Open Access
ARTICLE
The Physiological Mechanisms Underlying N2-Fixing Common Bean (Phaseolus vulgaris L.) Tolerance to Iron Deficiency
1
Faculty of Sciences and Techniques, University of Kairouan, Sidi Bouzid, 9100, Tunisia
2
Laboratory of Ecosystems and Biodiversity in Arid Land of Tunisia, Faculty of Sciences, University of Sfax, Sfax, Tunisia
* Corresponding Author: Abdelmajid Krouma. Email: -kairouan.tn
(This article belongs to the Special Issue: Abiotic and Biotic Stress Tolerance in Crop)
Phyton-International Journal of Experimental Botany 2023, 92(7), 2133-2150. https://doi.org/10.32604/phyton.2023.029048
Received 29 January 2023; Accepted 03 March 2023; Issue published 29 May 2023
Abstract
Iron is an essential element for plants as well as all living organisms, functioning in various physiological and biochemical processes such as photosynthesis, respiration, DNA synthesis, and N2 fixation. In the soil, Fe bioavailability is extremely low, especially under aerobic conditions and at high pH ranges. In contrast, plants with nodules on their roots that fix atmospheric nitrogen need much more iron. To highlight the physiological traits underlying the tolerance of N2-fixing common bean to iron deficiency, two genotypes were hydroponically cultivated in a greenhouse: Coco nain (CN) and Coco blanc (CB). Plants were inoculated with an efficient strain of Rhizobium tropici, CIAT899, and received a nutrient solution added with 0 µM Fe (severe Fe deficiency, SFeD), 5 µM Fe (moderate Fe deficiency, MFeD) or 45 µM Fe (control, C). Several physiological parameters related to photosynthesis and symbiotic nitrogen fixation were then analyzed. Iron deficiency significantly reduced whole plant and nodule growth, chlorophyll biosynthesis, photosynthesis, leghemoglobin (LgHb), nitrogenase (N2ase) activity, nitrogen, and Fe nutrition, with some genotypic differences. As compared to CB, CN maintained better Fe allocation to shoots and nodules, allowing it to preserve the integrity of its photosynthetic and symbiotic apparatus, thus maintaining the key functional traits of the plant metabolism (chlorophyll biosynthesis and photosynthesis in shoots, leghemoglobin accumulation, and nitrogenase activity in root nodules). Plant growth depends on photosynthesis, which needs to be supplied with sufficient iron and nitrogen. Fe deficiency stress index (FeD-SI) and Fe use efficiency (FeUE) are two physiological traits of tolerance that discriminated the studied genotypes.Keywords
Nomenclature
C | Control |
CB | Coco blanc |
CN | Coco nain |
DAG | Days after germination |
DW | Dry weight |
FeD-SI | Fe deficiency stress index |
FeUE | Fe use efficiency |
FW | Fresh weight |
LgHb | Leghemoglobin |
MFeD | Moderate iron deficiency |
PSI | Photosystem I |
PSII | Photosystem II |
SFeD | Severe iron deficiency |
Iron, which represents the fourth most common element in the Earth’s crust, is weakly available for root uptake. However, approximately 30% of the world’s cultivated soils are considered Fe deficient due to the dominance of the insoluble form [1,2]. In calcareous soils, known by their alkalinity, the soil solution does not provide more than 10% of the plant requirements for Fe [3]. Li et al. [4] and Mahender et al. [1] reported that, over neutrality, a one-unit increase in soil pH reduced iron availability by 95%. Inadequate production of chlorophyll and hampered chloroplast development are observed under insufficient iron nutrition, then specific symptoms of Fe-chlorosis are induced [5–8]. In fact, as a co-factor for enzymes such as heme, iron-sulfur (Fe–S) clusters, ferredoxin (Fd), superoxide dismutase, and peroxidase, Fe plays a key role in plant metabolism [1,9,10]. Fe functions in several physiological and biochemical cellular processes, which include photosynthesis, respiration, N2 fixation, and protein formation in plants [11,12]. For photosynthesis, the function of various cytochromes containing heme Fe in the electron transport chain is well established. Fe is involved in the functioning of ferredoxin, cytochrome-b6f, PSI, and PSII [13]. Through these proteins and enzymes, Fe acts on photosynthesis, mitochondrial respiration, nucleic acid synthesis, internal metal balance, the maintenance of structural and functional integrity of proteins, and maintains the normal growth and development of plants [1,10]. Iron deficiency can disrupt electron transfer in the electron transport chain, reduce ATP and NADPH production, and disturb the Calvin cycle, leading to a reduction in photosynthesis [9,14].
Legumes are known to be sensitive to Fe deficiency, particularly when they depend on symbiotic nitrogen fixation for N nutrition [15,16]. Fe is involved in the key Fe-containing proteins, leghemoglobin, and nitrogenase, in the root nodules [17,18]. Previous research has found that this micronutrient can inhibit Rhizobial bacteria survival and multiplication in root nodules, as well as whole plant and nodule growth and nodule initiation [15,16]. For symbiotic nitrogen fixation in root nodules, iron is required as an essential micronutrient because of its redox properties. In lupin [19,20] and common bean [15,16] plants, low iron availability severely affects key metabolic functions and decreases nodule number and biomass. In soybeans, nodules benefit from 45% of the total plant need for iron [21]. On the other hand, nodule initiation was less sensitive to Fe deficiency in Arachis hypogaea than in Lupinus angustifolius [22].
Many techniques have been used to increase Fe concentrations in crops and improve their Fe nutrition, including genetic engineering, conventional breeding, and agronomic approaches [23]. Direct plant pulverization with Fe solutions has demonstrated its effectiveness for rapid plant growth [4] and improved yield and fruit quality [23,24]. Overall researchers have focused on this direct, but expensive, polluting, and unsustainable approach for iron chlorosis correction, leaving aside other efficient approaches [25]. One of these is the exploration of the available plant resources on the basis of their differences in response to iron deficiency in order to highlight tolerant genotypes and identify useful traits of tolerance [26]. In a previous study on four common bean genotypes subjected to iron deficiency, we identified genotypic variability in response to this nutritional constraint based on their rhizosphere acidification and Fe-chelate reductase activity [27]. CN was identified as a tolerant genotype, whereas CB was identified as sensitive. In the present study, we granted a special interest to this symbiosis regarding its key metabolic reactions, photosynthesis, symbiotic nitrogen fixation, and their related parameters and interrelationships, in response to iron deficiency. The main objective was to highlight the common thread that connects shoot photosynthetic activity to nodule symbiotic nitrogen fixation and identify some physiological traits of common bean tolerance to limited Fe nutrition.
2.1 Plant Material and Growth Condition
Two common bean genotypes, CB, sensitive, and CN, tolerant, were inoculated with an efficient strain of Rhizobium tropici CIAT 899 (originating from the International Center of Tropical Agriculture, Colombia) and grown individually in the glasshouse under natural light in 1 L pots filled with the following nutrient solution (Hewitt, 1966): KH2PO4 (1.60 mM), MgSO4 (1.50 mM), K2SO4 (1.50 mM), CaSO4 (3.50 mM), H3BO3 (4 µM), MnSO4 (4 µM), ZnSO4 (1 µM), CuSO4 (1 µM), CoCl2 (0.12 µM), (Na)6(Mo)7O24 (0.12 µM). Iron was added separately as K–Fe–EDTA form in the following concentrations: 0, 5 and 45 µM. The pH of the solution was maintained at neutrality with CaCO3. During the first 2 weeks, i.e. before the beginning of nitrogen fixation, the plant nutrient solution was complemented with 2 mM urea to meet the plant’s nitrogen need after exhaustion of cotyledonous reserves [28]. Thereafter, the N-free nutrient solution was renewed weekly. It was aerated with a flow of 400 ml mn−1 of filtered air via a compressor and ‘spaghetti tube’ distribution system. The day/night temperatures and relative humidity were 25/20 ± 5°C and 65/85 ± 5%, respectively. Two harvests were made: the initial one at the appearance of the first trifoliate leaf at 14 days after germination (DAG), the second at the beginning of flowering at 45 DAG. Before the final harvest (45 DAG), nondestructive measurements (Spad index, photosynthesis, and nitrogenase activity) were made. At each harvest, plants were separated into leaves, stems, roots, and nodules. The last organs were soaked in a 0.01 M CaCl2 solution [29,30] and washed thoroughly and successively in 3 baths of ultra-pure water in order to avoid the contamination of roots and nodules with elements from the nutrient solution. All plant materials were dried at 60°C for 72 h, then pulverized into a fine powder, except nodules organs allowed to leghemoglobin analysis.
2.2 Leghaemoglobin Determination
Nodules (500 mg) were homogenized in aliquots of Drabkin’s reagent (10 ml) and leghaemoglobin was quantified spectrophotometrically at 540 nm. Bovin haemoglobin was used as a standard, and values are expressed as milligrams per gram of nodule fresh weight. The quantification of leghaemoglobin of the preparation of the Drabkin’s solution was described in detail by Krouma et al. [31].
2.3 Nondestructive Measurements
Relative leaf chlorophyll concentrations were estimated in vivo using a SPAD-502 (Konica-Minolta, Japan) prior to measurements of gas exchange, on the third fully expanded apical leaves. Measurements were made on ten plants of each soil and genotype. Values are presented as SPAD units.
The same third fully expanded apical leaves used for Spad index were used for gas exchange measurements using an LI-6400 (LI-COR, Inc., Nebraska, USA) portable gas exchange system. Ten plants of each genotype and soil were used. A saturating light of 1000 μmol m−2 s−1 was used to induce photosynthesis. This light was fitted to the standard 6 cm2 clamp on the leaf chamber. The other parameters were maintained constant, sample pCO2 at 362 mbar, flow rate at 500 μmol s−1, and temperature at 25°C.
Nitrogenase (EC 1.7.99.2) activity of the nodules (Acetylene Reduction Activity–ARA) was measured in situ as previously described [28]. To avoid nodule disturbance, the level of the solution was lowered to 40% of the bottle volume 1 day before the assay. The aliquots were analyzed for ethylene in a Hewlett Packard 4890 gas chromatograph equipped with a Poropak T Column and a flame ionization detector. The ARA values were computed as C2H4 concentration by flow rate and expressed as μmol C2H4 h−1 plant−1.
2.4 Mineral Analysis (N and Fe)
For nitrogen concentration and repartition in plant organs, 50 mg of fine dry matter of each organ were digested in concentrated H2SO4, then N was determined according to Kjeldahl method. The symbiotic nitrogen fixation (SNF) was estimated as the difference between N quantities (mmol plant−1) at initial harvest (14 DAG) and final harvest (45 DAG).
For active Fe concentration, 25 mg of fine powder of dry plant organ were shacked in 10 ml of HCl (1N) for 24 h then filtered. Active iron (Fe2+) content was determined by the atomic absorption spectrophotometry method according to Koseoglu et al. [32].
2.5 Criteria used and Calculation
FeD-SI: Fe deficiency stress index, calculated as follow: FeD-SI = 1 − (Ps/Pc), where
Ps: parameter in stressed plants (An, DW, spad, LgHb, N, N2ase)
Pc: parameter in control plants (An, DW, spad, LgHb, N, N2ase)
FeUE-An: Fe use efficiency for photosynthesis, calculated as the ratio of net photosynthesis (µmol CO2 m−2 s−1) to shoot Fe concentration (mg g−1 DW)
FeUE-DW: Fe use efficiency for plant growth, calculated as the ratio of total biomass (g plant−1) to shoot Fe concentration (mg g−1 DW)
FeUE-DWnod: Fe use efficiency for nodules growth, calculated as the ratio of nodules biomass (mg plant−1) to shoot Fe concentration (mg g−1 DW)
FeUE-LgHb: Fe use efficiency for LgHb accumulation in nodules, calculated as the ratio of LgHb concentration (mg g−1 nod FW) to shoot Fe concentration (mg g−1 DW)
FeUE-N: Fe use efficiency for symbiotic nitrogen fixation, calculated as the ratio of total nitrogen fixed (mmoml plant−1) to shoot Fe concentration (mg g−1 DW)
FeUE-Spad: Fe use efficiency for chlorophyll biosynthesis, calculated as the ratio of spad index to shoot Fe concentration (mg g−1 DW)
The experiment was conducted in a completely randomized design with three treatments, two genotypes and ten replicates. All data are presented as mean ± standard error. Analysis of variance (ANOVA, one-way) was performed to determine whether the effects of treatments on the respective factor were significant. The significance of differences among treatment means was determined by Fisher’s least significant difference test (LSD) at 5%. Treatment means were declared significant when the difference between any two treatments was greater than the LSD value generated from the ANOVA. They are marked by different letters in the figures.
The daily monitoring of plant behavior showed the apparition of specific symptoms of iron chlorosis on young leaves after 6 days in CB and 10 days in CN under severe Fe deficiency (SMFeD), and after 11 days in CB and 15 days in CN under moderate Fe deficiency (MFeD). Fe chlorosis in CB is more severe than in CN and evolves towards necrosis in SFeD (Fig. 1).
Figure 1: Common bean plant aspect when subjected to severe iron deficiency (SFeD), moderate iron deficiency (MFeD), or sufficiently supplied with iron (control, C). CN: coco nain, CB: coco blanc
In order to confirm these genotypic differences, we measured the Spad index. Fig. 2 shows that submitting plants to iron deficiency decreased significantly the spad index in the two genotypes. However, CN was less affected than CB. The spad index decreased by 26% and 14% in ARA 14 and by 50% and 22% in CB, respectively, under SFeD and MFeD, as compared to control plants. Even under Fe deficiency, CN accumulated 63% and 20% more chlorophyll than CB, respectively, under SFeD and MFeD.
Figure 2: Spad index in common bean plants subjected to severe (SFeD or moderate iron deficiency MFeD, or sufficiently subjected with iron (control)). Within columns, means with the same letter are not significantly different at α = 0.05 according to Fisher’s Least Significant Difference. Bars on the columns represent the standard error of the mean (n = 10)
Whole plant growth represented as total biomass production showed the same schema of variation as spad with a significant decrease under Fe deprivation (Fig. 3A). DW decreased by 32% and 9% in ARA 14 and by 43% and 22% in CB, respectively, under SFeD and MFeD, as compared to control plants. Under these conditions of Fe deficiency, CN produced 52% and 47% more biomass than CB, respectively under SFeD and MFeD. When quantified separately, nodules biomass expressed the same response to Fe deficiency as the whole plant. A significant decrease under SFeD reaching −52% and −63%, respectively, in CN and CB; and a less pronounced effect under MFeD (−11% and −18%, respectively, in CN and CB). Independently of Fe deficiency severity, CN is usually less affected than CB (Fig. 3B).
Figure 3: Whole plant (A) and nodules (B) growth in common bean plants subjected to severe (SFeD or moderate iron deficiency MFeD, or sufficiently subjected with iron (control)). Within columns, means with the same letter are not significantly different at α = 0.05 according to Fisher’s Least Significant Difference. Bars on the columns represent the standard error of the mean (n = 10)
Measurements made on gas exchange parameters demonstrated that limiting iron availability decreased net photosynthetic activity (Pn) in the two common bean genotypes (Fig. 4A). However, CN maintains its superiority, as compared to CB independently of iron concentration in the medium. Pn decreased by 67% at SFeD and by 29% at MFeD in CN, and by 77% at SFeD and by 43% at MFeD in CB, as compared to control plants. Under limited iron availability, net photosynthesis in CN exceeded that of CB by 58% at SFeD and by 41% at MFeD. For evapotranspiration (ET), the same pattern of variation was observed (Fig. 4B). In fact, ET decreased by 64% and 29%, respectively, at SFeD and MFeD in CN, as compared to control plants; and by 68% and 43%, respectively, at SFeD and MFeD in CB, as compared to control plants. ET in CN exceeded that in CB by 25% and 38%, respectively, at SFeD and MFeD. Iron deficiency decreased also significantly stomatal conductance (SC) in the two studied genotypes (Fig. 4C). SC decreased by 84% and 22% in CN and by 93% and 37% in CB, respectively, at SFeD and MFeD as compared to control plants.
Figure 4: Gas exchange parameters expressed as (A) net photosynthetic assimilation (An), (B) evapotranspiration (ET) and (C) stomatal conductance (SC) in common bean plants subjected to severe (SFeD or moderate iron deficiency MFeD, or sufficiently subjected with iron (control)). Within columns, means with the same letter are not significantly different at α = 0.05 according to Fisher’s Least Significant Difference. Bars on the columns represent the standard error of the mean (n = 10)
The symbiotic performance of common genotypes was estimated with two methods. The first one is a non-destructive measurement that estimated the nitrogenase activity (Acetylene reduction activity, ARA), and the second method is the quantification of nitrogen in the plant (symbiotically fixed). Fig. 5A showed that Fe deficiency significantly decreased nitrogenase activity in the two common bean genotypes. In CN, ARA decreased by 82% and 42%, whereas it decreased by 90% and 56% in CB, respectively, at SFeD and MFeD. Nitrogenase activity was more than two times higher in CN, as compared to CB under SFeD, 1.6 times higher at MFeD, confirming its superiority at this level. These results are confirmed by the calculated nitrogen fixed in the plants. CN fixed 43% more nitrogen at SFeD and more than 100% more nitrogen at MFeD, as compared to CB (Fig. 5B). Besides these genotypic differences, iron deficiency hampered nitrogen fixation in the two genotypes. Accumulated N in the plants decreased by 63% and 12% in CN, and by 66% and 49% in CB, respectively, at SFeD and MFeD, as compared to control plants.
Figure 5: Variation of the nitrogenase activity estimated by the acetylene reduction activity (ARA) in root nodules (A), symbiotic nitrogen fixation estimated by quantified total N in plants (B) and leghemoglobin concentration in root nodules (C) of two common bean varieties (CN and CB) according to iron availability. Within columns, means with the same letter are not significantly different at α = 0.05 according to Fisher’s Least Significant Difference. Bars on the columns represent the standard error of the mean (n = 10)
Leghemoglobin (LgHb) is an important Fe-containing protein that controls O2 diffusion in the bacteroid and protects nitrogenase activity. For this purpose, we analyzed this hemoprotein in the root nodules of common beans, in connection with Fe availability. Fig. 5C demonstrated that decreasing Fe concentration in the nutrient solution significantly decreased LgHb in common bean root nodules. In CN, LgHb concentration decreased by 62% and 46% in CN, respectively, at SFD and MFeD, as compared to control plants, whereas it decreased by 74% and 62% in CB, respectively, at SFeD and MFeD, as compared to control plants. The superiority of CN was also maintained at this level with 87% and 81% more LgHb than CB, respectively, at SFeD and MFeD.
The analysis of Fe-extractible fraction in the different plant organs demonstrated that Fe concentration decreased in shoots, roots, and nodules of CN and CB at SFeD and MFeD (Fig. 6). Nodules and roots accumulated the most available Fe (2–3 times more than shoots) in conditions of limited iron availability. However, when quantifying the accumulated iron in the plant, we remark that CN accumulated more Fe in its plant organs (40% to 70% more Fe in shoots and roots, 3.5 times in nodules at MFeD, and 54% to 80% in the total plant, depending on the severity of iron deficiency), than CB (Table 1).
Figure 6: Effect of iron concentration in the medium on the extractible Fe concentrations in plant organs. Within columns, means with the same letter are not significantly different at α = 0.05 according to Fisher’s Least Significant Difference. Bars on the columns represent the standard error of the mean (n = 10)
To verify our hypothesis of physiological dissection of N2-fixing common bean response to iron deficiency, we established a number of correlations. Fig. 7A which connects plant growth to net photosynthesis showed a strict positive correlation between the two genotypes. CN maintains usually higher photosynthetic activity with better biomass production. Furthermore, plant growth is highly dependent on symbiotic nitrogen fixation (Fig. 7B), with specific performance of CN, as compared to CB.
Figure 7: Relationship between biomass production and net photosynthesis (A), and between plant growth and symbiotically fixed N (B). Vertical and horizontal bars represent ± standard errors of means of 10 replicates
When studying the net photosynthesis as a function of the shoot Fe content, we obtained a close and positive correlation between these two parameters, which justifies their interdependence. In fact, as reported in Table 1, the genotype CN showed also higher potentialities of iron allocation to shoots, particularly under limiting Fe availability, with better photosynthesis (Fig. 8A). In the same way, the correlation between the fixed nitrogen calculated in the whole plant and the nitrogenase activity showed a strict positive relationship (Fig. 8B). The genotype CN maintained its superiority on CB even under limited iron availability, expressing greater nitrogenase activity and providing it with a higher amount of nitrogen.
Figure 8: Relationship between photosynthesis and shoot Fe (A), and between symbiotically fixed nitrogen and N2ase (estimated by ARA) activity (B). Vertical and horizontal bars represent ± standard errors of means of 10 replicates
Finally, we correlated nitrogenase activity with net photosynthesis (Fig. 9A) and with Fe in nodules (Fig. 9B). For the first correlation, a close positive relationship with the same degree in the two genotypes was established, reflecting the dependence of the nitrogenase activity on photosynthesis. For the second correlation, the relationship is positive but weak, in CB and positive and strict in CN. The latter allocates more iron to their nodules and develops better nitrogenase activity.
Figure 9: Relationship between N2ase (estimated by ARA) activity and photosynthesis (A), and between N2ase activity and nodules Fe (B). Vertical and horizontal bars represent ± standard errors of means of 10 replicates
In order to continue in this deep analysis of the genotypic differences in the response to iron deficiency in common inoculated with the efficient strain of Rhizobium tropici, CIAT899, we calculated the Fe deficiency stress index (FeD-SI) based on the different studied parameters (Table 2). Our results demonstrated that FeD-SI increased significantly with increasing Fe deficiency severity. When moving from MFeD to SFeD, FeD-SI increased 1.9 and 2.3 times when calculated based on spad, 2.3 and 1.8 times when calculated based on An, 5.3 and 1.4 times when calculated based on fixed nitrogen, 3.6 and 2 times when calculated based on plant growth, 1.4 and 1.2 times when calculated based on the LgHb and 2 and 1.6 times when calculated based on N2ase activity, respectively, in CN and CB. However, CN expressed usually lower values than CB. In a second time, we calculated Fe use efficiency for chlorophyll biosynthesis (FeUE-spad), photosynthesis (FeUE-An), nitrogen fixation (FeUE-N), plant (FeUE-DW) and nodules growth (FeUE-nodDW) and for LgHb accumulation (FeUE-LgHb). Table 3 showed that CN maintained its superiority over CB regarding all these parameters. CN expressed usually higher efficiency of Fe use for these physiological traits.
The results showed that exposing common bean plants to iron deficiency-induced symptoms of iron deficiency that were more severe and precocious in CB than CN, in SFeD as well as in MFeD. Furthermore, chlorophyll biosynthesis and photosynthesis decreased in the two genotypes while maintaining the superiority of CN. In fact, iron is known to be involved in chlorophyll biosynthesis and photosynthesis [11,33,34], as well as the metabolism of some plastidial proteins [26]. Therefore, the observed symptoms of Fe chlorosis and the associated reduction of chlorophyll biosynthesis and net photosynthetic assimilation in this study can be explained by the drastic decrease in iron availability for these key functions. This is confirmed by the drastic decrease in the physiologically active form of iron (Fe2+, Fig. 6) where CN maintained a better concentration as compared to CB. In fact, Fe is directly and indirectly involved in numerous key proteins and enzymes of the photosynthetic mechanism, where Photosystem II (PS-II) contains Fe proteins and loses its efficiency if Fe availability is limited because of the failure of the electron transfer chain [35,36]. Photosystem I (PS-I) also requires Fe as Fe–S clusters. The electron transfer chain requires three forms of Fe as protein cofactors: heme, nonheme, and Fe–S clusters. As a result, Fe deficiency causes stunted roots, leaf chlorosis, and poor maturation in plants [37,38]. Ferhi et al. [39] reported that direct as well as induced iron deficiency reduced the spad index, photosynthesis, and chlorophyll fluorescence in pea plants, and the genotypes studied are differently affected. Krouma et al. [27] working on four common ban genotypes, demonstrated that symptoms of iron chlorosis were more severe and more precocious in the most sensitive genotype, BAT477. According to our results that showed an important recuperation of these physiological functions under MFeD, Valentinuzzi et al. [40] demonstrated that the application of a low Fe concentration to Fe-deficient plants (1 μM) is sufficient to promote the reactivation of the plant key functions (e.g., photosynthesis) with a consequent improvement in biomass accumulation. Otherwise, iron deficiency reduced chlorophyll biosynthesis and inhibited Fe translocation to young leaves. Taken together, the above-analyzed results demonstrated that CN is more tolerant to Fe deficiency than CB. The first genotype is characterized by better Fe allocation to shoots, allowing it to maintain higher chlorophyll, photosynthesis, and plant growth. As shown in Table 2, the quantity of Fe in the shoots of CN was 1.4 and 1.7 times higher than that in CB, respectively, at SFeD and MFeD.
Other than the shoot metabolic reactions, common bean plants inoculated with Rhizobium tropici develop root nodules that fix atmospheric nitrogen. The current results demonstrated that iron deficiency significantly decreased nodule biomass and disturbed nitrogen fixation. However, the genotypic differences previously observed were maintained at this level. Under conditions of limited Fe availability, CN produces more nodule biomass, has nitrogenase activity that is 1.6–2 times that of CB, and fixes 43%–100% more nitrogen than CB. Otherwise, CN maintained higher nodule Fe concentrations as compared to CB subjected to iron deficiency, with 3.5 times more iron in these organs (Table 1). Thus, we can explain the symbiotic superiority of CN as compared to CB by its greater capability of Fe allocation to these organs to support their growth and nitrogenase activity. Chikoti et al. [41] reported that iron is considered an essential micronutrient for symbiotic nitrogen fixation in legume plants because of its redox properties. Numerous studies have found that iron deficiency reduces plant growth, nodule biomass, and number in lupine [20], lentil [42], peanut [43], and common bean [16]. O’Hara et al. [43] demonstrated that nodule development is more sensitive to Fe deficiency than nodule initiation. Brear et al. [18] demonstrated that the demand for iron is higher for nodule initiation, development, and activities than that for host plant growth. This is due to the involvement of iron in the key Fe-containing proteins (leghemoglobin and nitrogenase) of the symbiotic nitrogen fixation mechanism [18]. Otherwise, Fe participates in hemoglobin biosynthesis, which is more important in the tolerant genotype CN than in the sensitive genotype CB. Such hemoproteins play a key role in O2 concentration and transport in bacteroids, and their presence in these organs can guarantee nitrogenase activity and nitrogen fixation. Thus, we can suggest that the main cause of the observed differences in LgHb accumulation and nitrogen fixation between the contrasting genotypes resides at the level of Fe allocation to nodules. This could explain why CN has 1.6- and 1.3-times more nodule biomass, 1.9- and 1.8-times more LgHb, 2.25- and 1.6-times more ARA, and 1.4- and 2.2-times more nitrogen than CB at SFeD and MFeD, respectively. Regarding all these results and explanations related to the photosynthetic and symbiotic apparatus, we can suggest that the basis of the genotypic differences in response to iron deficiency in N2-fixing common bean consists in the capacity of the tolerant genotype to preserve the integrity of its photosynthetic and symbiotic apparatus in order to maintain the key functional traits of the general plant metabolism (chlorophyll biosynthesis and photosynthesis in shoots, leghemoglobin accumulation, and nitrogenase activity in root nodules), allowing it to produce more biomass. Such conditions remain linked to an adequate shoot and nodule supply with iron. Otherwise, symbiotic nitrogen fixation in root nodules is still dependent on organic matter from shoots. Taken together, all these conditions and their interdependence seem to coexist in the tolerant genotype CN. The established correlations in this study (Figs. 7–9) confirm the above-mentioned relationships. Plant growth is highly dependent on net photosynthesis, which is closely dependent on shoot Fe, and on nitrogen fixation, which is dependent on nitrogenase activity. The latter remains closely dependent on photosynthesis as a carbon source and on Fe supply. Thus, a common thread exists in N2-fixing common bean which makes the link between the shoot and root nodules key metabolic functions. The genotype that establishes a good connection between these different components in conditions of iron deficiency succeeds in maintaining good metabolic functioning and then plant growth. The calculated Fe-sensitivity index (FeD-SI) based on the above-treated parameters confirms the observed genotypic differences and the particular tolerance of CN (Table 1). Depending on the spad index, net photosynthesis, nitrogen fixed, plant growth, and LgHb or N2ase activity, the FeD-SI was usually higher in CB than in CN. Thus, the first suggestion that emerges from this study is the usefulness of FeD-SI as a trait of tolerance to iron deficiency, which can be used for further screening programs. On the other hand, the calculated Fe use efficiency for the same physiological parameters clearly discriminated the studied genotypes (Table 2). At SFeD, FeUE-spad, FeUE-An, FeUE-N, FeUE-DW, and FeUE-LgHb were respectively 1.5, 1.34, 3.6, 1.11, and 1.7 times higher in CN, as compared to CB. Therefore, another characteristic of common bean tolerance to Fe deficiency is the efficiency of Fe use for the different metabolic reactions in shoots as well as in nodules. In shoots, iron is directly involved in chlorophyll biosynthesis, electron chain transport, and numerous enzymes and proteins [1,9,10], whereas in nodules, Fe is demonstrated essential as a cofactor for nitrogenase activity, some components of the respiratory chain as well as many other enzymes [44]. Fe is required for the mitochondrial respiratory chain and the biosynthesis of leghemoglobin [45,46]. Gonzalez-Guerrero et al. [45] and Clarke et al. [46] reported that N2-fixing nodules have a very higher requirement for iron than any other plant organ. Thus, we can suggest that limited iron availability in the rhizosphere influences plant growth through the restriction of photosynthesis which produces the necessary organic matter and carbohydrates. However, the latter can be influenced either directly through the restriction of Fe allocation to shoots (limited chlorophyll biosynthesis, electron chain transfer, Fe-dependent enzymes, and proteins…), or indirectly through reduced nitrogen allocation to shoots due to disturbed symbiotic nitrogen fixation (limited LgHb, N2ase, enzymes and Fe-dependent proteins) (Fig. 10). The relative tolerance of CN observed in the present study can be attributed to its ability to efficiently use the low available forms of Fe to support these interdependent physiological functions in shoots (chlorophyll and photosynthesis) and root nodules (N2ase activity). Therefore, in addition to FeD-SI, Fe use efficiency (FeUE), independent of the parameter used for its calculation, is an important trait of iron deficiency tolerance that discriminated the studied genotypes. It can be used for further screening programs.
Figure 10: Schematic representation of proposed iron influences on plant growth in N2-fixing common bean. Low iron availability reduced photosynthesis following the restriction of Fe allocation to shoots (reduction of chlorophyll biosynthesis and functioning of Fe-dependent enzymes and proteins) and following the restriction of shoot supply with nitrogen (reduction of N2ase activity through hampered Fe-dependent enzymes and proteins, Leghemoglobin (LHb), ferredoxin…)
In summary, the present study demonstrated the genotypic differences in response to iron deficiency in N2-fixing common beans. These differences are explained by the capacity of the tolerant genotype to preserve the integrity of its photosynthetic and symbiotic apparatus allowing it to maintain the key functional traits of the plant metabolism (chlorophyll biosynthesis and photosynthesis in shoots, leghemoglobin accumulation and nitrogenase activity in root nodules). These conditions remain strictly related to the adequate shoot and nodule supply with iron. Otherwise, N2ase in nodules is still dependent on organic matter from shoots. Plant growth is highly linked to photosynthesis and symbiotic nitrogen fixation which depends strictly on their supply with Fe. FeD-SI and FeUE are a useful trait of tolerance to iron deficiency that can be used for further screening programs.
Funding Statement: This study was supported by the Ministry of Higher Education and Scientific Research and Conducted within the Framework of the Partnership for Research and Innovation in the Mediterranean Area (PRIMA), Project DiVicia: Use and Management of Vicia Species for Sustainability and Resilience in Biodiversity-Based Farming Systems.
Conflicts of Interest: The author declares that they have no conflicts of interest to report regarding the present study.
References
1. Mahender, A., Swamy, B. P. M., Anandan, A., Ali, J. (2019). Tolerance of iron-deficient and -toxic soil conditions in rice. Plants, 8(2), 31. https://doi.org/10.3390/plants8020031 [Google Scholar] [PubMed] [CrossRef]
2. Dos Santos, M. S., Sanglard, L. M. V. P., Barbosa, M. L., Namorato, F. A., de Melo, D. C. et al. (2019). Silicon nutrition mitigates the negative impacts of iron toxicity on rice photosynthesis and grain yield. Ecotoxicology and Environmental Safety, 189, 110008. https://doi.org/10.1016/j.ecoenv.2019 [Google Scholar] [CrossRef]
3. Mortvedt, J. J. (1991). Correcting iron deficiencies in annual and perennial plants: Present technologies and future prospects. Plant and Soil, 130, 273–279. [Google Scholar]
4. Li, M., Wang, S., Tian, X., Li, S., Chen, Y. et al. (2016). Zinc and iron concentrations in grain milling fractions through combined foliar applications of Zn and macronutrients. Field Crops Research, 187, 135–141. [Google Scholar]
5. Lucena, J. J. (2000). Effects of bicarbonate, nitrate and other environmental factors on iron deficiency chlorosis. A review. Journal of Plant Nutrition, 23, 1591–1606. [Google Scholar]
6. Chatterjee, A., Lovas, S., Rasmussena, H., Goos, R. J. (2017). Foliar application of iron fertilizers to control iron deficiency chlorosis of soybean. Crop Forage Turfgrass Management, 3(1), 1–7. [Google Scholar]
7. Selby-Pham, J., Lutz, A., Moreno-Moyano, L. T., Boughton, B. A., Roessner, U. et al. (2017). Diurnal changes in transcript and metabolite levels during the iron deficiency response of rice. Rice, 10(1), 14. https://doi.org/10.1186/s12284-017-0152-7 [Google Scholar] [PubMed] [CrossRef]
8. Ma, J., Zhang, M., Liu, Z., Chen, H., Li, Y. C. et al. (2019). Effects of foliar application of the mixture of copper and chelated iron on the yield, quality, photosynthesis, and microelement concentration of table grape (Vitis vinifera L.). Scientia Horticulturae, 254, 106–115. https://doi.org/10.1016/j.scienta.2019.04.075 [Google Scholar] [CrossRef]
9. None (2015). Plant nutrition 3: Micronutrients and metals. Plant Cell, 27(5), tpc.115.tt0515. https://doi.org/10.1105/tpc.115.tt0515 [Google Scholar] [CrossRef]
10. Li, M., Zhang, P., Adeel, M., Guo, Z., Chetwynd, A. J. et al. (2021). Physiological impacts of zero valent iron, Fe3O4 and Fe2O3 nanoparticles in rice plants and their potential as Fe fertilizers. Environmental Pollution, 269, 116134. https://doi.org/10.1016/j.envpol.2020.116134 [Google Scholar] [PubMed] [CrossRef]
11. Krouma, A. (2021). Differential response of pea (Pisum sativum L.) genotypes to iron deficiency in relation to the growth, rhizosphere acidification and ferric chelate reductase activities. Australian Journal of Crop Science, 15(6), 925–932. https://doi.org/10.21475/ajcs.21.15.06.p3171 [Google Scholar] [CrossRef]
12. Zhang, X., Zhang, D., Sun, W., Wang, T. (2019). The adaptive mechanism of plants to iron deficiency via iron uptake, transport, and homeostasis. International Journal of Molecular Science, 20, 2424. [Google Scholar]
13. Briat, J. F., Curie, C., Gaymard, F. (2007). Iron utilization and metabolism in plants. Current Opinion in Plant Biology, 10(3), 276–282. https://doi.org/10.1016/j.pbi.2007.04.003 [Google Scholar] [PubMed] [CrossRef]
14. Yadavalli, V., Neelam, S., Rao, A. S., Reddy, A. R., Subramanyam, R. (2012). Differential degradation of photosystem I subunits under iron deficiency in rice. Journal of Plant Physiology, 169(8), 753–759. https://doi.org/10.1016/j.jplph.2012.02.008 [Google Scholar] [PubMed] [CrossRef]
15. Krouma, A., Drevon, J. J., Abdelly, C. (2006). Genotypic variation of N2 fixing common bean (Phaseolus vulgaris L.) in response to iron deficiency. Journal of Plant Physiology, 163, 1094–1100. [Google Scholar] [PubMed]
16. Krouma, A., Slatni, T., Abdelly, C. (2008). Differential tolerance to lime-induced chlorosis of N2-fixing common bean (Phaseolus vulgaris L.). Symbiosis, 46, 137–143. [Google Scholar]
17. Moreau, S., Meyer, J. M., Puppo, A. (1995). Uptake of iron by symbiosomes and bacteroids from soybean nodules. FEBS Letters, 361, 225–228. [Google Scholar] [PubMed]
18. Brear, E. M., Day, D. A., Smith, P. M. C. (2013). Iron: An essential micronutrient for the legume-rhizobium symbiosis. Frontiers Plant Science, 4, 359. https://doi.org/10.3389/fpls.2013.00359 [Google Scholar] [PubMed] [CrossRef]
19. Caro, A., Puntarulo, S. (1996). Effects of in vivo iron supplementation on oxygen radical production by soybean roots. Biochimica and Biophysyca Acta, 1291, 245–251. https://doi.org/10.1016/S0304-4165(96)00071-2 [Google Scholar] [PubMed] [CrossRef]
20. Tang, C., Robson, A. D., Dilworth, M. J. (1990). The role of iron in nodulation and nitrogen fixation in Lupinus angustifolius L. New Phytologyst, 114(2), 173–182. [Google Scholar]
21. Grillet, L., Ouerdane, L., Flis, P., Hoang, M. T., Isaure, M. P. et al. (2014). Ascorbate efflux as a new strategy for iron reduction and transport in plants. Journal of Biological Chemistry, 289, 2515–2525. https://doi.org/10.1074/jbc.M113.514828 [Google Scholar] [PubMed] [CrossRef]
22. Tang, C., Robson, A. D., Dilworth, M. J. (1991). Which stage of nodule initiation in Lupinus angustifolius L. is sensitive to iron deficiency? New Phytologyst, 117, 243–250. [Google Scholar]
23. Wei, Y., Shohag, M. J. I., Yang, X., Zhang, Y. (2012). Effects of foliar iron application on iron concentration in polished rice grain and its bioavailability. Journal of Agricultural and Food Chemistry, 60, 11433–11439. [Google Scholar] [PubMed]
24. Caliskan, S., Ozkaya, I., Caliskan, M. E., Arslan, M. (2008). The effects of nitrogen and ironfertilization on growth, yield and fertilizer use efficiency of soybean in a Mediterranean-type soil. Field Crops Research, 108, 126–132. [Google Scholar]
25. Krouma, A. (2023). Potential of animal manure amendments in combating calcareous induced iron deficiency in pearl millet. Plant Stress, 7, 100139. https://doi.org/10.1016/j.stress.2023.100139 [Google Scholar] [CrossRef]
26. Salhi, K., Hajlaoui, H., Krouma, A. (2022). Genotypic differences in response of durum wheat (Triticum durum Desf.) to lime-induced iron chlorosis. Plant Direct, 6, e377. https://doi.org/10.1002/pld3.377 [Google Scholar] [PubMed] [CrossRef]
27. Krouma, A., Abdelly, C. (2003). Importance of iron use efficiency in common bean (Phaseolus vulgaris L.) for iron chlorosis resistance. Journal of Plant Nutrition and Soil Science, 4, 525–528. [Google Scholar]
28. Drevon, J. J., Kalia, V. C., Pedelahore, P. (1988). In situ open-flow assay of acetylene reductionactivity by soybean root nodules: Influence of acetylene and oxygen. Plant Physiology and Biochemistry, 26, 73–78. [Google Scholar]
29. Bouslimi, H., Ferreira, R., Dridi, N., Brito, P., Martins-Dias, S. et al. (2021). Effects of barium stress in Brassica juncea and Cakile maritima: The indicator role of some antioxidant enzymes and secondary metabolites. Phyton-International Journal of Experimental Botany, 90(1), 145–158. https://doi.org/10.32604/phyton.2020.011752 [Google Scholar] [CrossRef]
30. Sghaier, D. B., Bankaji, I., Pedro, S., Caçador, I., Sleimi, N. (2019). Photosynthetic behaviour and mineral nutrition of Tamarix gallica cultivated under Aluminum and NaCl combined stress. Phyton-International Journal of Experimental Botany, 88(3), 239–252. https://doi.org/10.32604/phyton.2019.06887 [Google Scholar] [CrossRef]
31. Krouma, A., Benhamed, K., Abdelly, C. (2008). Symbiotic response of common bean (Phaseolus vulgaris L.) to iron deficiency. Acta Physiologiae Plantarum, 30, 27–34. [Google Scholar]
32. Koeseoglu, A. T., Acikgoz, V. (1995). Determination of iron chlorosis with extractable iron analysis in peach leaves. Journal of Plant Nutrition, 18, 153–161. [Google Scholar]
33. Barhoumi, S., Ellouzi, H., Krouma, A. (2023). Functional analysis of the genotypic differences in response of Pea (Pisum sativum L.) to calcareous-induced iron deficiency. Phyton-Internatonal Journal of Experimental Bototany, 92(2), 521–536. https://doi.org/10.32604/phyton.2022.023555 [Google Scholar] [CrossRef]
34. Ksouri, R., Debez, A., Mahmoudi, H., Ouerghi, Z., Gharsalli, M. et al. (2007). Genotypic variability within Tunisian grapevine varieties (Vitis vinifera L.) facing bicarbonate-induced iron deficiency. Plant Physiology and Biochemistry, 45, 315–322. [Google Scholar] [PubMed]
35. Bertamini, M., Muthuchelian, K., Nedunchezhian, N. (2002). Iron deficiency induced changes on the donor side of PS II in field grown grapevine (Vitis vinifera L. cv. Pinot noir) leaves. Plant Science, 162, 599–605. [Google Scholar]
36. Jiang, C., Gao, H., Zou, Q., Shi, L. (2007). Effects of iron deficiency on photosynthesis and photosystem II function in soybean leaf. Journal of Plant Physiology and Molecular Biology, 33, 53–60. [Google Scholar] [PubMed]
37. Kabir, A. H., Paltridge, N. G., Roessner, U., Stangoulis, J. C. (2013). Mechanisms associated with Fe-deficiency tolerance and signaling in shoots of Pisum sativum. Physiologia Plantarum, 147, 381–395. [Google Scholar] [PubMed]
38. Wang, M. Y., Xia, R. X., Hu, L. M., Dong, T., Wu, Q. S. (2007). Arbuscular mycorrhizal fungi alleviate iron deficient chlorosis in Poncirus trifoliata L. Raf under calcium bicarbonate stress. Journal of Horticultural Science and Biotechnology, 82, 776–780. [Google Scholar]
39. Ferhi, J., Gharsalli, M., Abdelly, C., Krouma, A. (2017). Potential of the physiological response of Pea plants (Pisum sativum L.) to iron deficiency (direct or lime-induced). Bioscience Journal, 33(5), 1208–1218. [Google Scholar]
40. Valentinuzzi, F., Pii, Y., Carlo, P., Roberto, T., Fontanella, M. C. et al. (2020). Root-shoot-root Fe translocation in cucumber plants grown in a heterogeneous Fe provision. Plant Science, 293, 110431. https://doi.org/10.1016/j.plantsci.2020.110431 [Google Scholar] [PubMed] [CrossRef]
41. Chikoti, Y. F., Duangkhet, S. M., Chungopast, S., Tajima, S., Ma, J. F. et al. (2020). Effect of ferritin on nitrogen fixation in Lotus japonicus nodules under various iron concentrations. Journal of Plant Physiology, 252, 153247. https://doi.org/10.1016/j.jplph.2020.153247 [Google Scholar] [PubMed] [CrossRef]
42. Rai, R., Prasad, V., Choudhury, S. K., Sinha, N. P. (1984). Iron nutrition and symbiotic N2 fixation of lentil (lens culinaris) genotypes in calcareous soil. Journal of Plant Nutrition, 7(1–5), 399–405. [Google Scholar]
43. O’Hara, G. W., Boonkerd, N., Dilworth, M. J. (1988). Mineral constraints to nitrogen fixation. Plant and Soil, 108, 93–110. [Google Scholar]
44. Guerinot, M. L. (1991). Iron uptake and metabolism in the rhizobia/legume symbioses. Plant and Soil, 130, 199–209. [Google Scholar]
45. Gonzalez-Guerrero, M., Escudero, V., Saez, A., Tejada-Jimenez, M. (2016). Transition metal transport in plants and associated endosymbionts: Arbuscular mycorrhizal fungi and rhizobia. Frontiers in Plant Science, 7, 1088. https://doi.org/10.3389/fpls.2016.01088 [Google Scholar] [PubMed] [CrossRef]
46. Clarke, V. C., Loughlin, P. C., Day, D. A., Smith, P. M. C. (2014). Transport processes of the legume symbiosome membrane. Frontiers in Plant Science, 5. https://doi.org/10.3389/fpls.2014.00699 [Google Scholar] [PubMed] [CrossRef]
Cite This Article
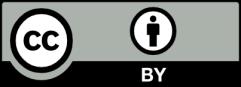
This work is licensed under a Creative Commons Attribution 4.0 International License , which permits unrestricted use, distribution, and reproduction in any medium, provided the original work is properly cited.