressful condition when the soil lacks water, causing an increase in soil temperature (especially during the day), a decrease in the availability of nutrients, and, ultimately, an increase in soil salinity [8,22]; And the risk of drought stress increases in soils with low fertility, such as those with sandy textures [8].
The most significant factor in the spread of drought in recent years has been climate change, which has shown its adverse effects in the subtropical and tropical regions of the world, resulting in unpredictable rainfall patterns [3,29]; Consequently, some areas experience flooding, while others experience a lack of rainfall, and in general, climate change has contributed to higher summer temperatures and decreased rainfall, which negatively affect crops and soil fertility [29,83,84]. As a result of global warming, air temperatures are predicted to rise by 2 degrees Celsius by the end of this century, and drought will affect 50% of agricultural lands by 2050 [29,85], and with the current trend of climate change by 2100, it seems that rice yield will fall by 20%–30%, corn yield will fall by 20%–45%, soybean yield will fall by 30%–60%, and wheat yield will drop by 5%–50% [29].
During drought stress, plants undergo irreversible changes in their morphology, anatomy, and physiology, which ultimately reduce their growth and productivity [7,8,86]. Water stress causes plants to lose their water content, turgor, and leaf water potential, and stop cell enlargement, resulting in a decrease in growth [87]. Drought stress also causes the leaves’ stomata to close, reducing photosynthesis, respiration, leaf surface, and length of the vegetative growth phase, ultimately leading to the plant’s death [29,86,88]. Furthermore, drought destroys soil structure, causing redox, reducing productivity, limiting root penetration, and limiting nutrients and water uptake [22] (Fig. 3).
Figure 3: Responses of plants to drought stress at different levels. NADP-ME: NADP malic enzyme, FBPase: fructose 1,6-bisphosphatase, PEPcase: phosphoenol pyruvate carboxylase, PPDKs: pyruvate phosphate dikinases, CIPKs: CBL interacting protein kinases, MAPKs: mitogen-activated protein kinases, SROs: similar to RCD-ONE, PPs: protein phosphatases, CDPKs: calcium-dependent protein kinases, ABA: abscisic acid, LEA: late embryogenesis abundant proteins, ROS: reactive oxygen species, SKIP: ski-interacting protein, SNAC: stress-responsive NAC transcription factor, SERF: serum response factor, ZFP: zinc finger transcription factor, DST: drought and salt tolerance. Nomenclature was proposed by Zargar et al. [100]
Crop yields under drought stress are affected by a variety of factors, such as genotype, growth and development stage of the plant, duration, and intensity of drought, and other environmental conditions [89]. In recent decades, scientists have been looking for drought-resistant cultivars using genetic modification, however, using management methods that reduce environmental stress also plays a major role in reducing drought stress [8,90]. In drought-stress conditions, it is essential to use beneficial soil microorganisms that are highly compatible with the environment is one of the best ways to improve soil fertility and stimulate plant growth [11,91,92]. Among the most critical soil microorganisms with a high degree of compatibility with the environment is Trichodermaspp., of the Hypocreaceae family [7,91]. The species of Trichoderma are found in many ecosystems around the world, and they can be used in a variety of ways, including seed priming and root colonization [29,90].
It is believed that Trichodermaspp. increases plant tolerance to drought stress by improving morphological, physiological, and biochemical structures [92], and these changes can be categorized in the following three ways: (1) maximizing plant water utilization efficiency, (2) reducing ROS damage, and (3) secreting phytohormones [5]. Studies have shown that the treatment of seeds by Trichodermaspp. increases the germination rate in water deficit conditions, and studies conducted on tomato and Arabidopsis have shown that germination percentages of seeds treated with T. harzianum were increased in osmotic potential up to 0.3 megapascals [5,93].
The researcher found that inoculating plants with Trichodermaspp. increases root growth, which increases the root’s access to water and nutrients, improving the water condition in various plant organs [5,7,27,46,94]; and through improving stomatal conduction increases plants’ efficiency of water consumption and tolerance to drought stress [8,95]. Research conducted on cocoa seedlings has shown that the colonization of roots by T. hamatum under drought stress increases root growth and improves water status in the plan [96]. Cornejo-Ríos et al. found that inoculating tomatoes with T. asperellum increased root growth and water efficiency [29], and similar results were also reported in corn, sugarcane, and rice inoculations by Trichodermaspp [5,7,8,92]. In general, priming seeds with Trichodermaspp. delays drought stress by 3–5 days [97].
As mentioned, inoculating plants with Trichodermaspp. improves stomatal conductance regulation, impacting transpiration, carbon dioxide absorption, and photosynthesis [8,95,98]. Several studies have shown that various species of Trichoderma, including T. viridie, T. asperelloides, T. virnes, and T. hariziunm, improve the quality of photosynthesis in plants [13,46,94], by improving the quality and content of photosynthetic pigments, this increases plant growth and immunity [7,29,92]. Additionally, Trichodermaspp. inoculation has been shown to enhance various plant organ growth and biomass production under drought-stress conditions [5,98,99]. In several studies conducted on tomatoes, it has been shown that Trichodermaspp. improves the synthesis of chlorophyll pigments, growth of length and weight of different organs, electrolyte leakage, nutrient absorption, biomass, and plant performance in drought conditions [27,29,94]. Estévez-Geffriaud et al. also showed in their study on corn that T. asperellum improved photosynthetic factors, relative water content, and seed weight and numbers under drought stress [7]. Mishra et al. found that Trichodermaspp. boosts chlorophyll accumulation, root and shoot growth, tiller number, and crop yield in rice under drought conditions [92]; Additionally, studies on mung bean and sugarcane have shown that Trichodermaspp. inoculation improves plant morphology and physiological factors under drought stress [8,86].
As a result of reducing oxidative stress, Trichodermaspp. increases plants’ resistance to drought stress [5,101]. Studies have shown that inoculating plant roots with Trichodermaspp. increases the expression of enzymatic and non-enzymatic antioxidant genes, reduces ROSs, and reduces the amount of lipid peroxide in plant seedlings [44,46,102]. A study conducted on tomato has shown that root colonization by Trichoderma atroviride ID20G (Ta) not only increases the activity of antioxidant enzymes such as APX, GPX, CAT, and SOD, but also greatly reduces the level of MDA and Hydrogen peroxide, increasing tomato seedling resistance to drought stress [44]. Several studies on rice have also shown that Trichodermaspp. increases the expression of antioxidant enzyme genes, and inoculating rice seedlings with T. harzianum under drought stress increases the activity of antioxidant enzymes, such as POD, CAT, SOD, and APX [5,103,104]. A similar study on wheat has also shown that inoculation by T. spirale, T. virens, and T. simmonsii inhibits ROS formation in drought conditions and activates the mechanism of antioxidant enzymes [22]; Similar results have also been reported for cucumbers and maize [5,105].
The colonization of plant roots by Trichodermaspp. regulates the secretion of endogenous plant hormones, including auxin, ASA, SA, JA, ET, and ABA [12,22,99]. Some studies on the effects of Trichodermaspp. inoculation on different plant organs has indicated a decrease in the expression of ABA synthesis marker genes [ABA is under the control of genes encoding the enzyme 9-cis-epoxycarotenoid dioxygenase (NCED)], and ABA content, which may be an adaptive advantage since it inhibits plant growth when conditions are droughty [5,12,106]. Trichodermaspp., on the other hand, inhibits plant production of ACC deaminase (ACCD), which plays an essential role in ET biosynthesis and may decrease plant root growth under drought stress [107]. In a study on tomatoes inoculated with T. brevicompactum, the JA and SA marker genes were positively regulated in the roots and the leaves, respectively, although ABA content in the roots increased slightly [12]. Further, studies conducted on tomato seedlings under drought stress have found that root inoculation with T. harzianum improves the regulation of proline, phenol, flavonoid, indole butyric acid (IBA), IAA, ethylene, and GA contents [27,108]. As a result of the study on sugarcane, researchers confirmed that, in addition to increasing proline concentration, T. asperellum also increases sugar division and, thus, increases root and stem growth under drought stress [8].
According to a study conducted on rice under drought stress, root inoculation by T. harzianum caused the expression of 70% of genes, most of which are related to metabolic pathways, secondary metabolites, carbon cycling, phenylpropanoid biosynthesis, and glutathione biosynthesis [46]. It appears that T. harzianum plays a major role in delaying the effects of drought stress in rice. The simultaneous inoculation of Trichodermaspp. with other beneficial soil microorganisms may improve the performance of plants under stress [86]. For example, in studies conducted on Astragalus mongholicus under drought stress, it was shown that inoculating Trichodermaspp. simultaneously with Dark septate endophytes (DSE), Paraboeremia putaminum, or Paraphoma radicina, in addition to improving the plant’s morphophysiology (such as improving root growth, antioxidant enzymes, and growth hormones), can also affect the soil environment (such as changes in the composition of the rhizosphere microbiome and improving the condition soil nutrients) [11,22]. In a study conducted on mung bean (Vigna radiata) under drought stress, it was also observed that the simultaneous use of Mycorrhiza, Rhizobium, and Trichoderma, in addition to improving the total soluble protein, increased the leaf area and internode length compared to the separate application of each of the microorganisms [86].
The degradation of soil quality is one of the main causes of land degradation, affecting hydraulic, erosion, geochemical, and biological cycles [14]. Salinity is one of the factors that directly affect the biological, chemical, and physical properties of soil and can directly destroy its texture and structure, as well as reduce its fertility [14,109]. Salinity is a major concern around the world, especially in arid and semi-arid regions, as it causes agricultural lands to become barren [110,111]. Approximately 7% of the world’s land has been affected by salinity, including 45 million hectares of cultivated land (20%), and 30% of all agricultural land is predicted to be affected under severe salinity stress by 2050 [25,112,113].
Recent decades have seen severe soil salinity in several areas, including India, Java Island, and Mediterranean countries, particularly the southeast of Spain [14,32,114,115]. Soil salinity has been elevated as a result of rising sea levels, irrigation by salty water, prolonged droughts, heavy rains, and improper land management [10,14,110,114,115]. Soil salinity, along with the proliferation of various diseases in the region, is a serious threat to agricultural production and environmental sustainability, which in severe cases leads to the abandonment of agricultural lands [14,25,116].
Salinity is one of the most common and harmful abiotic stresses, causing take in excessive sodium (Na+) and chloride (Cl−) ions by plants [52,117,118]. In addition to disrupting metabolism, it causes physiological, morphological, molecular, and biochemical changes in plants, and may even lead to death if it continues [1,2,32]. Salinity-sensitive plants cannot tolerate high concentrations of NaCl in the soil, and their behavior in a saline environment depends on their growth stage and the duration of their exposure to salt [32,119]. Plants are more sensitive to saline soil in the stage of seedling growth and early vegetative growth [32,120], and salinity generally reduces seed germination, seedling growth, number of leaves, fresh and dry weight of the plant, vegetative and reproductive growth, and lastly weakens the plant [21,25,118,121]. In a study conducted on Suaeda salsa, the branch number, the stem diameter, the length of the branch, and the plant height decreased as the NaCl content increased [122]. Similar results have been reported in the case of tomato, which has an average level of salt tolerance [32,123].
Increased salt concentrations affect various plant processes, such as photosynthetic pathways and pigments, protein content, respiration, metabolism of nitrogen and carbon, and absorption of water and nutrients, which lead to plant senescence and reduced yields [1,21,25,32,124]. In addition to blocking the phosphorylating and non-phosphorylating electron transfer pathways, the increase in salt concentration alters the osmotic pressure, and the processes of balance, uptake, and transport of ions, disrupting membrane function and causing cell dehydration [2,32,125]. In general, salinity stress affects water absorption, water use efficiency, leaf water potential, and transpiration rate, and disrupts plant metabolism [1,2] (Fig. 4).
Figure 4: The effect of soil salinity on the morphophysiological factors of plants. Nomenclature was proposed by Otlewska et al. [113]
Plants exposed to saline stress produce ROS, which causes oxidative stress, resulting in damage to essential molecules, such as proteins, carbohydrates, lipids, and even nucleic acids [52,126–128]. In the studies conducted on maize, it has been determined that salinity, in addition to reducing plant yield by about 20% to 40%, causes the production of large amounts of reactive oxygen species, which, further to the mentioned cases, disrupts the activity of intracellular enzymes and increases the content of thiobarbituric acid reactive substances (TBARS) [129,130]. Also, the reduction of plant endurance due to salinity makes plants more susceptible to various diseases, such as Fusarium oxysporum, Alternaria solani, and Phytophthora [32] (Fig. 4).
Plants have some natural solutions to increase their resistance to salinity, including an increase in enzymes (POX, SOD, APX, GR, CAT, and POD) and non-enzymatic (Carotenoids, Flavonoids, Phenolic compounds, Ascorbate, and GSH) antioxidants in various organs to reduce ROS production [32,127,129,131,132]. On the other hand, plants reduce osmotic potential and water potential by accumulating ions and osmolytes (Glycine, Proline, Betaine, and other amino acids), which improves water absorption by cells while also removing accumulated ROSs [2,21,32,133]. Furthermore, plants increase their resistance to salinity by selectively absorbing Na+ and Cl− from the medium or by accumulating and separating these ions in vacuoles [2].
In the past decade, several strategies have been used to reduce the effects of salinity stress on plants and improve saline soils, including irrigation with fresh water, cultivating salinity-resistant cultivars, using organic fertilizers, using mineral fertilizers (nitrogen, gypsum, potassium, and sulfur) and mulching [14,76,114]. However, due to the high cost, time-consuming, and relatively low beneficial effects, scientists have been investigating new solutions that will have a permanent impact, be less expensive, and be harmonious with the environment [76]. One of the best solutions is manipulating the rhizosphere of plants using beneficial soil microorganisms, which in addition to improving plant growth, improve mineral and water absorption by plants [1,76,114,134]. Trichodermaspp. is known as a key symbiont for plants in saline conditions, and it has been studied in recent years for its benefits in reducing salt stress on plants [32,135,136].
Researchers have identified the positive effects of Trichodermaspp. on a variety of plants including wheat, corn, rice, soybean, cucumber, tomato, and French bean when exposed to salinity stress [1,114,129]. However, the growth and reproduction of Trichodermaspp. are affected by different environmental conditions, especially high salt concentration [1,14,109,137], thus, finding salt-resistant species of Trichoderma is crucial to the advancement of agricultural projects based on microorganisms. In a study conducted on Trichodermaspp., it was found that T. asperellum is more resistant to environmental salinity than T. virens [1]. And a study conducted in India found that T. asperellum, among all Trichoderma species isolated from the soils of eastern Uttar Pradesh, has the highest resistance to salinity and high temperatures [25].
As a result of studies done on cultivated plants under salt stress, it has been shown that inoculating plants with Trichodermaspp. improves seed germination and stimulates the growth of different plant organs, as well as improving plant productivity [2,129,135]. Studies conducted on corn grown under salt stress have shown that inoculating plants with T. asperellum and T. harzianum, in addition to increasing the production of IAA, improves germination percentage, seedling establishment, length of shoot and root, leaf area, fresh and dry weights, as well as total biomass [21,109,129]. The inoculation of peanuts cultivated in the saline medium by T. harzianum, T. asperellum, and T. virens also showed an increase in IAA production, germination, shoot growth, biomass, and minimizing plant death, which indicates that Trichodermaspp. reduces salinity stress in peanut vegetative and reproductive growth [1,114].
Inoculation of plants with Trichodermaspp. enhances their antioxidant defense system by modifying the expression of genes related to oxidative stress and thus, increases the resistance of plants against salinity [2,129,135]. In their study on strawberries cultivated under salt stress, Sekmen Cetinel et al. found that T. citrinoviride treatment reduces hydrogen peroxide and lipid peroxidation as well as increases the activity of POX and SOD antioxidant enzymes [52]. Yasmeen et al. also found that T. harzianum treatment improved the morpho-physiological structure of rice seedlings and also reduced the destructive effects of ROSs in rice under salt stress through the activity of antioxidants (CAT and SOD) [118]. Studies conducted on maize showed that T. asperellum and T. harzianum reduced MDA accumulation in different organs of plants under salt stress, which demonstrated that these fungi reduce ROS levels [21,129].
Furthermore, Trichodermaspp. reduces the effects of salinity and improves the morpho-physiological and biochemical structures of plants, improving the absorption of water and nutrients, photosynthesis, and hormonal function [32,118,129]. The inoculation of corn with T. harzianum and T. asperellum improves photosynthetic processes and increases Ca2+ and K+ content in seedlings, thereby reducing Na+ content. These fungi also enhance the accumulation of osmolytes, which improves water absorption of cells, and they also enhance the activity of enzymes and non-enzymes antioxidants that reduce salt-induced oxidative damage [109,118,129]. A study conducted on Cucurbita pepo found that T. harzianum and T. viride treatments, in addition to increasing the synthesis of photosynthetic pigments, anthocyanins, carotenoids, GSH, proteins, and proline, enhanced water absorption and membrane stability, improving plant performance under salinity conditions [2].
The studies conducted on tomatoes and rice under salt stress found that T. harzianum increases the proline, flavonoids, and phenols content of the plant in addition to improving photosynthetic performance, water, and nutrition absorption [32,114,118]. According to studies done on strawberries (T. citrinoviride) and peanuts (T. virens and T. asperellum), Trichodermaspp. increases the photosynthetic efficiency and enzymatic and non-enzymatic antioxidants in saline environments [1,52]. It has been shown that using Trichodermaspp., including T. harzianum and T. asperellum, can significantly reduce soil pH and salt ions content, as well as improve the proportion of useful soil fungi (such as Trichoderma and Ceratobasidium), and the composition of the soil fungal community, thus improving maize yield and growth [14,109].
Additionally, Trichodermaspp. is capable of reducing the risk of other biotic and abiotic stresses at the same time as improving plant structures under salt stress [118,135]. Singh et al. stated that T. asperellum could be used for biological control of plant pathogens including Sclerotinia sclerotiorum, Colletotrichum capsici, Scletotium rolfsii, Rhizoctonia bataticola, and Fusarium oxysporum, as well as enhance plant resistance to salt stress [25]. Furthermore, the studies conducted on tomatoes have shown that T. harzianum, T. asperelloides, and T. arenarium sp. nov. can be used as a biocontrol method for Fusarium oxysporum, in addition to improving seedling resistance to salinity [10,32]. In their studies on strawberries, Sekmen Cetinel et al. discovered that inoculation of seedlings with T. citrinoviride by improving antioxidant enzyme activity, stimulating growth, reducing hydrogen peroxide accumulation, and improving photosynthesis can improve plant resistance to salinity as well as reducing the risk of Rhizoctonia solani infection [52]. Moreover, in a study on sorghum-sudangrass under the simultaneous stress of salinity and bauxite residues (red mud; a byproduct of alumina production), it was discovered that the inoculation of seedlings with T. asperellum improved the production of siderophore, IAA, phosphorus bioavailability, ACC deaminase, chlorophyll content, and biomass, which made the plants more resistant to both environmental stresses [135].
Although there have been not many studies on the combination of Trichodermaspp. with other factors that improve the soil environment, these few studies also indicate that when used in conjunction with other soil-improving factors, Trichodermaspp. can reduce salinity stress even more effectively [14,32]. In addition to increasing water penetration, stability of aggregates, salt leaching acceleration, and water storage capacity, organic matter can also reduce soil electrical conductivity and exchangeable sodium. Mbarki et al. reported in their study that the simultaneous use of T. harzianum and compost in soil not only affected soil microbiology and saline soil modification but also caused a relative increase in this particular strain of Trichoderma [14]. In their study on tomato seedlings, Kasyap et al. found that when T. harzianum and Hypocrea are applied simultaneously, T. harzianum adapts more easily to saline environments, and also increases tomato resistance to root rot disease, as well as reducing the risk of salinity stress [32]. Additionally, Yang et al. found that simultaneous inoculation of roots with Glomus sp. and T. longibrachiatum not only enhanced biomass but also improved K+/Na+ ratio in seedlings and increased AMF growth in the rhizosphere of maize [76].
The present study found that Trichodermaspp. improves physiological, morphological, and biochemical characteristics of plants that are subjected to drought and salinity stress, Nevertheless, further studies are needed to determine how different strains of Trichoderma affect plants under different stresses. The study also revealed that some Trichoderma species have a higher sensitivity to stresses, therefore, more in-depth research should be conducted on the effects of these stresses on different Trichoderma species, so that more suitable species can be selected for use in fields. Additionally, the results of this study indicated that simultaneous inoculation of different species of Trichoderma with other microorganisms could increase plant resistance to salt stress and drought stress, however, more studies on simultaneous inoculation of Trichoderma species with other fungi and bacteria are needed because of the limitations of current knowledge. The increasing number of studies on the effects of Trichodermaspp. on plant molecular structures, as well as the expansion of field studies, allows us to examine in greater detail how Trichodermaspp. affects plants under salinity and drought stress, and the extent to which it improves plant performance in field conditions.
Funding Statement: This work was supported by the Anhui Province Large-Scale Online Open Course (MOOC) Demonstration Project (2018mooc428); the Quality Engineering Project of Suzhou University: College of Geographic Information and Energy Agriculture Modern Industry (szxy2021cyxy06); the Key Project of Quality Engineering in Anhui Province (2021jyxm1499); the Scientific Research Platform Project of Suzhou University: Research Center of Non-Point Source Pollution Control and Ecological Remediation (2021XJPT11).
Author Contributions: MRB wrote the manuscript. MRB and HYZ edited the manuscript. All authors read and approved the final version of the manuscript.
Conflicts of Interest: The authors declare that they have no conflicts of interest to report regarding the present study.
References
1. Yusnawan, E., Taufiq, A., Wijanarko, A., Susilowati, D. N., Praptana, R. H. et al. (2021). Changes in volatile organic compounds from salt-tolerant Trichoderma and the biochemical response and growth performance in saline-stressed groundnut. Sustainability, 13(23), 13226. [Google Scholar]
2. Soliman, M. H., Alnusaire, T. S., Abdelbaky, N. F., Alayafi, A. A., Hasanuzzaman, M. et al. (2020). Trichoderma-induced improvement in growth, photosynthetic pigments, proline, and glutathione levels in Cucurbita pepo seedlings under salt stress. Phyton-International Journal of Experimental Botany, 89(3), 473–486. https://doi.org/10.32604/phyton.2020.08795 [Google Scholar] [CrossRef]
3. Harman, G. E., Howell, C. R., Viterbo, A., Chet, I., Lorito, M. (2004). Trichoderma species—Opportunistic, avirulent plant symbionts. Nature Reviews Microbiology, 2(1), 43–56. [Google Scholar] [PubMed]
4. Yedidia, I., Benhamou, N., Chet, I. (1999). Induction of defense responses in cucumber plants (Cucumis sativus L.) by the biocontrol agent Trichoderma harzianum. Applied and Environmental Microbiology, 65(3), 1061–1070. [Google Scholar] [PubMed]
5. Chepsergon, J., Mwamburi, L., Kassim, M. K. (2014). Mechanism of drought tolerance in plants using Trichodermaspp. International Journal of Science and Research, 3(11), 1592–1595. [Google Scholar]
6. Vieira, P. M., Santos, M. P., Andrade, C. M., Souza-Neto, O. A., Ulhoa, C. J. et al. (2017). Overexpression of an aquaglyceroporin gene from Trichoderma harzianum improves water-use efficiency and drought tolerance in Nicotiana tabacum. Plant Physiology and Biochemistry, 121, 38–47. [Google Scholar] [PubMed]
7. Estévez-Geffriaud, V., Vicente, R., Vergara-Díaz, O., Narváez Reinaldo, J. J., Trillas, M. I. (2020). Application of Trichoderma asperellum T34 on maize (Zea mays) seeds protects against drought stress. Planta, 252(1), 1–12. [Google Scholar]
8. Scudeletti, D., Crusciol, C. A. C., Bossolani, J. W., Moretti, L. G., Momesso, L. et al. (2021). Trichoderma asperellum inoculation as a tool for attenuating drought stress in sugarcane. Frontiers in Plant Science, 12, 645542. [Google Scholar] [PubMed]
9. Ahmad, P., Hashem, A., Abd-Allah, E. F., Alqarawi, A., John, R. et al. (2015). Role of Trichoderma harzianum in mitigating NaCl stress in Indian mustard (Brassica juncea L) through antioxidative defense system. Frontiers in Plant Science, 6, 868. [Google Scholar] [PubMed]
10. Ding, M. Y., Chen, W., Ma, X. C., Lv, B. W., Jiang, S. Q. et al. (2021). Emerging salt marshes as a source of Trichoderma arenarium sp. nov. and other fungal bioeffectors for biosaline agriculture. Journal of Applied Microbiology, 130(1), 179–195. [Google Scholar] [PubMed]
11. He, C., Liu, C., Liu, H., Wang, W., Hou, J. et al. (2022). Dual inoculation of dark septate endophytes and Trichoderma viride drives plant performance and rhizosphere microbiome adaptations of Astragalus mongholicus to drought. Environmental Microbiology, 24(1), 324–340. [Google Scholar] [PubMed]
12. Racić, G., Vukelić, I., Prokić, L., Ćurčić, N., Zorić, M. et al. (2018). The influence of Trichoderma brevicompactum treatment and drought on physiological parameters, abscisic acid content and signalling pathway marker gene expression in leaves and roots of tomato. Annals of Applied Biology, 173(3), 213–221. [Google Scholar]
13. Afifi, H. H., Hassan, N. A., Abou-hadid, A. F., Madkour, M. A. (2021). The ability of selected Trichodermaspp. to improve wheat (Triticum asetivum L.). Plant Cell Biotechnology and Molecular Biology, 22(47–48), 61–74. [Google Scholar]
14. Mbarki, S., Cerdà, A., Brestic, M., Mahendra, R., Abdelly, C. et al. (2017). Vineyard compost supplemented with Trichoderma harzianum T78 improve saline soil quality. Land Degradation & Development, 28(3), 1028–1037. [Google Scholar]
15. Braga, A. B., Costa, C. J., Pomella, A. W., Ribeiro, E. J., Santos, L. D. et al. (2019). Evaluation of lethality temperature and use of different wall materials in the microencapsulation process of Trichoderma asperellum conidias by spray drying. Powder Technology, 347(3), 199–206. [Google Scholar]
16. Sun, R., Dsouza, M., Gilbert, J. A., Guo, X., Wang, D. et al. (2016). Fungal community composition in soils subjected to long-term chemical fertilization is most influenced by the type of organic matter. Environmental Microbiology, 18(12), 5137–5150. [Google Scholar] [PubMed]
17. Simon, S., Petrášek, J. (2011). Why plants need more than one type of auxin. Plant Science, 180(3), 454–460. [Google Scholar] [PubMed]
18. Harman, G. E. (2000). Myths and dogmas of biocontrol changes in perceptions derived from research on Trichoderma harzinum T-22. Plant Disease, 84(4), 377–393. [Google Scholar] [PubMed]
19. Lee, S., Yap, M., Behringer, G., Hung, R., Bennett, J. W. (2016). Volatile organic compounds emitted by Trichoderma species mediate plant growth. Fungal Biology and Biotechnology, 3(1), 1–14. [Google Scholar]
20. Rawat, L., Singh, Y., Shukla, N., Kumar, J. (2012). Seed biopriming with salinity tolerant isolates of Trichoderma harzianum alleviates salt stress in rice: Growth, physiological and biochemical characteristics. Journal of Plant Pathology, 94(2), 353–365. [Google Scholar]
21. Kumar, K., Manigundan, K., Amaresan, N. (2017). Influence of salt tolerant Trichodermaspp. on growth of maize (Zea mays) under different salinity conditions. Journal of Basic Microbiology, 57(2), 141–150. [Google Scholar] [PubMed]
22. Li, M., Ren, Y., He, C., Yao, J., Wei, M. et al. (2022). Complementary effects of dark septate endophytes and Trichoderma strains on growth and active ingredient accumulation of Astragalus mongholicus under drought stress. Journal of Fungi, 8(9), 920. [Google Scholar] [PubMed]
23. Ramírez-Pimentel, J. G., Aguirre-Mancilla, C. L., Raya-Pérez, J. C., Acosta-García, G., Iturriaga, G. (2021). Trichoderma harzianum mutants enhance antagonism against phytopathogenic fungi, phosphorus assimilation and drought tolerance in Jalapeño pepper plants. Chilean Journal of Agricultural Research, 81(3), 270–280. [Google Scholar]
24. Harman, G. E. (2011). Multifunctional fungal plant symbionts: New tools to enhance plant growth and productivity. New Phytologist, 189(3), 647–649. [Google Scholar] [PubMed]
25. Singh, V., Keswani, C., Ray, S., Upadhyay, R. S., Singh, D. P. et al. (2019). Isolation and screening of high salinity tolerant Trichodermaspp. with plant growth property and antagonistic activity against various soilborne phytopathogens. Archives of Phytopathology and Plant Protection, 52(7–8), 667–680. [Google Scholar]
26. Foyer, C. H., Rasool, B., Davey, J. W., Hancock, R. D. (2016). Cross-tolerance to biotic and abiotic stresses in plants: A focus on resistance to aphid infestation. Journal of Experimental Botany, 67(7), 2025–2037. [Google Scholar] [PubMed]
27. Mona, S. A., Hashem, A., Abd_Allah, E. F., Alqarawi, A. A., Soliman, D. W. K. et al. (2017). Increased resistance of drought by Trichoderma harzianum fungal treatment correlates with increased secondary metabolites and proline content. Journal of Integrative Agriculture, 16(8), 1751–1757. [Google Scholar]
28. Hermosa, R., Viterbo, A., Chet, I., Monte, E. (2012). Plant-beneficial effects of Trichoderma and of its genes. Microbiology, 158(1), 17–25. [Google Scholar] [PubMed]
29. Cornejo-Ríos, K., Osorno-Suárez, M. D. P., Hernández-León, S., Reyes-Santamaría, M. I., Juárez-Díaz, J. A. et al. (2021). Impact of Trichoderma asperellum on chilling and drought stress in tomato (Solanum lycopersicum). Horticulturae, 7(10), 385. [Google Scholar]
30. Harman, G. E. (2006). Overview of mechanisms and uses of Trichoderma spp. Phytopathology, 96(2), 190–194. [Google Scholar] [PubMed]
31. Sharma, R., Joshi, A., Dhaker, R. (2012). A brief review on mechanism of Trichoderma fungus use as biological control agents. International Journal of Innovations in Bio-Sciences, 96(2), 190–194. [Google Scholar]
32. Kashyap, P. L., Solanki, M. K., Kushwaha, P., Kumar, S., Srivastava, A. K. (2020). Biocontrol potential of salt-tolerant Trichoderma and Hypocrea isolates for the management of tomato root rot under saline environment. Journal of Soil Science and Plant Nutrition, 20(1), 160–176. [Google Scholar]
33. Ramírez-Valdespino, C. A., Casas-Flores, S., Olmedo-Monfil, V. (2019). Trichoderma as a model to study effector-like molecules. Frontiers in Microbiology, 10, 1030. [Google Scholar]
34. Verma, H., Kumar, D., Kumar, V., Kumari, M., Singh, S. K. et al. (2021). The potential application of endophytes in management of stress from drought and salinity in crop plants. Microorganisms, 9(8), 1729. [Google Scholar] [PubMed]
35. Yan, C., Xie, D. (2015). Jasmonate in plant defence: Sentinel or double agent? Plant Biotechnology Journal, 13(9), 1233–1240. [Google Scholar] [PubMed]
36. Shoresh, M., Yedidia, I., Chet, I. (2005). Involvement of jasmonic acid/ethylene signaling pathway in the systemic resistance induced in cucumber by Trichoderma asperellum T203. Phytopathology, 95(1), 76–84. [Google Scholar] [PubMed]
37. Tornero, P., Gadea, J., Conejero, V., Vera, P. (1997). Two PR-1 genes from tomato are differentially regulated and reveal a novel mode of expression for a pathogenesis-related gene during the hypersensitive response and development. Molecular Plant-Microbe Interactions, 10(5), 624–634. [Google Scholar] [PubMed]
38. Nicolás, C., Hermosa, R., Rubio, B., Mukherjee, P. K., Monte, E. (2014). Trichoderma genes in plants for stress tolerance-status and prospects. Plant Science, 228, 71–78. [Google Scholar]
39. del Carmen Martinez-Ballesta, M., Carvajal, M. (2014). New challenges in plant aquaporin biotechnology. Plant Science, 217, 71–77. [Google Scholar]
40. Maurel, C., Boursiac, Y., Luu, D. T., Santoni, V., Shahzad, Z. et al. (2015). Aquaporins in plants. Physiological Reviews, 95(4), 1321–1358. [Google Scholar] [PubMed]
41. Liu, X. D., Wei, Y., Zhou, X. Y., Pei, X., Zhang, S. H. (2015). Aspergillus glaucus aquaglyceroporin gene glpF confers high osmosis tolerance in heterologous organisms. Applied and Environmental Microbiology, 81(19), 6926–6937. [Google Scholar] [PubMed]
42. Xu, H., Cooke, J. E., Zwiazek, J. J. (2013). Phylogenetic analysis of fungal aquaporins provides insight into their possible role in water transport of mycorrhizal associations. Botany, 91(8), 495–504. [Google Scholar]
43. Pacheco-Trejo, J., Aquino-Torres, E., Reyes-Santamaría, M. I., Islas-Pelcastre, M., Pérez-Ríos, S. R. (2022). Plant defensive responses triggered by Trichodermaspp. as tools to face stressful conditions. Horticulturae, 8(12), 1181. [Google Scholar]
44. Pehlivan, N., Saruhan-Güler, N., Alpay-Karaoğlu, Ş. (2018). The effect of Trichoderma seed priming to drought resistance in tomato (Solanum lycopersicum L.) plants. Hacettepe Journal of Biology and Chemistry, 46(2), 263–272. [Google Scholar]
45. Adnan, M., Islam, W., Shabbir, A., Khan, K. A., Ghramh, H. A. et al. (2019). Plant defense against fungal pathogens by antagonistic fungi with Trichoderma in focus. Microbial Pathogenesis, 129(8), 7–18. [Google Scholar] [PubMed]
46. Bashyal, B. M., Parmar, P., Zaidi, N. W., Aggarwal, R. (2021). Molecular programming of drought-challenged Trichoderma harzianum-bioprimed rice (Oryza sativa L.). Frontiers in Microbiology, 12, 655165. [Google Scholar] [PubMed]
47. Cai, F., Chen, W., Wei, Z., Pang, G., Li, R. et al. (2015). Colonization of Trichoderma harzianum strain SQR-T037 on tomato roots and its relationship to plant growth, nutrient availability and soil microflora. Plant and Soil, 388(1), 337–350. [Google Scholar]
48. Vieira, P. M., Coelho, A. S. G., Steindorff, A. S., de-Siqueira, S. J. L., Silva, R. D. N. et al. (2013). Identification of differentially expressed genes from Trichoderma harzianum during growth on cell wall of Fusarium solanias a tool for biotechnological application. BMC Genomics, 14(1), 1–11. [Google Scholar]
49. Zehra, A., Meena, M., Dubey, M. K., Aamir, M., Upadhyay, R. (2017). Activation of defense response in tomato against Fusarium wilt disease triggered by Trichoderma harzianum supplemented with exogenous chemical inducers (SA and MeJA). Brazilian Journal of Botany, 40(3), 651–664. [Google Scholar]
50. Gal-Hemed, I., Atanasova, L., Komon-Zelazowska, M., Druzhinina, I. S., Viterbo, A. et al. (2011). Marine isolates of Trichodermaspp. as potential halotolerant agents of biological control for arid-zone agriculture. Applied and Environmental Microbiology, 77(15), 5100–5109. [Google Scholar] [PubMed]
51. Sofo, A., Tataranni, G., Xiloyannis, C., Dichio, B., Scopa, A. (2012). Direct effects of Trichoderma harzianum strain T-22 on micropropagated shoots of GiSeLa6® (Prunus cerasus × Prunus canescens) rootstock. Environmental and Experimental Botany, 76, 33–38. [Google Scholar]
52. Sekmen-Cetinel, A. H., Gokce, A., Erdik, E., Cetinel, B., Cetinkaya, N. (2021). The effect of Trichoderma citrinoviride treatment under salinity combined to Rhizoctonia solani infection in strawberry (Fragaria × ananassa Duch.). Agronomy, 11(8), 1589. [Google Scholar]
53. Herrera-Téllez, V. I., Cruz-Olmedo, A. K., Plasencia, J., Gavilanes-Ruíz, M., Arce-Cervantes, O. (2019). The protective effect of Trichoderma asperellum on tomato plants against Fusarium oxysporum and Botrytis cinerea diseases involves inhibition of reactive oxygen species production. International Journal of Molecular Sciences, 20(8), 2007. [Google Scholar]
54. Guzmán-Guzmán, P., Kumar, A., de los Santos-Villalobos, S., Parra-Cota, F. I., Orozco-Mosqueda, M. D. C. et al. (2023). Trichoderma species: Our best fungal allies in the biocontrol of plant diseases—A review. Plants, 12(3), 432. [Google Scholar]
55. Sánchez-Cruz, R., Mehta, R., Atriztán-Hernández, K., Martínez-Villamil, O., del Rayo Sánchez-Carbente, M. et al. (2021). Effects on Capsicum annuum plants colonized with Trichoderma atroviride P. karst strains genetically modified in Taswo1, a gene coding for a protein with expansin-like activity. Plants, 10(9), 1919. [Google Scholar]
56. Speckbacher, V., Ruzsanyi, V., Martinez-Medina, A., Hinterdobler, W., Doppler, M. et al. (2020). The lipoxygenase Lox1 is involved in light- and injury-response, conidiation, and volatile organic compound biosynthesis in the mycoparasitic fungus Trichoderma atroviride. Frontiers in Microbiology, 11, 2004. [Google Scholar] [PubMed]
57. Rubio, M. B., Pardal, A. J., Cardoza, R. E., Gutiérrez, S., Monte, E. et al. (2017). Involvement of the transcriptional coactivator ThMBF1 in the biocontrol activity of Trichoderma harzianum. Frontiers in Microbiology, 8, 2273. [Google Scholar] [PubMed]
58. Kai, K., Mine, K., Akiyama, K., Ohki, S., Hayashi, H. (2018). Anti-plant viral activity of peptaibols, trichorzins HA II, HA V, and HA VI, isolated from Trichoderma harzianum HK-61. Journal of Pesticide Science, 43(4), 283–286. [Google Scholar] [PubMed]
59. Peng, K. C., Lin, C. C., Liao, C. F., Yu, H. C., Lo, C. T. et al. (2021). Expression of L-amino acid oxidase of Trichoderma harzianum in tobacco confers resistance to Sclerotinia sclerotiorum and Botrytis cinerea. Plant Science, 303, 110772. [Google Scholar] [PubMed]
60. Mironenka, J., Różalska, S., Bernat, P. (2021). Potential of Trichoderma harzianum and its metabolites to protect wheat seedlings against Fusarium culmorum and 2, 4-D. International Journal of Molecular Sciences, 22(23), 13058. [Google Scholar] [PubMed]
61. Poveda, J., Hermosa, R., Monte, E., Nicolás, C. (2019). The Trichoderma harzianum Kelch protein ThKEL1 plays a key role in root colonization and the induction of systemic defense in Brassicaceae plants. Frontiers in Plant Science, 10, 1478. [Google Scholar] [PubMed]
62. Mahmoud, G. A. E., Abdel-Sater, M. A., Al-Amery, E., Hussein, N. A. (2021). Controlling Alternaria cerealis MT808477 tomato phytopathogen by Trichoderma harzianum and tracking the plant physiological changes. Plants, 10(9), 1846. [Google Scholar] [PubMed]
63. Zhang, F., Chen, C., Zhang, F., Gao, L., Liu, J. et al. (2017). Trichoderma harzianum containing 1-aminocyclopropane-1-carboxylate deaminase and chitinase improved growth and diminished adverse effect caused by Fusarium oxysporum in soybean. Journal of Plant Physiology, 210, 84–94. [Google Scholar] [PubMed]
64. Gomes, E. V., Ulhoa, C. J., Cardoza, R. E., Silva, R. N., Gutiérrez, S. (2017). Involvement of Trichoderma harzianum Epl-1 protein in the regulation of Botrytis virulence-and tomato defense-related genes. Frontiers in Plant Science, 8, 880. [Google Scholar] [PubMed]
65. Chen, S. C., Ren, J. J., Zhao, H. J., Wang, X. L., Wang, T. H. et al. (2019). Trichoderma harzianum improves defense against Fusarium oxysporum by regulating ROS and RNS metabolism, redox balance, and energy flow in cucumber roots. Phytopathology, 109(6), 972–982. [Google Scholar] [PubMed]
66. Batool, R., Umer, M. J., Wang, Y., He, K., Zhang, T. et al. (2020). Synergistic effect of Beauveria bassiana and Trichoderma asperellum to induce maize (Zea mays L.) defense against the Asian corn borer, Ostrinia furnacalis (Lepidoptera, Crambidae) and larval immune response. International Journal of Molecular Sciences, 21(21), 8215. [Google Scholar] [PubMed]
67. Chou, H., Xiao, Y. T., Tsai, J. N., Li, T. T., Wu, H. Y. et al. (2019). In vitro and in planta evaluation of Trichoderma asperellum TA as a biocontrol agent against Phellinus noxius, the cause of brown root rot disease of trees. Plant Disease, 103(11), 2733–2741. [Google Scholar] [PubMed]
68. Yu, W., Mijiti, G., Huang, Y., Fan, H., Wang, Y. et al. (2018). Functional analysis of eliciting plant response protein Epl1-Tas from Trichoderma asperellum ACCC30536. Scientific Reports, 8(1), 1–13. [Google Scholar]
69. Zhang, H., Ji, S., Guo, R., Zhou, C., Wang, Y. et al. (2019). Hydrophobin HFBII-4 from Trichoderma asperellum induces antifungal resistance in poplar. Brazilian Journal of Microbiology, 50(3), 603–612. [Google Scholar] [PubMed]
70. Kumar, S., Shukla, V., Dubey, M. K., Upadhyay, R. S. (2021). Activation of defense response in common bean against stem rot disease triggered by Trichoderma erinaceum and Trichoderma viride. Journal of Basic Microbiology, 61(10), 910–922. [Google Scholar] [PubMed]
71. Singh, J., Singh, P., Vaishnav, A., Ray, S., Rajput, R. S. et al. (2021). Belowground fungal volatiles perception in okra (Abelmoschus esculentus) facilitates plant growth under biotic stress. Microbiological Research, 246(2), 126721. [Google Scholar] [PubMed]
72. Ramírez-Valdespino, C. A., Porras-Troncoso, M. D., Corrales-Escobosa, A. R., Wrobel, K., Martínez-Hernández, P. et al. (2018). Functional characterization of TvCyt2, a member of the p450 monooxygenases from Trichoderma virens relevant during the association with plants and mycoparasitism. Molecular Plant-Microbe Interactions, 31(3), 289–298. [Google Scholar]
73. Shoresh, M., Harman, G. E., Mastouri, F. (2010). Induced systemic resistance and plant responses to fungal biocontrol agents. Annual Review of Phytopathology, 48(1), 21–43. [Google Scholar] [PubMed]
74. Fu, J., Xiao, Y., Wang, Y. F., Liu, Z. H., Yang, K. J. (2019). Trichoderma affects the physiochemical characteristics and bacterial community composition of saline-alkaline maize rhizosphere soils in the cold-region of Heilongjiang Province. Plant and Soil, 436(1), 211–227. [Google Scholar]
75. Yin, D., Deng, X., Song, R. (2016). Synergistic effects between Suilllus luteus and Trichoderma virens on growth of Korean spruce seedlings and drought resistance of Scotch pine seedlings. Journal of Forestry Research, 27(1), 193–201. [Google Scholar]
76. Yang, R., Qin, Z., Wang, J., Zhang, X., Xu, S. et al. (2022). The interactions between arbuscular mycorrhizal fungi and Trichoderma longibrachiatum enhance maize growth and modulate root metabolome under increasing soil salinity. Microorganisms, 10(5), 1042. [Google Scholar] [PubMed]
77. Ważny, R., Rozpądek, P., Jędrzejczyk, R. J., Śliwa, M., Stojakowska, A. et al. (2018). Does co-inoculation of Lactuca serriola with endophytic and arbuscular mycorrhizal fungi improve plant growth in a polluted environment? Mycorrhiza, 28(3), 235–246. [Google Scholar] [PubMed]
78. Metwally, R., Al-Amri, S. (2020). Individual and interactive role of Trichoderma viride and arbuscular mycorrhizal fungi on growth and pigment content of onion plants. Letters in Applied Microbiology, 70(2), 79–86. [Google Scholar] [PubMed]
79. Gupta, A., Rico-Medina, A., Caño-Delgado, A. I. (2020). The physiology of plant responses to drought. Science, 368(6488), 266–269. [Google Scholar] [PubMed]
80. Muñoz-Espinoza, V. A., López-Climent, M. F., Casaretto, J. A., Gómez-Cadenas, A. (2015). Water stress responses of tomato mutants impaired in hormone biosynthesis reveal abscisic acid, jasmonic acid and salicylic acid interactions. Frontiers in Plant Science, 6(138), 997. [Google Scholar]
81. Lesk, C., Rowhani, P., Ramankutty, N. (2016). Influence of extreme weather disasters on global crop production. Nature, 529(7584), 84–87. [Google Scholar] [PubMed]
82. Bodner, G., Nakhforoosh, A., Kaul, H. P. (2015). Management of crop water under drought: A review. Agronomy for Sustainable Development, 35(2), 401–442. [Google Scholar]
83. Saba, H., Vibhash, D., Manisha, M., Prashant, K., Farhan, H. et al. (2012). Trichoderma a promising plant growth stimulator and biocontrol agent. Mycosphere, 3(4), 524–531. [Google Scholar]
84. Arora, N. K. (2019). Impact of climate change on agriculture production and its sustainable solutions. Springer, 2(2), 95–96. [Google Scholar]
85. Gornall, J., Betts, R., Burke, E., Clark, R., Camp, J. et al. (2010). Implications of climate change for agricultural productivity in the early twenty-first century. Philosophical Transactions of the Royal Society B: Biological Sciences, 365(1554), 2973–2989. [Google Scholar]
86. Kaur, S., Kumar, P. (2020). Ameliorative effect of trichoderma, rhizobium and mycorrhiza on internodal length, leaf area and total soluble protein in mung bean (Vigna radiata [L.] R. Wilazek) under drought stress. Journal of Pharmacognosy and Phytochemistry, 9(4), 971–977. [Google Scholar]
87. Jaleel, C. A., Manivannan, P., Wahid, A., Farooq, M., Al-Juburi, H. J. et al. (2009). Drought stress in plants: A review on morphological characteristics and pigments composition. International Journal of Agriculture and Biology, 11(1), 100–105. [Google Scholar]
88. Wahab, A., Abdi, G., Saleem, M. H., Ali, B., Ullah, S. et al. (2022). Plants’ physio-biochemical and phyto-hormonal responses to alleviate the adverse effects of drought stress: A comprehensive review. Plants, 11(13), 1620. [Google Scholar] [PubMed]
89. Medina, S., Vicente, R., Amador, A., Araus, J. L. (2016). Interactive effects of elevated [CO2] and water stress on physiological traits and gene expression during vegetative growth in four durum wheat genotypes. Frontiers in Plant Science, 7, 1738. [Google Scholar] [PubMed]
90. Contreras-Cornejo, H. A., Ortiz-Castro, R., López-Bucio, J., Mukherjee, P. (2013). Promotion of plant growth and the induction of systemic defence by Trichoderma: Physiology, genetics and gene expression. Trichoderma: Biology and Applications, 175, 96. [Google Scholar]
91. Tucci, M., Ruocco, M., De Masi, L., de Palma, M., Lorito, M. (2011). The beneficial effect of Trichodermaspp. on tomato is modulated by the plant genotype. Molecular Plant Pathology, 12(4), 341–354. [Google Scholar] [PubMed]
92. Mishra, D., Rajput, R. S., Zaidi, N. W., Singh, H. (2020). Sheath blight and drought stress management in rice (Oryza sativa) through Trichodermaspp. Indian Phytopathology, 73(1), 71–77. [Google Scholar]
93. Mastouri, F., Björkman, T., Harman, G. E. (2010). Seed treatment with Trichoderma harzianum alleviates biotic, abiotic, and physiological stresses in germinating seeds and seedlings. Phytopathology, 100(11), 1213–1221. [Google Scholar] [PubMed]
94. Rawal, R., Scheerens, J. C., Fenstemaker, S. M., Francis, D. M., Miller, S. A. et al. (2022). Novel Trichoderma isolates alleviate water deficit stress in susceptible tomato genotypes. Frontiers in Plant Science, 13, 869090. [Google Scholar] [PubMed]
95. Miura, K., Tada, Y. (2014). Regulation of water, salinity, and cold stress responses by salicylic acid. Frontiers in Plant Science, 5, 4. [Google Scholar] [PubMed]
96. Bae, H., Sicher, R. C., Kim, M. S., Kim, S. H., Strem, M. D. et al. (2009). The beneficial endophyte Trichoderma hamatum isolate DIS 219b promotes growth and delays the onset of the drought response in Theobroma cacao. Journal of Experimental Botany, 60(11), 3279–3295. [Google Scholar] [PubMed]
97. Bashir, M. A., Silvestri, C., Ahmad, T., Hafiz, I. A., Abbasi, N. A. et al. (2020). Osmotin: A cationic protein leads to improve biotic and abiotic stress tolerance in plants. Plants, 9(8), 992. [Google Scholar] [PubMed]
98. Doni, F., Isahak, A., Che-Mohd-Zain, C., Mohd-Ariffin, S., Wan-Mohamad, W. et al. (2014). Formulation of Trichoderma sp. SL2 inoculants using different carriers for soil treatment in rice seedling growth. SpringerPlus, 3(1), 532. [Google Scholar] [PubMed]
99. Contreras-Cornejo, H. A., Macías-Rodríguez, L., Cortés-Penagos, C., López-Bucio, J. (2009). Trichoderma virens, a plant beneficial fungus, enhances biomass production and promotes lateral root growth through an auxin-dependent mechanism in Arabidopsis. Plant Physiology, 149(3), 1579–1592. [Google Scholar] [PubMed]
100. Zargar, S. M., Gupta, N., Nazir, M., Mahajan, R., Malik, F. A. et al. (2017). Impact of drought on photosynthesis: Molecular perspective. Plant Gene, 11(1), 154–159. [Google Scholar]
101. Farooq, M., Wahid, A., Kobayashi, N., Fujita, D., Basra, S. (2009). Plant drought stress: Effects, mechanisms and management. In: Sustainable agriculture, pp. 153–188. Berlin: Springer. [Google Scholar]
102. Mastouri, F., Björkman, T., Harman, G. E. (2012). Trichoderma harzianum enhances antioxidant defense of tomato seedlings and resistance to water deficit. Molecular Plant-Microbe Interactions, 25(9), 1264–1271. [Google Scholar] [PubMed]
103. Ereful, N. C., Liu, L. Y., Greenland, A., Powell, W., Mackay, I. et al. (2020). RNA-seq reveals differentially expressed genes between two indica inbred rice genotypes associated with drought-yield QTLs. Agronomy, 10(5), 621. [Google Scholar]
104. Yogendra, S. G., Uma, S. S. (2014). Enhance activity of stress related enzymes in rice (Oryza sativa L.) induced by plant growth promoting fungi under drought stress. African Journal of Agricultural Research, 9(19), 1430–1434. [Google Scholar]
105. Guler, N. S., Pehlivan, N., Karaoglu, S. A., Guzel, S., Bozdeveci, A. (2016). Trichoderma atroviride ID20G inoculation ameliorates drought stress-induced damages by improving antioxidant defence in maize seedlings. Acta Physiologiae Plantarum, 38(6), 1–9. [Google Scholar]
106. Speirs, J., Binney, A., Collins, M., Edwards, E., Loveys, B. (2013). Expression of ABA synthesis and metabolism genes under different irrigation strategies and atmospheric VPDs is associated with stomatal conductance in grapevine (Vitis vinifera L. cv Cabernet Sauvignon). Journal of Experimental Botany, 64(7), 1907–1916. [Google Scholar] [PubMed]
107. Viterbo, A., Landau, U., Kim, S., Chernin, L., Chet, I. (2010). Characterization of ACC deaminase from the biocontrol and plant growth-promoting agent Trichoderma asperellum T203. FEMS Microbiology Letters, 305(1), 42–48. [Google Scholar] [PubMed]
108. De-Palma, M., Salzano, M., Villano, C., Aversano, R., Lorito, M. et al. (2019). Transcriptome reprogramming, epigenetic modifications and alternative splicing orchestrate the tomato root response to the beneficial fungus Trichoderma harzianum. Horticulture Research, 6(1), 5. [Google Scholar] [PubMed]
109. Fu, J., Xiao, Y., Wang, Y. F., Liu, Z. H., Zhang, Y. F. et al. (2021). Trichoderma asperellum alters fungal community composition in saline-alkaline soil maize rhizospheres. Soil Science Society of America Journal, 85(4), 1091–1104. [Google Scholar]
110. Elkelish, A. A., Alhaithloul, H. A. S., Qari, S. H., Soliman, M. H., Hasanuzzaman, M. (2020). Pretreatment with Trichoderma harzianum alleviates waterlogging-induced growth alterations in tomato seedlings by modulating physiological, biochemical, and molecular mechanisms. Environmental and Experimental Botany, 171, 103946. [Google Scholar]
111. AbdElgawad, H., Zinta, G., Hegab, M. M., Pandey, R., Asard, H. et al. (2016). High salinity induces different oxidative stress and antioxidant responses in maize seedlings organs. Frontiers in Plant Science, 7(580), 276. [Google Scholar] [PubMed]
112. Shahid, S. A., Zaman, M., Heng, L. (2018). Soil salinity: Historical perspectives and a world overview of the problem. In: Guideline for salinity assessment, mitigation and adaptation using nuclear and related techniques, pp. 43–53. Germany: Springer. [Google Scholar]
113. Otlewska, A., Migliore, M., Dybka-Stępień, K., Manfredini, A., Struszczyk-Świta, K. et al. (2020). When salt meddles between plant, soil, and microorganisms. Frontiers in Plant Science, 11, 1429. [Google Scholar]
114. Taufiq, A., Yusnawan, E. (2020). Influence of Trichoderma as a seed treatment on the growth and yield of groundnut under saline environment. Journal of Degraded and Mining Lands Management, 8(1), 2401–2409. [Google Scholar]
115. Marlet, S., Bouksila, F., Bahri, A. (2009). Water and salt balance at irrigation scheme scale: A comprehensive approach for salinity assessment in a Saharan oasis. Agricultural Water Management, 96(9), 1311–1322. [Google Scholar]
116. Rawat, L., Singh, Y., Shukla, N., Kumar, J. (2013). Salinity tolerant Trichoderma harzianum reinforces NaCl tolerance and reduces population dynamics of Fusarium oxysporum f. sp. ciceri in chickpea (Cicer arietinum L.) under salt stress conditions. Archives of Phytopathology and Plant Protection, 46(12), 1442–1467. [Google Scholar]
117. Mahajan, S., Tuteja, N. (2005). Cold, salinity and drought stresses: An overview. Archives of Biochemistry and Biophysics, 444(2), 139–158. [Google Scholar] [PubMed]
118. Yasmeen, R., Shaheed-Siddiqui, Z. (2017). Physiological responses of crop plants against Trichoderma harzianum in saline environment. Acta Botanica Croatica, 76(2), 154–162. [Google Scholar]
119. Prasad, S. R., Bagali, P. G., Hittalmani, S., Shashidhar, H. (2000). Molecular mapping of quantitative trait loci associated with seedling tolerance to salt stress in rice (Oryza sativa L.). Current Science, 78(2), 162–164. [Google Scholar]
120. Ali, B., Hafeez, A., Javed, M. A., Afridi, M. S., Abbasi, H. A. et al. (2022). Role of endophytic bacteria in salinity stress amelioration by physiological and molecular mechanisms of defense: A comprehensive review. South African Journal of Botany, 151(10), 33–46. [Google Scholar]
121. Jithesh, M., Prashanth, S., Sivaprakash, K., Parida, A. K. (2006). Antioxidative response mechanisms in halophytes: Their role in stress defence. Journal of Genetics, 85(3), 237–254. [Google Scholar] [PubMed]
122. Guan, B., Yu, J., Chen, X., Xie, W., Lu, Z. (2011). Effects of salt stress and nitrogen application on growth and ion accumulation of Suaeda salsa plants. 2011 International Conference on Remote Sensing, Environment and Transportation Engineering, pp. 8268–8272. Nanjing, China. [Google Scholar]
123. Li, J., Liu, L., Bai, Y., Zhang, P., Finkers, R. et al. (2011). Seedling salt tolerance in tomato. Euphytica, 178(3), 403–414. [Google Scholar]
124. Tejera, N. A., Campos, R., Sanjuan, J., Lluch, C. (2004). Nitrogenase and antioxidant enzyme activities in Phaseolus vulgaris nodules formed by Rhizobium tropici isogenic strains with varying tolerance to salt stress. Journal of Plant Physiology, 161(3), 329–338. [Google Scholar] [PubMed]
125. Jacoby, R., Che-Othman, M., Millar, A., Taylor, N. (2016). Analysis of the sodium chloride-dependent respiratory kinetics of wheat mitochondria reveals differential effects on phosphorylating and non-phosphorylating electron transport pathways. Plant, Cell & Environment, 39(4), 823–833. [Google Scholar]
126. Bai, Y., Kissoudis, C., Yan, Z., Visser, R. G., van-der-Linden, G. (2018). Plant behaviour under combined stress: Tomato responses to combined salinity and pathogen stress. The Plant Journal, 93(4), 781–793. [Google Scholar] [PubMed]
127. Foyer, C. H., Noctor, G. (2005). Redox homeostasis and antioxidant signaling: A metabolic interface between stress perception and physiological responses. The Plant Cell, 17(7), 1866–1875. [Google Scholar] [PubMed]
128. Khan, M. I. R., Khan, N. A. (2017). Reactive oxygen species and antioxidant systems in plants: Role and regulation under abiotic stress. Germany: Springer. [Google Scholar]
129. Fu, J., Liu, Z., Li, Z., Wang, Y., Yang, K. (2017). Alleviation of the effects of saline-alkaline stress on maize seedlings by regulation of active oxygen metabolism by Trichoderma asperellum. PLoS One, 12(6), e0179617. [Google Scholar] [PubMed]
130. Singh, P. K., Singh, R., Singh, S. (2013). Cinnamic acid induced changes in reactive oxygen species scavenging enzymes and protein profile in maize (Zea mays L.) plants grown under salt stress. Physiology and Molecular Biology of Plants, 19(1), 53–59. [Google Scholar] [PubMed]
131. Gill, S. S., Tuteja, N. (2010). Reactive oxygen species and antioxidant machinery in abiotic stress tolerance in crop plants. Plant Physiology and Biochemistry, 48(12), 909–930. [Google Scholar] [PubMed]
132. Ali, B., Hafeez, A., Ahmad, S., Javed, M. A., Afridi, M. S. et al. (2022). Bacillus thuringiensis PM25 ameliorates oxidative damage of salinity stress in maize via regulating growth, leaf pigments, antioxidant defense system, and stress responsive gene expression. Frontiers in Plant Science, 13, 9. [Google Scholar]
133. Yin, L., Wang, S., Li, J., Tanaka, K., Oka, M. (2013). Application of silicon improves salt tolerance through ameliorating osmotic and ionic stresses in the seedling of Sorghum bicolor. Acta Physiologiae Plantarum, 35(11), 3099–3107. [Google Scholar]
134. Baez-Rogelio, A., Morales-García, Y. E., Quintero-Hernández, V., Muñoz-Rojas, J. (2017). Next generation of microbial inoculants for agriculture and bioremediation. Microbial Biotechnology, 10(1), 19–21. [Google Scholar] [PubMed]
135. Anam, G. B., Reddy, M. S., Ahn, Y. H. (2019). Characterization of Trichoderma asperellum RM-28 for its sodic/saline-alkali tolerance and plant growth promoting activities to alleviate toxicity of red mud. Science of the Total Environment, 662, 462–469. [Google Scholar] [PubMed]
136. Gul Jan, F., Hamayun, M., Hussain, A., Jan, G., Iqbal, A. et al. (2019). An endophytic isolate of the fungus Yarrowia lipolytica produces metabolites that ameliorate the negative impact of salt stress on the physiology of maize. BMC Microbiology, 19(1), 1–10. [Google Scholar]
137. Sundareshwar, P., Morris, J., Koepfler, E., Fornwalt, B. (2003). Phosphorus limitation of coastal ecosystem processes. Science, 299(5606), 563–565. https://doi.org/10.1126/science.1079100 [Google Scholar] [PubMed] [CrossRef]
Cite This Article
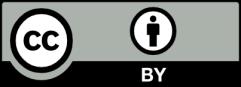
This work is licensed under a Creative Commons Attribution 4.0 International License , which permits unrestricted use, distribution, and reproduction in any medium, provided the original work is properly cited.