Open Access
ARTICLE
Genotypic Divergence, Photosynthetic Efficiency, Sodium Extrusion, and Osmoprotectant Regulation Conferred Salt Tolerance in Sorghum
1
Department of Crop Botany, Bangladesh Agricultural University, Mymensingh, 2202, Bangladesh
2
Planning and Development Division, Bangladesh Institute of Nuclear Agriculture, Mymensingh, 2202, Bangladesh
3
Department of Soil Science, Bangladesh Agricultural University, Mymensingh, 2202, Bangladesh
4
Agriculture and Food Technology Discipline, School of Agriculture, Geography, Environment, Ocean and Natural Sciences,
University of the South Pacific, Samoa
5
Agriculture Discipline, College of Science, Health, Engineering and Education, Murdoch University, Perth, WA 6150, Australia
6
Plant Production Department, College of Food and Agriculture, King Saud University, Riyadh, 11451, Saudi Arabia
7
Grassland and Forage Division, National Institute of Animal Science, Rural Development Administration, Cheonan, 31000, Korea
8
Department of Agronomy, Faculty of Agriculture, University of Poonch Rawalakot, Rawalakot, 12350, Pakistan
9
Department of Agronomy, Hajee Mohammad Danesh Science and Technology University, Dinajpur, Bangladesh
10 Faculty of Agriculture, Kafrelsheikh University, Kafrelsheikh, 33516, Egypt
* Corresponding Authors: Ayman El Sabagh. Email: ; A. K. M. Zakir Hossain. Email:
Phyton-International Journal of Experimental Botany 2023, 92(8), 2349-2368. https://doi.org/10.32604/phyton.2023.028974
Received 30 January 2023; Accepted 12 April 2023; Issue published 25 June 2023
Abstract
Salt stress is one of the major limitations to modern agriculture that negatively influences plant growth and productivity. Salt tolerant cultivar can provide excellent solution to enhance stress tolerance with plant fitness to unfavorable environments. Therefore, this study was aimed to screen salt tolerant sorghum genotypes through evaluating of different morphological, biochemical, and physiological attributes in response to salinity stress. In this study, we have been evaluated total six sorghum genotypes including Hybrid sorgo, Debgiri, BD-703, BD-706, BD-707, and BD-725 under salt stress (12 dS m−1 NaCl). The response variables included length and weight of root and shoot, root: shoot ratio (RSR), photosynthesis (A), transpiration rate (E), elemental concentrations (K+ , Na+ and K+ /Na+ ), photochemical efficiency of photosystem II (Fv/Fm), water use efficiency (WUE) and pigment content (chlorophyll a, and b). The results revealed that saline environment significantly reduced all response variables under study of sorghum genotypes, however, Hybrid sorgo remained unmatched by recording the maximum root and shoot traits. The same genotype recorded higher photosynthetic efficiency which was attributed to Na+ extrusion, K+ uptake and higher K+ /Na+ ratio (1.8 at stress), while these mechanisms were not fully active in rest of genotypes. Moreover, this study also implies the involvement of proline in imparting tolerance against saline environment in Hybrid sorgo genotype. Overall, BD-703 remained the most salt sensitive genotype as evident from the minimum morphological growth traits and the least biosynthesis of osmoprotectants. These findings open new research avenues for salt stress alleviation by identifying elite salt-tolerant genotypes of sorghum for breeding programs.Keywords
The addition of excess sodium salts (e.g., NaCl, NaSO4, NaHCO3, etc.) to agricultural soils is a major threat to agricultural production that inhibits plant growth and declines yield [1]. About 6% of the world’s soil and 20% of irrigated soil are salt-affected, which is expected to double by 2050 [2]. The agricultural soil is becoming more saline due to the addition of a disproportionate amount of several water-soluble salts. Salts of different compounds such as sodium, potassium, magnesium, calcium, sulfates, carbohydrates, bicarbonates, and chlorides are the primary cause of soil salinization [3–5]. Among them, the critical factor is several forms of sodium salts (NaCl, NaSO4, Na2CO3, and NaHCO3) primarily deposited to the soil by sodium-containing fertilizers and irrigation water [3–5]. The SS impairs a series of physiological processes and induces numerous molecular alterations in crop plants [6,7]. A few of the most pronounced salinity-induced alterations include inhibition of stomatal regulation, malfunctioning of photosynthetic machinery, and electron transport chain, which reduce the photosynthesis process and hamper plant growth [8,9]. More importantly, a higher accumulation of Na+ in the cytosol is responsible for creating toxicity in cells [3]. Additionally, the excessive accumulation of Na+ leads to disruption of metabolic processes such as osmotic imbalances and synthesis of reactive oxygen species (ROS) in crop plants. These metabolic disruptions lead to cellular injury, lipid peroxidation, oxidative stress, and even plant death [7,10].
To cope with SS induced disruptions, plants accumulate several osmoprotectants (proline, soluble sugar, and glycine betaine, etc.) [11–14], which act as defense molecules for enhancing plants’ acclimation through osmotic homeostasis. Moreover, plants have evolved antioxidant defense systems that protect from cellular injury and lipid peroxidation under saline conditions [10]. Furthermore, plants tend to throw out sodium ions (Na+) from cells to escape from salt toxicity which is known as the ions extrusion mechanism [7]. Plants which exhibit these potential physiological adaptations during salt stress are salt resistant, so it is necessary to consider these physiological adaptations during the screening of crop genotypes for getting higher salt resistance. Thus, to ensure food security for the ever-increasing population, it is necessary to select crop genotypes based on their adaptability potential for countering the deleterious effects of soil salinity.
Sorghum [Sorghum bicolor (L.) Moench] is a coarse grain crop of the Poaceae family that is being cultivated widely in warm, arid regions under both irrigated and rainfed conditions [15–22]. Although it can tolerate soil salinity up to 6.8 dSm−1 [23], higher salinity levels reduce its yield by 16%–63% [24]. Previously, it has been suggested to explore the genotypic variations in sorghum for screening out tolerant genotypes [25–28]. The research gaps exist pertaining to sorghum genotypes’ response in terms of morphological, physiological, and biochemical alterations under saline conditions. Thus, it was hypothesized that sorghum genotypes might respond differently to induced SS, and different morphological and physiological traits might assist in screening out the salt-tolerant genotypes. Moreover, it is expected that salt-tolerant sorghum genotypes may be grown in bare lands, which are fallow due to high soil salinity and may contribute to the global food security. Sorghum is the 5th most cultivated grain crop in the world. Drought tolerance efficiency is highly recognized compared to salt. However, sorghum cultivation is effective in saline-affected areas, though it has not yet been well established. Therefore, screening of salt-tolerant efficient genotypes will encourage us to enhance salt tolerance in sorghum through an appropriate breeding program. Therefore, this study was designed to screen out salt-tolerant sorghum genotypes from the pool of promising high-yielding cultivars based on different morphological, biochemical, and physiological attributes in response to salinity stress. We found that the Hybrid sorgo genotype showed better salt adaptation against salt stress. This study would be helpful to the sorghum breeders and farmers for improving agricultural traits and enhancing salt stress tolerance in sorghum.
2.1 Plant Materials and Growth Conditions
The trial was conducted at the Department of Crop Botany, Bangladesh Agricultural University, Bangladesh. The planting material included viable seeds of sorghum genotypes (Hybrid sorgo, Debgiri, BD 703, BD 706, BD 707, and BD 725). Among the six genotypes, Debgiri and Hybrid sorgo were collected from India and Japan, respectively, while the rest were collected from the seed bank of Bangladesh Agricultural Research Institute (BARI). The experiment was conducted in a completely randomized design (CRD) with three replications. Sorghum seeds were surface-sterilized with a 5% NaOCl (sodium hypochlorite) solution and then rinsed thrice with distilled water. The seeds were placed into a net for germination for four days. Thereafter, sorghum seedlings were transferred to the plastic box (area 70 cm3 × 24 cm3 × 23 cm3 having the size of 32 L) containing a hydroponic system containing a perforated styrofoam tray (9 × 3 = 27 holes) (Fig. 1). Pre-germinated 5-day-old seedlings were planted into the holes of the tray and supplied with the half-strength Hoagland’s nutrient solutions [29] containing 1 mM K2SO4, 0.2 mM KH2PO4, 2 mM Ca(NO3)2.4H2O, 0.5 mM MgSO4.7H2O, 2 mM CaCl2.2H2O, 1 µM H3BO3, 200 µM Fe-EDTA, 2 µM MnSO4.6H2O, 0.3 µM CuSO4.5H2O, 0.5 µM ZnSO4.7H2O and 0.01 µM (NH4)6Mo7O24. The pH of the hydroponic solution was maintained at 6.0. The sorghum seedlings were cultivated in a control growth chamber having a constant temperature of 25°C, white fluorescent light (300–350 μmol m−2 s−1) for 16 and 8-h dark cycles, and 60%–65% relative humidity. The 4-week-old seedlings were exposed to NaCl stress (Electrical Conductivity value of 12 dS m−1), while the control treatment was also maintained in a standard nutrient solution (control). The salt treatment was terminated after a week. In order to remove salt ions from the root surface, the plant roots were washed properly with distilled water. The samples of control and salt-treated sorghum plants were harvested separately and kept at −80°C until further analyses.
Figure 1: Laboratory arrangements of (a) sorghum seedlings, (b) set up of the hydroponic system, (c) growth condition of the sorghum seedlings inside the growth chamber, and (d) comparison of plants growing in controlled and stressed conditions
The length (mm) of roots (RL) and shoots (SL) were recorded using a ruler scale, while fresh weights (g) of roots (RFW) and shoots (SFW) were taken immediately using a precision digital balance. The plant samples were dried in electronic even at 70°C for 72 h, and the root dry weight (RDW) and shoot dry weight (SDW) was also recorded. The total fresh weights (TFW) were obtained by aggregating RFW and SFW. The root-shoot dry weight ratio (RSR) was calculated using the following formula:
RSR=RDWSDW (1)
2.3 Photosynthesis Indices and Water Use Efficiency
The photosynthesis indices were recorded using a photosynthetic machine (LCi–SD photosynthetic system, ADC BioScientific, Ltd., Hertfordshire, UK). The gaseous exchange traits, including A (rate of photosynthesis), gs (stomatal conductance), and E (rate of transpiration) were measured at 350 µmol m−2 s−1 PPFD in ambient temperature. For A determination, the leaves’ internal CO2 level was maintained at 400 ppm. The topmost leaves were chosen for the assessment in all growth conditions. The instantaneous water use efficiency (WUEins) was obtained by following the protocol of Huang et al. [30]. The maximal photochemical efficiency of photosystem II (Fv/Fm) was recorded in every genotype. The Fv/Fm was recorded in sorghum leaves using a fluorometer (Pocket PEA, Hansatech, Norfolk, UK), and the data were recorded from control and salt-treated leaves. The plants were adapted at dark for 30 min prior to data recording. The Pocket PEA was used to quantify the Fv/Fm at saturated 3500 µmol m−2 s−1 PPFD [31].
The measurement of leaf chlorophyll content (Chl a, b) from control and salt-treated plants was performed. Fifty milligrams of fresh leaves tissue were homogenized with 10 mL of 80% acetone assay [32], which was incubated at 65°C for 30 min in the dark. The absorbance of the solution was measured using UV-vis spectrophotometry (DR6000, Hach, Germany). The readings of the solution were taken at 647 and 663 nm, respectively. Total Chl (a+b) was calculated as the sum of Chl a and b, while the ratio of chlorophyll a and b (Chl a/b) was also estimated.
The ions concentration was determined according to standard protocols [33]. Sorghum seedlings’ roots and shoots were separated and washed thrice with Milli-Q water to remove salt from the surface. Plant samples were dried in an oven for 72 h at 70°C and subjected to digestion using a solution (HClO4/HNO3, 1:3 v/v) [34]. The elemental concentrations (K+, Na+) were measured by atomic absorption spectrophotometer (Perkin-Elmer 2380, Perkin Elmer, Rutherfordton, USA).
The proline accumulation was estimated according to the standard methods [3,35] by taking 50 mg plant tissues which were homogenized using 10 mL of 3% sulfosalicylic acid and centrifuged at 10,000 rpm for 10 min. The supernatant, acid ninhydrin, and glacial acid were mixed 1:1:1 in a new tube and incubated at 95°C water bath for an hour, and subsequently transferred in the tubes in an ice bath for 5 min. Toluene was supplemented to the mixture and shaken well. Two phases (aqueous and oil) were developed in the mixture, and the solution from the upper phase was measured using UV-vis spectrophotometric (DR6000, Hach, Germany); absorbance was taken at 520 nm. The proline content was calculated using L-proline by following the standard method.
2.6 Screening of Salt-Tolerant Genotypes
The salt-tolerant genotype was screened using the STIs of each trait were calculated according to the method [36] using the following formula:
STI=Dc×Ds(Xc)2 (2)
where, Dc = data of a genotype belonging to the control environment; Ds = data of the same genotype belonging to the stress environment, and Xc = average data of genotypes belonging to the control environment.
2.7 Heatmap and Principal Component Analysis (PCA)
To determine the response patterns of different physiological traits of six sorghum genotypes, the standardized STI values were used to construct a two-way hierarchical clustering heat map using the open-source statistical package Complex Heatmap in the R program (R version 4.1.3). The principal component analysis (PCA), biplot, and correlation–matrix scatter plot were generated using the R program.
The MINITAB software (Minitab version 20) was used to compare the treatment means, while data were subjected to two–way (genotype and salt stress) and one–way (genotype with STI values) analysis of variance (ANOVA) with a significant level of p < 0.05. All the results were presented as mean value ± standard error of three replications.
3.1 Morpho-Physiological Traits
Salt stress-induced phenotypic variations in the root (RL) and shoot (SL) length, and their fresh (RFW and SFW) and dry (RDW and SDW) weights among the six sorghum genotypes have been demonstrated in Fig. 2. All genotypes regulated the morpho-physiological traits in response to salinity compared to control plants (Table 1). The genotype (G) and stress (S) interactions were highly significant for most of the morpho-physiological traits except the Fv/Fm and stomatal conductance (Table 1). However, the Hybrid sorgo genotype remained unmatched in mitigating salt stress compared to other sorghum genotypes. After salt treatment, RL and SL showed significant variation between the control (414.1 and 602.0 mm, respectively) and salt-stressed (346.3 and 558.3 mm, respectively) genotypes (Fig. 2). The RL of 337.1 and 455.7 mm for BD-703 and Debgiri, respectively, under control conditions, while the corresponding figures for BD-703 and Hybrid sorgo were 271 and 411 mm, respectively (Table 2). Similarly, SL ranged from 559.9–658 mm under the control condition and 522.2–610 mm under the stress condition (Table 2). Moreover, the lowest reduction of RL and SL due to salt stress was found in Hybrid Sorgo, and they are 9.8% and 2.94%, respectively.
Figure 2: Boxplots demonstrating the descriptive statistics of morpho-physiological and biochemical traits of 6 sorghum genotypes grown in control and salt conditions under a hydroponic system. The treatment means are denoted with blue dots, while the medians are characterized by the white and thick horizontal box dividing lines. The higher and lower whiskers and the box boundaries of ups and down denote the Q3 (75th percentile), Q1 (25th percentile), maximum (Q1+1.5 IQR), and minimum (Q1–1.5 IQR) values, respectively. IQR denotes Interquartile Range. *, *** and ns denote significant variation between treatments at 5%, 0.1% levels of probability, and non-significant, respectively
The salt stress negatively affected SFW and RFW (Table 1) as salt-stressed plants produced a significantly lower SFW (4.95 g) and RFW (2.8 g) compared to control plants (10.2 and 4.03 g, respectively) (Fig. 2). The maximum SFW was observed in Hybrid sorgo (6.27 g) and minimum in BD-706 (3.39 g), while RFW was maximum in Hybrid sorgo (3.84 g) and minimum in BD-707 (1.86 g) under SS condition (Table 2). Again, the mean values of SDW and RDW under stress conditions (0.74 and 0.17 g, respectively) were significantly lower than the control plants (1.25 and 0.31 g, respectively) (Fig. 2). The SDW and RDW ranged from 0.55–0.95 g and 0.09–0.28 g under salt-stressed conditions (Table 2). The TFW, TDW, and RSR were significantly affected by salinity compared to control plants (Table 1). The average TFW, TDW, and RSR of each genotype were substantially higher in control plants (14.2, 1.57, and 0.25 g, respectively) in comparison to the stressed plants (7.75, 0.91, and 0.22 g, respectively) (Fig. 2). The TFW, TDW, and RSR showed significant variations, while the TFW and TDW were maximum in Hybrid sorgo (10.1 and 0.28 g, respectively) and minimum in BD-707 (5.54 and 0.09 g, respectively) under SS (Table 2). The RSR showed a similar fashion as RSR ranged from 0.19–0.34 under the control condition, while their values were 0.17–0.30 under the SS condition (Table 2).
3.2 Photosynthesis, Stomatal Conductance, and Transpiration
Salt stress considerably declined the rate of photosynthesis (A) and rate of transpiration (E) in all sorghum genotypes (Fig. 3). However, the decrement of stomatal conductance (gs) was not statistically significant in all the genotypes. The genotype Hybrid sorgo remained superior in terms of photosynthesis parameters under study. The reduction rate of photosynthesis due to salt stress was minimum in Hybrid sorgo, which is 39.8%. The performance of gs in Hybrid sorgo was also better compared to the rest of the genotypes (Fig. 3b). However, the E rate was significantly higher in Debgiri under salt stress, while BD-703 showed the lowest E rate under SS (Fig. 3b).
Figure 3: The (a) rate of photosynthesis (A; µmol CO2 m−2 s−1), (b) stomatal conductance (gs; mol m−2 s−1), and (c) rate of transpiration (E; mmol m−2 s−1) of six sorghum genotypes growing in control and salt-stressed conditions. Vertical bars on top of each bar are SEM (n = 3). Different letters on treatment means represent the significant difference at 5% levels of significance. The parameters which lack letters represent non-significant
3.3 Quantum Yield of PSII (Fv /Fm) and Water Use Efficiency (WUE)
The rate of Fv/Fm and WUE were differentially regulated under salt stress in sorghum genotypes. The Fv/Fm level was significantly regulated in Debgiri, BD-703, and BD-725, while no considerable variation was observed in the rest of the genotypes (Fig. 4a). The WUE was higher in Hybrid sorgo and BD-703 than in the rest of the sorghum genotypes (Fig. 4b). Under salt stress, the WUE was increased in Hybrid sorgo than in control, and Hybrid sorgo showed 44.4% higher WUE than the control condition.
Figure 4: (a) Maximum photochemical efficiency of PS II (Fv/Fm) and (b) Instantaneous Water use efficiency (WUEins; µmol mmol−1) of six sorghum genotypes exposed to control and salt stress conditions. Vertical bars on top of each bar are SEM (n = 3). Different letters on treatment means represent the significant difference at 5% levels of significance. The parameters which lack letters represent non-significant
3.4 Alteration of Leaf Pigments During Salt Stress
The leaf pigments content was significantly reduced in all genotypes due to salinity as leaf Chl a, Chl b, Total Chl as well as Chl a/b ratio were significantly higher in non-stressed plants compared to salt-stressed plants (Fig. 5). Significant genotypic variations were observed in Chl a, Chl b, total Chl, and Chl a/b among the genotypes because Chl a level severely declined in BD-703, while Hybrid sorgo exhibited non-significant variation of Chl a level in the saline environment (Fig. 5a). The BD-706 genotype showed a significant reduction of Chl b than the other genotypes, while Hybrid sorgo showed no significant variation under control and SS conditions (Fig. 5b). The same pattern was observed for total Chl, which was the sum of Chl a and Chl b (Fig. 5c). The Chl a and Chl b ratios were differently regulated in sorghum genotypes. The lowest Chl ratio was found in BD-703 under SS, and it was oppositely altered between BD-706 and BD-725 (Fig. 5d). However, no significant difference was observed in Hybrid sorgo under SS and normal conditions.
Figure 5: (a) The chlorophyll a (Chl a; mg g−1 FW), (b) chlorophyll b (Chl b; mg g−1 FW), (c) total chlorophyll (TotalChl; mg g−1 FW), and (d) ratio of Chl a over Chl b (Chl a/b) of six sorghum genotypes grown in control and salt stress conditions. Vertical bars on top of each bar are SEM (n = 3). Different letters on treatment means represent the significant difference at 5% levels of significance
3.5 Regulation of Ion Concentrations
The sodium (Na+) and potassium (K+) accumulations were differently altered during salt stress in sorghum genotypes (Fig. 3). The highest Na+ concentration was found in BD-706 among the six sorghum cultivars (Fig. 3a). However, the Na+ level was significantly lower in Hybrid sorgo compared to BD-706. The K+ accumulation significantly increased in Hybrid sorgo under salt stress (Fig. 6b). The K+/Na+ ratio was higher in control plants than salt-stress plants (Fig. 6c). The K+/Na+ was highest, and almost similar accumulation patterns were observed in Hybrid sorgo and BD-703 under salt stress.
Figure 6: (a) The Na+ concentrations (%), (b) K+ concentrations (%), and (c) ratio of K+ over Na+ (K+/Na+) of six sorghum genotypes grown in two stress conditions (control and salt). Vertical bars on top of each bar are SEM (n = 3). Different letters on treatment means represent the significant difference at 5% levels of significance
3.6 Accumulation of Proline under Salt Stress
The proline concentration was significantly enhanced under salt stress compared to normal conditions (Fig. 7). The leaf proline accumulation was significantly higher in Hybrid Sorgo under SS (Fig. 7a). The root proline was considerably accumulated in Hybrid Sorgo, while the genotypes Debgiri, BD-707, and BD-725 showed a similar accumulation pattern in roots under SS (Fig. 7b). The highest total proline accumulation was found in the Hybrid Sorgo genotype (Fig. 7c).
Figure 7: The (a) leaf proline (LeafPro; mg/100g FW), (b) root proline (RootPro; mg/100g FW), and (c) total proline (TotalPro; mg/100g FW) concentrations in sorghum genotypes grown in control and stress environments. Vertical bars on top of each bar are SEM (n = 3). Different letters on treatment means represent the significant difference at 5% levels of significance
3.7 Variations in Stress Tolerance Indices (STI)
The analyses of the hierarchical clustering heatmap of genotypes and several physiological traits were presented in Fig. 8. A total of 24 different traits were presented in the heatmap, in which the highest number of traits (total 15) showed up-regulation patterns in Hybrid Sorgo (Fig. 8a). The genotypes and observed parameters were clustered using STI, and the number of clusters was fixed by the gap statistic method before clustering. The hierarchical clustering heatmap of genotypes and traits, along with the dendrogram, is depicted in Fig. 8a. Six (6) sorghum genotypes were clustered into three row-clusters according to the existing variations in the different traits (Hybrid Sorgo in Cluster-2, BD-703 and BD-707 in Cluster-3, and the other three were in Cluster-1). Similarly, the parameters were clustered into three column-clusters where Cluster-1, Cluster-2, and Cluster-3 comprised 12, 4, and 8 traits, respectively (Fig. 8a). The highly linked parameters, for instance, Chl b, Chl a, TotalChl, RDW, RFW, SFW, SDW, RSR, TFW, Fv/Fm, A, and WUEins were assembled in Cluster-1; K+ (TotalK), K+/Na+ (KNa), TDW and RootPro under Cluster-2 and E, Chl a/b (Chlab), RL, LeafPro, TotalPro, gs, SL and Na+ (TotalNa) were compiled in Cluster-3 (Fig. 8a).
Figure 8: (a) Hierarchical clustering and heat map represent the STI of 6 sorghum genotypes and 24 different traits under salt stress conditions. STIs were standardized to a relative scale of −2 to +2 for the heat map. Different traits are represented by different columns, whereas genotypes are represented by different rows of the heatmap. Both the genotypes and traits were clustered into three groups each. The intensity of genotype-traits’ association is illustrated using various colors and their intensities. Salt sensitivity or the lower STIs are illustrated as dark red color, while salt tolerance or higher STIs are presented as dark blue color. (b) Radar plot showing STI values of different traits in three clusters of 6 sorghum genotypes. The control values are standardized to 1, and the STI values are expressed as times of the control
According to the STI values, the genotype Hybrid sorgo in Cluster-2 showed a greater extent of salt tolerance, followed by Cluster-3 and Cluster-1 (Fig. 8b). Out of 24 traits, the lowest STI values for Na+ were considered as salt-tolerant, while the greater STI values in the other 24 parameters, including proline, were depicted as tolerance attributes. The STI values of TDW and root-Pro were higher in Cluster-1 and subsequently in Cluster-2 and Cluster-3 (Fig. 8b). Additionally, the maximum STI of K+ and K+/Na+ were observed in Cluster-2, followed by Cluster-1 and Cluster-3. The STI values of the leaf and total proline in the clusters were ranked as Cluster-2 > Cluster-3 > Cluster-1 (Fig. 8b). However, the heat map illustrated that the genotype under Cluster-2, such as Hybrid sorgo, exhibited higher salt tolerance compared to the genotypes belonging to Cluster-1 and Cluster-3 (Fig. 8b).
3.8 Principal Component Analysis
The PCA results exhibited that the leading two principal components (PCs) are responsible for 68.9% of the overall dissimilarity (Fig. 9). The PC1 and PC2 were considered to construct PCA-biplot. In PCA-biplots, PC1 exhibited 51.7% of the total variation, while PC2 exhibited 17.2% variability among the traits. The first biplot showed the variations and correlation between the treatments. Traits include Total Na+, root Pro, leaf Pro, and total Pro were nearly located in the same, which indicated a strong positive correlation and contribution to SS (Fig. 9a). The genotypic variance and/or correlations with the total 24 traits were also clarified. The genotypes Hybrid sorgo and BD-725 clustered in the same plot, while Debgiri, BD-725, and BD-707 were clustered in another plot (Fig. 9b). These results indicate a strong positive correlation between the Hybrid sorgo and BD-725 in terms of salt tolerance.
Figure 9: (a) Principal component analysis (PCA)-biplot of 6 sorghum genotypes based on the variance in 24 traits grown under stressed and non-stressed conditions. (b) Distribution of genotypic variance in different ordinates according to PC1 and PC2. (c) Proportion of variance (%) and (d) Eigenvalues of first 10 components derived from PCA. Contribution (%) of the top 15 observed traits to (e) PC1 and (f) PC2. In PCA, PC1, as well as PC2 described 51.7% and 17.2% of the total dissimilarity, respectively. Arrows indicate the strength of the trait influence on the first two PCs. The contribution of the traits to the first two components in PCA was denoted by the different color intensities and lengths of the arrows. The darker red and long arrows indicate a higher contribution, while the darker green and shorter arrows indicate a lower contribution of the variables
3.9 Correlation among the Traits
Most of the morpho-physiological and biochemical traits were correlated with each other (Fig. 10). However, the correlation levels were different among the traits. According to the results of Pearson’s correlation analyses, RL, TotalK, E, gs, A, KNa, Fv/Fm, RFW, SFW, TFW, SDW, TDW, SL, RD, and WUE showed strong positive correlation, while LeafPro, TotalPro, TotalNa, and RootPro showed moderate to strong correlations (Fig. 10).
Figure 10: Correlation matrix of the measured 24 traits in six sorghum genotypes grown in control and salt stress conditions based on Pearson’s correlation analysis. Red and Blue shades specify negative and positive correlations, respectively. The higher the color intensity, the higher the correlation coefficient. Asterisks denote a significant correlation between traits, and *, **, and *** specify 5%, 1%, and 0.1% levels of significance, respectively. Insignificant (p ≥ 0.05) correlations are specified by blank blocks
The study was conducted to screen out the salt-tolerant genotypes of sorghum based on morphological, physiological, and biochemical traits, while the results revealed a combination of different mechanisms associated with salt-stress tolerance in sorghum. These research findings highlighted several morphological, physiological, and biochemical traits which were linked to salt tolerance.
4.1 Genotypic Variations, Robust Growth, and Photosynthetic Efficiency Confer Salt Tolerance
Our study exhibited Hybrid sorgo as the superior genotype that potentially maintained growth-related traits in a saline environment. This might be ascribed to the regulation of Chl in salt-stressed plants. A noticeable reduction of plant biomass was observed for the rest of the sorghum genotypes, confirming morphological inhibition as one of the adverse effects of SS. These findings are in concurrence with previously reported results where inhibition of growth attributes was closely linked to the sensitivity of crop plants to salt toxicity [3]. These findings also state that the salinity of NaCl salt gives rise to shortened growth attributes in stress conditions than control. These salinity-induced shortened growths might have happened to the declined cell elongation rate and a smaller number of elongated cells [37–39]. Cell elongation in salinity might be hampered due to a decline in the transportation of crucial ions like NO3− as well as a higher rate of absorption of Na+ ions in plants under elevated salt stress [29]. Furthermore, declined turgor pressure, in addition to the restricted availability of water to the cell, might be another reason for the declined fresh weight of the plant [40–42]. However, growth reduction was also reported to be the direct result of physiological damage under salt toxicity [43]. Likewise, our results regarding better morphological growth traits of salt-tolerant genotype (Hybrid sorgo) could also be explained by the regulation of the photosynthesis process under SS [7]. High photosynthesis and transpiration maintaining the ability of plants under SS has already been recognized as one of the most pronounced strategies of crop plants in order to offset the deleterious effects of the saline environment [44].
Although optimum accumulation of Na+ results in the balancing of osmoregulation, however over-accumulation in the cytosol can be toxic to plants by inhibiting K+ transport and disturbing K+ in cells [45]. Salt-tolerant plants inhibit Na+ or push it out from cells to mitigate salt toxicity [3]. Our study indicated that Hybrid sorgo seedlings accumulated low Na+ and high K+, suggesting it is one of the key mechanisms of Hybrid sorgo for maintaining lower Na+ that led to better adaptation under SS. These results are supported by previously reported conclusions whereby the extrusion of salt ions was declared responsible for imparting salinity tolerance in crop plants [46]. In addition, salt tolerance often depends on the ability to maintain K+/Na+ ratio through declining Na+ and enhancing Na+ and in halophytes [47]. In this study, the ability of Na+ and K+ homeostasis in Hybrid sorgo enhanced adaptation to SS, while the rest of the sorghum genotypes showed severe morphological and photosynthetic disruptions. A recent study suggested that high Na+ accumulation led to disruption of metabolic processes and reduced plant growth and productivity [3]. However, our findings have explicitly indicated that the mechanism of Na+ ion exclusion was fully active in Hybrid sorgo, while the K+ depletion (losses of K+ from the tissue due to a high level of Na+) hypothesis was applicable for the rest of the five sorghum genotypes.
4.3 Regulation of Osmoprotectants
In the saline environment, plants tend to accumulate several compatible solutes (proline, soluble sugar, glycine betaine, etc.), which protect cells from the adverse effects of osmotic stress [3,48]. Proline (Pro) serves as a beneficial safeguard for plants exposed to salinity stress by preventing cellular damages [49]. Besides contributing as an excellent osmolyte, Pro acts as an antioxidative defense molecule, metal chelator, and signaling molecule in salt-affected plants [6,50]. In this study, Pro accumulation was considerably higher in Hybrid sorgo than in the rest of the genotypes under SS. However, this observation suggests that a high concentration of Pro provided more support to Hybrid sorgo for coping with salt-induced osmotic stress, while the rest of sorghum genotypes were severely affected by salt-induced toxicity. These results indicate that 12 dS m−1 was above the salinity tolerance potential of the remaining five genotypes of sorghum which must have suffered oxidative damages.
Principal component analysis (PCA)-biplot is a multivariate analysis that facilitates the main characters for the selection of salt-tolerant genotypes by combining two dimensions (traits and objects) and minimizing variations [51]. The PCA revealed that the traits include TDW, gs, SFW, SDW, TFW, RDW, E, A, SL, total Na, RL, KNa, RFW, TotalPro, LeafPro, Chl a, total Chl, and root Pro were correlated differentially among the genotypes. In this study, the correlation pattern disclosed a considerable and strong positive relationship between K+/Na+ and almost all of the traits except for Na+ and proline concentrations (Fig. 10). Similarly, A, E, and gs maintained a negative correlation with total Na+. Interestingly, similar trends were recorded for the total proline concentrations. Therefore, considering PCA and correlation analyses, the proline accumulation, ion concentration, and photosynthetic regulation-related traits might be effective as evidence for screening salt tolerant genotypes as suggested by a number of previous researches [4,13,52,53]. Moreover, underlying mechanisms that enable crop plants to cope with the adverse effects of salinity by maintaining photosynthetic efficiency, salt extrusion, and biosynthesis of osmoprotectants need further research [26,54–57].
This work unveils the mechanistic basis of salt tolerance in sorghum. Differential regulations of morphological and physiological traits among the six sorghum genotypes indicated their sensitivity in response to salt stress. It was inferred that the Hybrid sorgo genotype surpassed the rest of the genotypes in terms of salt tolerance by maintaining morphological growth, physiological functioning, and photosynthetic activity in a saline environment. Additionally, significantly higher salt extrusion and K+ uptake by Hybrid sorgo were attributed to higher salt tolerance. This study also implies the involvement of osmoprotectants (e.g., proline) in imparting tolerance against excessive salts. The traits associated with genotypic variation in salt stress tolerance provide meaningful information to breeders and farmers to improve sorghum production on saline soils. These findings open up new avenues for research into salt stress alleviation and help to screen elite genotypes for sorghum breeding programs. However, these combined insights may encourage us to set more suitable approaches for alleviating salt stress in sorghum and other plant species.
Acknowledgement: The authors extend their appreciation to Researchers Supporting Project number (RSP2023R390), King Saud University, Riyadh, Saudi Arabia. We would like to acknowledge Md. Eakramul Hoque, Md. Rishad Abdullah, Maruf Abid, and Marufa Sultana, for the technical assistance. We would also like to acknowledge Md. Ashrafuzzaman, Md. Masudul Karim and Md. Abdur Razzak, for the helpful discussion.
Funding Statement: This research was funded by the Researchers Supporting Project No. (RSP2023R390), King Saud University, Riyadh, Saudi Arabia. The first author was awarded partial funding to conduct this study from the Ministry of Science and Technology, Bangladesh, through the National Science and Technology Fellowship.
Conflicts of Interest: The authors declare that they have no conflicts of interest to report regarding the present study.
References
1. Isayenkov, S. V., Maathuis, F. J. M. (2019). Plant salinity stress: Many unanswered questions remain. Frontiers in Plant Science, 10, 80. https://doi.org/10.3389/fpls.2019.00080 [Google Scholar] [PubMed] [CrossRef]
2. Butcher, K., Wick, A. F., DeSutter, T., Chatterjee, A., Harmon, J. (2016). Soil salinity: A threat to global food security. Agronomy Journal, 108(6), 2189–2200. https://doi.org/10.2134/agronj2016.06.0368 [Google Scholar] [CrossRef]
3. Rahman, M. A., Woo, J. H., Lee, S. H., Park, H. S., Kabir, A. H. et al. (2022). Regulation of Na+/H+ exchangers, Na+/K+ transporters, and lignin biosynthesis genes, along with lignin accumulation, sodium extrusion, and antioxidant defense, confers salt tolerance in alfalfa. Frontiers in Plant Science, 13, 1041764. https://doi.org/10.3389/fpls.2022.1041764 [Google Scholar] [PubMed] [CrossRef]
4. Mbarki, S., Talbi, O., Skalicky, M., Vachova, P., Hejnak, V. et al. (2022). Comparison of grain sorghum and alfalfa for providing heavy metal remediation of sandy soil with different soil amendments and salt stress. Frontiers in Environmental Science, 10, 1022629. https://doi.org/10.3389/fenvs.2022.1022629 [Google Scholar] [CrossRef]
5. Rahman, M. A., Lee, S. H., Ji, H. C., Kabir, A. H., Jones, C. S. et al. (2018). Importance of mineral nutrition for mitigating aluminum toxicity in plants on acidic soils: Current status and opportunities. International Journal of Molecular Sciences, 19(10), 3073. https://doi.org/10.3390/ijms19103073 [Google Scholar] [PubMed] [CrossRef]
6. Khan, I., Muhammad, A., Chattha, M. U., Skalicky, M., Chattha, M. B. et al. (2022). Mitigation of salinity-induced oxidative damage, growth, and yield reduction in fine rice by sugarcane press mud application. Frontiers in Plant Science, 13, 840900. https://doi.org/10.3389/fpls.2022.840900 [Google Scholar] [PubMed] [CrossRef]
7. Rahman, M. A., Alam, I., Kim, Y. G., Ahn, N. Y., Heo, S. H. et al. (2015). Screening for salt-responsive proteins in two contrasting alfalfa cultivars using a comparative proteome approach. Plant Physiology and Biochemistry, 89, 112–122. https://doi.org/10.1016/j.plaphy.2015.02.015 [Google Scholar] [PubMed] [CrossRef]
8. Munns, R., Tester, M. (2008). Mechanisms of salinity tolerance. Annual Review of Plant Biology, 59, 651–681. https://doi.org/10.1146/annurev.arplant.59.032607.092911 [Google Scholar] [PubMed] [CrossRef]
9. Deinlein, U., Stephan, A. B., Horie, T., Luo, W., Xu, G. et al. (2014). Plant salt-tolerance mechanisms. Trends in Plant Science, 19(6), 371–379. https://doi.org/10.1016/j.tplants.2014.02.001 [Google Scholar] [PubMed] [CrossRef]
10. Raza, A., Salehi, H., Rahman, M. A., Zahid, Z., Haghjou, M. M. et al. (2022). Plant hormones and neurotransmitter interactions mediate antioxidant defenses under induced oxidative stress in plants. Frontiers in Plant Science, 13, 961872. https://doi.org/10.3389/fpls.2022.961872 [Google Scholar] [PubMed] [CrossRef]
11. Chauhan, J., Srivastava, J. P., Singhal, R. K., Soufan, W., Dadarwal, B. K. et al. (2022). Alterations of oxidative stress indicators, antioxidant enzymes, soluble sugars, and amino acids in mustard [Brassica juncea (L.) Czern and Coss.] in response to varying sowing time, and field temperature. Frontiers in Plant Science, 13, 875009. https://doi.org/10.3389/fpls.2022.875009 [Google Scholar] [PubMed] [CrossRef]
12. Raza, A., Charagh, S., García-Caparrós, P., Rahman, M. A., Ogwugwa, V. H. et al. (2022). Melatonin-mediated temperature stress tolerance in plants. GM Crops & Food, 13(1), 196–217. https://doi.org/10.1080/21645698.2022.2106111 [Google Scholar] [PubMed] [CrossRef]
13. Lee, K. W., Rahman, M. A., Lee, S. H., Kim, Y. G., Lee, D. G. et al. (2019). Nitric oxide-induced proteomic analysis in rice leaves. Plant Biotechnology Reports, 13, 375–387. https://doi.org/10.1007/s11816-019-00544-1 [Google Scholar] [CrossRef]
14. Islam, M. R., Kamal, M. M., Hossain, M. F., Hossain, J., Azam, M. G. et al. (2023). Drought tolerance in mung bean is associated with the genotypic divergence, regulation of proline, photosynthetic pigment and water relation. Phyton, 92(3), 955–981. https://doi.org/10.32604/phyton.2023.025138 [Google Scholar] [PubMed] [CrossRef]
15. Zhu, G., An, L., Jiao, X., Chen, X., Zhou, G. et al. (2019). Effects of gibberellic acid on water uptake and germination of sweet sorghum seeds under salinity stress. Chilean Journal of Agricultural Research, 79(3), 415–424. https://doi.org/10.4067/S0718-58392019000300415 [Google Scholar] [CrossRef]
16. Silva, M. L. S., de Sousa, H. G., Silva, M. L. S., de Lacerda, C. F., Gomes-Filho, E. (2019). Growth and photosynthetic parameters of saccharine sorghum plants subjected to salinity. Acta Scientiarum Agronomy, 41, 42607. https://doi.org/10.4025/actasciagron.v41i1.42607 [Google Scholar] [CrossRef]
17. Coelho, D. S., Simões, W. L., Salviano, A. M., Mesquita, A. C., Alberto, K. C. (2018). Gas exchange and organic solutes in forage sorghum genotypes grown under different salinity levels. Revista Brasileira de Engenharia Agrícola e Ambiental, 22(4), 231–236. https://doi.org/10.1590/1807-1929/agriambi.v22n4p231-236 [Google Scholar] [CrossRef]
18. Rastogi, A., Kovar, M., He, X., Zivcak, M., Kataria, S. et al. (2020). JIP-test as a tool to identify salinity tolerance in sweet sorghum genotypes. Photosynthetica, 58(SI), 333–343. https://doi.org/10.32615/PS.2019.169 [Google Scholar] [CrossRef]
19. Punia, H., Tokas, J., Malik, A., Singh, S., Phogat, D. S. et al. (2021). Discerning morpho-physiological and quality traits contributing to salinity tolerance acquisition in sorghum [Sorghum bicolor (L.) Moench]. South African Journal of Botany, 140, 409–418. https://doi.org/10.1016/j.sajb.2020.09.036 [Google Scholar] [CrossRef]
20. Khaton, M., Sagar, A., Tajkia, J., Islam, M., Mahmud, M. et al. (2016). Effect of moisture stress on morphological and yield attributes of four sorghum varieties. Progressive Agriculture, 27, 265–271. https://doi.org/10.3329/pa.v27i3.30806 [Google Scholar] [CrossRef]
21. Chiluwal, A., Bheemanahalli, R., Kanaganahalli, V., Boyle, D., Perumal, R. et al. (2020). Deterioration of ovary plays a key role in heat stress? Induced spikelet sterility in sorghum. Plant Cell & Environent, 43(2), 448–462. https://doi.org/10.1111/pce.13673 [Google Scholar] [PubMed] [CrossRef]
22. Sanjari, S., Shobbar, Z. S., Ghanati, F., Afshari-Behbahanizadeh, S., Farajpour, M. et al. (2021). Molecular, chemical, and physiological analyses of sorghum leaf wax under post-flowering drought stress. Plant Physiology and Biochemistry, 159, 383–391. https://doi.org/10.1016/j.plaphy.2021.01.001 [Google Scholar] [PubMed] [CrossRef]
23. Hussain, S., Shaukat, M., Ashraf, M., Zhu, C., Jin, Q. et al. (2019). Salinity stress in arid and semi-arid climates: Effects and management in field crops. Climate change and agriculture. UK: IntechOpen. https://doi.org/10.5772/intechopen.78427 [Google Scholar] [CrossRef]
24. Calone, R., Sanoubar, R., Lambertini, C., Speranza, M., Antisari, L. V. et al. (2020). Salt tolerance and Na allocation in sorghum bicolor under variable soil and water salinity. Plants, 9(5), 561. https://doi.org/10.3390/plants9050561 [Google Scholar] [PubMed] [CrossRef]
25. Sun, Y., Niu, G., Osuna, P., Zhao, L., Ganjehunte, G. et al. (2014). Variability in salt tolerance of Sorghum bicolor L. Agricultural Science, 2(1), 9–21. https://doi.org/10.12735/as.v2i1p9 [Google Scholar] [CrossRef]
26. Dehnavi, A. R., Zahedi, M., Ludwiczak, A., Perez, S. C., Piernik, A. (2020). Effect of salinity on seed germination and seedling development of sorghum (Sorghum bicolor (L.) Moench) genotypes. Agronomy, 10(6), 859. https://doi.org/10.3390/agronomy10060859 [Google Scholar] [CrossRef]
27. Henderson, A. N., Crim, P. M., Cumming, J. R., Hawkins, J. S. (2020). Phenotypic and physiological responses to salt exposure in sorghum reveal diversity among domesticated landraces. American Journal of Botany, 107(7), 983–992. https://doi.org/10.1002/AJB2.1506 [Google Scholar] [PubMed] [CrossRef]
28. Sagar, A., Tajkia, J. E., Haque, M. E., Fakir, M. S. A., Hossain, A. Z. (2019). Screening of sorghum genotypes for salt-tolerance based on seed germination and seedling stage. Fundamental and Applied Agriculture, 4(1), 735–743. https://doi.org/10.3329/jbau.v16i3.39398 [Google Scholar] [CrossRef]
29. Hoagland, D. R., Arnon, D. I. (1950). The water-culture method for growing plants without soil. Circular. California Agricultural Experiment Station, 347, 32. [Google Scholar]
30. Huang, C., Wei, G., Jie, Y., Wang, L., Zhou, H. et al. (2014). Effects of concentrations of sodium chloride on photosynthesis, antioxidative enzymes, growth and fiber yield of hybrid ramie. Plant Physiology and Biochemistry, 76, 86–93. https://doi.org/10.1016/j.plaphy.2013.12.021 [Google Scholar] [PubMed] [CrossRef]
31. Haque, M. S., Kjaer, K. H., Rosenqvist, E., Sharma, D. K., Ottosen, C. O. (2014). Heat stress and recovery of photosystem II efficiency in wheat (Triticum aestivum L.) cultivars acclimated to different growth temperatures. Environmental and Experimental Botany, 99, 1–8. https://doi.org/10.1016/j.envexpbot.2013.10.017 [Google Scholar] [CrossRef]
32. Sun, H., Liu, S., Chen, K., Li, G. (2021). Spectrophotometric determination of chlorophylls in different solvents related to the leaf traits of the main tree species in Northeast China. Proceeding of the IOP Conference Series on Earth and Environmental Science, vol. 836, 012008. Weihai, China. https://doi.org/10.1088/1755-1315/836/1/012008 [Google Scholar] [CrossRef]
33. Kabir, A. H., Das, U., Rahman, M. A., Lee, K. W. (2021). Silicon induces metallochaperone-driven cadmium binding to the cell wall and restores redox status through elevated glutathione in Cd-stressed sugar beet. Physiologia Plantarum, 173(1), 352–368. https://doi.org/10.1111/ppl.13424 [Google Scholar] [PubMed] [CrossRef]
34. Rahman, M. A., Kabir, A. H., Song, Y., Lee, S. H., Hasanuzzaman, M. et al. (2021). Nitric oxide prevents fe deficiency-induced photosynthetic disturbance, and oxidative stress in alfalfa by regulating Fe acquisition and antioxidant defense. Antioxidants, 10(10), 1556. https://doi.org/10.3390/antiox10101556 [Google Scholar] [PubMed] [CrossRef]
35. Bates, L. S., Waldren, R., Teare, I. D. (1973). Rapid determination of free proline for water stress studies. Plant and Soil, 39, 205–207. https://doi.org/10.1007/BF00018060 [Google Scholar] [CrossRef]
36. Mwadzingeni, L., Shimelis, H., Tesfay, S., Tsilo, T. J. (2016). Screening of bread wheat genotypes for drought tolerance using phenotypic and proline analyses. Frontiers in Plant Science, 7, 1276. https://doi.org/10.3389/fpls.2016.01276 [Google Scholar] [PubMed] [CrossRef]
37. Mushtaq, N. U., Saleem, S., Tahir, I., Rehman, R. U. (2023). Analysis of salt stress mitigation by selenium application in proso millet. Journal of Soil Science and Plant Nutrition, 23, 881–899. https://doi.org/10.1007/s42729-022-01090-6 [Google Scholar] [CrossRef]
38. Saleem, S., Mushtaq, N. U., Shah, W. H., Rasool, A., Hakeem, K. R. et al. (2021). Morpho-physiological, biochemical and molecular adaptation of millets to abiotic stresses: A review. Phyton-International Journal of Experimental Botany, 90(5), 1363–1385. https://doi.org/10.32604/phyton.2021.014826 [Google Scholar] [CrossRef]
39. Mushtaq, N. U., Alghamdi, K. M., Saleem, S., Tahir, I., Bahieldin, A. et al. (2023). Exogenous Zinc mitigates salinity stress by stimulating proline metabolism in proso millet (Panicum miliaceum L.). Frontiers in Plant Science, 14, 417. https://doi.org/10.3389/fpls.2023.1053869 [Google Scholar] [PubMed] [CrossRef]
40. Rasool, A., Shah, W. H., Mushtaq, N. U., Saleem, S., Hakeem, K. R. et al. (2022). Amelioration of salinity induced damage in plants by selenium application: A review. South African Journal of Botany, 147, 98–105. https://doi.org/10.1016/j.sajb.2021.12.029 [Google Scholar] [CrossRef]
41. Mushtaq, N. U., Alghamdi, K. M., Saleem, S., Shajar, F., Tahir, I. et al. (2022). Selenate and selenite transporters in proso millet: Genome extensive detection and expression studies under salt stress and selenium. Frontiers in Plant Science, 13, 1060154. https://doi.org/10.3389/fpls.2022.1060154 [Google Scholar] [PubMed] [CrossRef]
42. Mushtaq, N. U., Saleem, S., Rasool, A., Shah, W. H., Hakeem, K. R. et al. (2021). Salt stress threshold in millets: Perspective on cultivation on marginal lands for biomass. Phyton-International Journal of Experimental Botany, 90(1), 51–64. https://doi.org/10.32604/phyton.2020.012163 [Google Scholar] [PubMed] [CrossRef]
43. Kabir, A. H., Ela, E. J., Bagchi, R., Rahman, M. A., Peiter, E. et al. (2023). Nitric oxide acts as an inducer of strategy-I responses to increase Fe availability and mobilization in Fe-starved broccoli (Brassica oleracea var. oleracea). Plant Physiology and Biochemistry, 194, 182–192. https://doi.org/10.1016/j.plaphy.2022.11.018 [Google Scholar] [CrossRef]
44. Nounjan, N., Chansongkrow, P., Charoensawan, V., Siangliw, J. L., Toojinda, T. et al. (2018). High performance of photosynthesis and osmotic adjustment are associated with salt tolerance ability in rice carrying drought tolerance QTL: Physiological and co-expression network analysis. Frontiers in Plant Science, 9, 1135. https://doi.org/10.3389/fpls.2018.01135 [Google Scholar] [PubMed] [CrossRef]
45. Assaha, D. V. M., Ueda, A., Saneoka, H., Al-Yahyai, R., Yaish, M. W. et al. (2017). The role of Na+ and K+ transporters in salt stress adaptation in glycophytes. Frontiers in Physiology, 8, 509. https://doi.org/10.3389/fphys.2017.00509 [Google Scholar] [PubMed] [CrossRef]
46. Mangal, V., Lal, M. K., Tiwari, R. K., Altaf, M. A., Sood, S. et al. (2023). Molecular insights into the role of reactive oxygen, nitrogen and sulphur species in conferring salinity stress tolerance in plants. Journal of Plant Growth Regulation, 42, 554–574. https://doi.org/10.1007/s00344-022-10591-8 [Google Scholar] [CrossRef]
47. Wang, X., Zhang, M. M., Wang, Y. J., Gao, Y. T., Li, R. et al. (2016). The plasma membrane NADPH oxidase OsRbohA plays a crucial role in developmental regulation and drought-stress response in rice. Physiologia Plantarum, 156(4), 421–443. https://doi.org/10.1111/ppl.12389 [Google Scholar] [PubMed] [CrossRef]
48. Krasensky, J., Jonak, C. (2012). Drought, salt, and temperature stress-induced metabolic rearrangements and regulatory networks. Journal of Experimental Botany, 63(4), 1593–1608. https://doi.org/10.1093/jxb/err460 [Google Scholar] [PubMed] [CrossRef]
49. Hayat, S., Hayat, Q., Alyemeni, M. N., Wani, A. S., Pichtel, J. et al. (2012). Role of proline under changing environments. Plant Signaling & Behavior, 7(11), 1456–1466. https://doi.org/10.4161/psb.21949 [Google Scholar] [PubMed] [CrossRef]
50. Rahman, M. A., Yong-Goo, K., Iftekhar, A., Liu, G. S., Hyoshin, L. et al. (2016). Proteome analysis of alfalfa roots in response to water deficit stress. Journal of Integrative Agriculture, 15(6), 1275–1285. https://doi.org/10.1016/S2095-3119(15)61255-2 [Google Scholar] [CrossRef]
51. Kose, A., Oguz, O., Ozlem, B., Ferda, K. (2018). Application of multivariate statistical analysis for breeding strategies of spring safflower (Carthamus tinctorius L.). Turkish Journal of Field Crops, 23(1), 12–19. https://doi.org/10.17557/tjfc.413818 [Google Scholar] [CrossRef]
52. Al-Ashkar, I., Alderfasi, A., Romdhane, W. B., Seleiman, M. F., El-Said, R. A. et al. (2020). Morphological and genetic diversity within salt tolerance detection in eighteen wheat genotypes. Plants, 9(3), 287. https://doi.org/10.3390/plants9030287 [Google Scholar] [PubMed] [CrossRef]
53. Maqbool, M. M., Wahid, A., Ali, A., Khan, S., Irshad, S. et al. (2020). Screening of maize hybrids against salt stress under hydroponic culture. Cereal Research Communications, 48, 49–55. https://doi.org/10.1007/s42976-020-00013-4 [Google Scholar] [CrossRef]
54. Punia, H., Tokas, J., Mor, V. S., Bhuker, A., Malik, A. et al. (2021). Deciphering reserve mobilization, antioxidant potential, and expression analysis of starch synthesis in sorghum seedlings under salt stress. Plants, 10(11), 2463. https://doi.org/10.3390/plants10112463 [Google Scholar] [PubMed] [CrossRef]
55. Ali, A. Y. A., Ibrahim, M. E. H., Zhou, G., Nimir, N. E. A., Jiao, X. (2020). Exogenous jasmonic acid and humic acid increased salinity tolerance of sorghum. Agronomy Journal, 112(2), 871–884. https://doi.org/10.1002/agj2.20072 [Google Scholar] [CrossRef]
56. Punia, H., Tokas, J., Bhadu, S., Mohanty, A. K., Rawat, P. et al. (2020). Proteome dynamics and transcriptome profiling in sorghum [Sorghum bicolor (L.) Moench] under salt stress. 3 Biotech, 10(9), 412. https://doi.org/10.1007/s13205-020-02392-1 [Google Scholar] [PubMed] [CrossRef]
57. Himani, B. S., Tokas, J. (2019). Variation in structural carbohydrates of forage sorghum [Sorghum bicolor (L.) Moench] under saline conditions. Forage Research, 45(2), 123–127. [Google Scholar]
Cite This Article
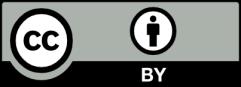
This work is licensed under a Creative Commons Attribution 4.0 International License , which permits unrestricted use, distribution, and reproduction in any medium, provided the original work is properly cited.