Open Access
ARTICLE
Lipid Production, Oxidative Status, Antioxidant Enzymes and Photosynthetic Efficiency of Coccomyxa chodatii SAG 216-2 in Response to Calcium Oxide Nanoparticles
1
Department of Botany & Microbiology, Faculty of Science, Assiut University, Assiut, 71516, Egypt
2
Department of Genetics and Plant Breeding, Bangladesh Agricultural University, Mymensingh, 2202, Bangladesh
* Corresponding Authors: Mohammad Anwar Hossain. Email: ; Mona F. A. Dawood. Email:
(This article belongs to the Special Issue: Selenium, Silicon and their Nanoparticles-mediated Environmental Stress Tolerance in Crop Plants)
Phyton-International Journal of Experimental Botany 2023, 92(7), 1955-1974. https://doi.org/10.32604/phyton.2023.028583
Received 26 December 2022; Accepted 09 March 2023; Issue published 29 May 2023
Abstract
The extensive use of nanoparticles (NPs) in diverse applications causes their localization to aquatic habitats, affecting the metabolic products of primary producers in aquatic ecosystems, such as algae. Synthesized calcium oxide nanoparticles (CaO NPs) are of the scarcely studied NPs. Thus, the current work proposed that the exposure to CaO NPs may instigate metabolic pathway to be higher than that of normally growing algae, and positively stimulate algal biomass. In this respect, this research was undertaken to study the exposure effect of CaO NPs (0, 20, 40, 60, 80, and 100 µg mL−1 ) on the growth, photosynthesis, respiration, oxidative stress, antioxidants, and lipid production of the microalga Coccomyxa chodatii SAG 216-2. The results showed that the algal growth concomitant with chlorophyll content, photosynthesis, and calcium content increased in response to CaO NPs. The contents of biomolecules such as proteins, amino acids, and carbohydrates were also promoted by CaO NPs with variant degrees. Furthermore, lipid production was enhanced by the applied nanoparticles. CaO NPs induced the accumulation of hydrogen peroxide, while lipid peroxidation was reduced, revealing no oxidative behavior of the applied nanoparticles on alga. Also, CaO NPs have a triggering effect on the antioxidant enzymes such as superoxide dismutase, catalase, ascorbate peroxidase, and guaiacol peroxidase. The results recommended the importance of the level of 60 µg mL−1 CaO NPs on lipid production (with increasing percentage of 65% compared to control) and the highest dry matter acquisition of C. chodatii. This study recommended the feasibility of an integrated treatment strategy of CaO NPs in augmenting biomass, metabolic up-regulations, and lipid accumulation in C. chodatii.Keywords
The wide use of the synthesized nanomaterials makes their final destination into the aquatic environment especially the world production of nanoparticles is rapidly increased. In this regard, when the world production of synthesized nanomaterials in 2010 was 309000 tons, about 0.4%–7% of the nano-materials were discharged into aquatic habitats [1]. Some of the NPs applied for groundwater and other water bodies treatments find also their way to aquatic system [2] affecting the aquatic organisms such as algae. Since algae are primary source of biomass production that supports all higher trophic levels of the aquatic body [3], have low nutritional requirements, and have quick examination of the entire life cycle [4], they are considered to be ideal model organism for assessing the impact of these NPs [5]. Therefore, intensive emphasizing of the biological effects of the synthesized NPs on algae and the related-mechanisms is of pivotal interest.
Microalgae are light-powered cell factories that produce various bioproducts of high trading significance, such as sugars, proteins, lipids, vitamins, and pigments [6]. Also, microalgae are adaptable organisms with high physiological plasticity; as a result, biomolecules production can vary depending on environmental conditions [7]. Because of their propensity to accumulate considerable amounts of lipids, most microalgae have emerged as attractive biofuel resources. Barreto et al. [8] reported that the physiology of microalgae has already been affected by nanoparticles especially the lipid production. Various studies included the biological impacts of metal oxide nanoparticles or organic ones on various aspects of algae. However, the use of metal oxide NPs is impressively increased. It has been reported that metal oxide NPs would steadily liberate the metal ion, hence affecting algal biology [9–11]. It has been reported that metal oxide NPs show biphasic nature as negative effect in the form of heavy metals and toxic agents or positive effect on the growth component or specific metabolic products. In the present study, we will shed light on the significance of one of the macronutrient NPs on microalga, CaO NPs.
Calcium (Ca) is an essential macronutrient for algae and plants. It is ubiquitous in all subcellular structures, cell walls, membranes, cytosol, nuclei, chloroplasts, mitochondria, etc. [12]. It is also ubiquitous in functions; it regulates an impressive and diverse number of physiological processes, including cell division, photosynthesis, respiration, nitrogen metabolism, enzymes, protein synthesis and phosphorylation, cell division, elongation and mobility, membrane stability, structural and physical properties of the cell wall [12,13]. Thus, transforming Ca to CaO NPs could increase its delivery inside the cell; hence increase its effectiveness on algae. CaO NPs are distinguishable nanomaterials due to their low toxicity and unique physicochemical characteristics [14] such as having good basicity, cost-effective, non-corrosive, being environmentally friendly manageable [15]. Besides, it can be synthesized in in short duration and easy to be recycled [16]. All these favorable characteristics facilitate its use in various applications such as drug delivery, synaptic delivery of chemotherapeutic agents, and photodynamic therapy [17]. In addition, CaO NPs distinguished with controlled size, infiltration into cell and tissue, prohibited aggregation by coating, precise contact, and easy dispersion which give it priority relative to other metal nanoparticles. Recent studies used CaO NPs in agricultural studies where growth enhancement of different plant species by CaO NPs was reported [18,19], but the role of CaO NPs to improve algal growth and metabolism is still elusive.
Interestingly, the researches concerning the effect of CaO NPs on algae have been rarely studied. To our knowledge, little is known about the regulatory effects of CaO NPs on Coccomyxa. Coccomyxa species, are fresh water microalga belongs to chlorophyta-Trebouxiophyceae. It lives freely in terrestrial ecosystem, floating in freshwater habitats, and as lichen photobionts, even present as impurity in the water used for cooling the nuclear power plant, and occurred in the chemical solutions and distilled water of laboratories [20]. It becomes suitable model for the determination of algal biochemical features because of their high growth rate, and the ability to produce high quality lipids [21]. Thus, the physiological effects of CaO NPs on C. chodatii should be studied in light of their major importance. The current work proposed that the exposure to CaO NPs may instigate metabolic pathway higher than that of normally growing algae. In this regard, it is crucial to understand the influential effect of CaO NPs on the algal biomass. Furthermore, the interaction between algal cells and the CaO NPs can affect metabolic pathways especially lipids as a major component of Coccomyxa and induce antioxidant properties. It has been assumed that the interaction of NPs and microalgae may instigate the pathways related to bioactive substances, so the mechanistic effect of these NPs could be cleared. Thus, it would increase the knowledge on emphasizing the environmental modulation and the interplay between NPs and algae, and match the prospective needs for sustainable nanotechnology evolution. Thus, the aim of the present research was to report the behavior of C. chodatii in response to different levels of CaO NPs via studying (i) algal growth and calcium accumulation, (ii) photosynthetic efficacy, (iii) primary metabolites accumulation, (iv) lipids accumulation, (v) oxidative damage and membrane integrity, and (vi) antioxidative responses.
2.1 Preparation and Characterization of CaO NPs
Calcium nitrate (Sigma–Aldrich) was used for the preparation of CaO NPs. Ca(NO3)2 was dehydrated in an oven at 150°C for 2 h and then ground using a SPEX8000 apparatus with a vial and balls of tungsten carbide and a ball-to-powder weight ratio (BPR) of 10:1 (Fritsch, Mini-mill-Pulverisette) [22]. The prepared CaO NPs were characterized by transmission electron microscopy, Fourier transform infrared spectroscopy (FTIR) and UV–Vis spectroscopy [23].
2.1.1 Transmission Electron Microscopy (TEM)
Chemically synthesized CaO NPs nanoparticles were screened by placing a droplet of the nanoparticles onto negative carbon-coated copper grids and drying them in the air. The TEM micrographs of the prepared NPs were analyzed with a JEOL TEM 100 CXII (Electron Microscope Unit, Assiut University, Egypt) where the size and shape of CaO NPs were investigated considering the method [22].
2.1.2 Fourier Transform Infrared Spectroscopy (FTIR)
FTIR spectroscopy was used to study the functional groups of the dried powder of CaO NPs. FTIR spectra over the scanning range of 4000–400 cm−1 were obtained with a resolution of 2 cm−1. The FTIR spectrum was obtained using the KBr pellet method [24] on a FTIR spectrophotometer (IR 470-Shimadzu-Japan).
The prepared CaO NPs nanoparticles were spectrally analyzed and scanned at wavelengths (200–800 nm) utilizing a double beam spectrophotometer (Perkin-Elmer lambda 750 spectrophotometer) as described by Yousef et al. [23].
2.2 Physiological Effects of CaO NPs on Coccomyxa chodatii SAG 216-2: Growth Conditions of Coccomyxa chodatii
C. chodatii SAG 216-2 was kindly offered by SAG (Güttingen University, Germany). Coccomyxa were grown under sterile conditions in modified BG11 medium [25]. The cultures were incubated at a temperature of 25°C ± 2°C, pH 7.5, and under continuous illumination with white fluorescent lamps (48.4 μmol m−2 s−1 and shaking at 130 rpm).
2.3 Experimental Design for the Effect of Calcium Oxide Nanoparticles (CaO NPs) on Algal Culture
The following calcium oxide nanoparticle treatments were applied to the algal cultures (zero time) at concentrations of 0 (control), 20, 40, 60, 80, and 100 μg mL−1 to evaluate and compare their effects on the growth, photosynthesis, respiration, antioxidants and lipid production. The cultures were grown under the aforementioned conditions and some traits were daily recorded for 6 days on fresh cultures such as optical density (OD) and chlorophyll (a+b). The cultures were conducted for 15 days and then harvested for the estimation of dry weight, lipids, sugars, protein, amino acids, oxidative damage, and antioxidants.
2.3.1 Determination of C. chodatii Growth and Calcium Content under Different Conditions
The absorbance of C. chodatii cultures was daily monitored at OD 750 nm throughout the experimental period (until the stationary phase after 6 days of exposure to different levels of CaO NPs) with a spectrophotometer (Unico UV-2100 spectrophotometer). The dry mass of C. chodatii was determined gravimetrically; 15 mL algal suspensions were filtrated using pre-weighed glass fiber filters. The filter papers along with algal cells were dried overnight in an oven at 80°C and reweighted; the dry mass was calculated as mg mL−1 algal suspension. Then the biomass productivity was estimated as the follow:
Algal biomass productivity (mg/DW) = algal biomass (mg)/days.
The content of calcium has been determined as described before by Biedermann and Schwarzenbach [26].
2.3.2 Estimation of Chlorophyll Content, Net Photosynthetic Efficiency, and Dark Respiration
Chlorophyll (a+b) was extracted by methanol according to Marker [27] and expressed in μg mL−1 algal suspension. The net photosynthetic oxygen evolution (PN) and dark respiratory oxygen uptake (RD) were monitored using Oxygraph plus, Hansatech Instruments Inc. (donation from the Alexander von Humboldt Foundation Germany to R. Abdel Basset). Two mL of algal culture was monitored for 15 min at 25°C with continuous red-light intensity of 100 mmol m−2 sec−1 and then PN and RD rates were calculated as mmol O2 mg Chl−1 h−1.
2.3.3 Estimation of Protein, Carbohydrate, and Amino Acid Contents
The contents of soluble proteins and amino acids were assessed using the methods explained by Lowry et al. [28] and Moore et al. [29], respectively using bovine serum albumin and glycine as standards, respectively. While sugar content was estimated using glucose as a standard following the method described by Fales [30] and Schlegel [31].
2.3.4 Extraction and Estimation of Total Lipids
The total lipids were extracted from algal cells by a mixture of chloroform: methanol (2:1 v/v) using the slightly modified version of Hara et al. [32]. Algal culture (70 mL) was centrifuged at 3000 rpm for 10 min. The supernatant was removed, and the algal biomass was suspended in 6 mL chloroform/methanol mixture, shaken well, incubated in the dark overnight; and then 5 mL MgCl2 was added and vortexed well. The chloroform–methanol formed a biphasic layer; the lower lipid layer was drawn off by a long syringe, transferred into a weighed aluminum foil cup, evaporated and reweighed. The weight of total lipids was determined gravimetrically, and the lipid contents were calculated as a percentage of dry mass.
2.3.5 Hydrogen Peroxide and Lipid Peroxidation
Hydrogen peroxide was determined based on the protocol of Yu et al. [33]. Lipid peroxidation was estimated by monitoring malondialdehyde (MDA) formation according to the method of Madhava Rao et al. [34] by using the thiobarbituric acid reaction.
For determination of the antioxidant enzymes, by combining prolonged freezing and sonication, 100 mL of algal culture was homogenized in 5 mL of 100 mM potassium phosphate buffer (pH 7.8) with 0.1 mM EDTA and 0.1 g polyvinyl pyrrolidone. The homogenate was centrifuged for 10 min at 5000 rpm at 4°C, and the supernatant was collected and used for superoxide dismutase (SOD), catalase (CAT), guaiacol peroxidase (POD), and ascorbate peroxidase (APX) assays. The enzyme activity was expressed as µmol−1 mg protein min−1. SOD activity was measured following [35] in a medium containing sodium carbonate buffer (pH 10.2), EDTA, enzyme extract, and epinephrine. The change in absorbance was monitored at 480 nm. CAT activity was evaluated by following the consumption of H2O2 for 1 min according to Aebi [36] and Matsumura et al. [37]. POD activity was measured spectrophotometrically according to the method of Tatiana et al. [38]. APX activity was calculated by observing the oxidation of ascorbate at 290 nm in the presence of H2O2 [39].
Experiments were presented in three replicates. The data were calculated as the means ± standard errors (n = 3). One-way ANOVA was used to analyze most data except the optical density and chlorophyll were subjected to two-way ANOVA. Duncan’s multiple range tests were used to compare the means. The significance of the data was determined at 5% level (p < 0.05).
3.1 Characterization of CaO NPs
3.1.1 Transmission Electron Microscopy (TEM)
TEM images of the synthesized CaO NPs are illustrated in Fig. 1A. CaO NPs are dispersed very well in the distilled water. The morphology of the prepared samples indicates that the diameter of the synthesized CaO NPs were less than 100 nm, which confirms the nanoscale of the obtained sample. The diameter of CaO NPs ranged from 15.5 to 41.7 nm and they are spherical in shape.
Figure 1: Characterization of the synthesized CaO NPs as presented by (A) TEM imaging, (B) FTIR, and (C) the absorbance spectra from 200–700 nm
3.1.2 Fourier Transform Infrared Spectroscopy (FTIR)
To verify the chemical purity of the prepared CaO NPs, FTIR spectroscopy was used. The FTIR spectrum clearly shows strong bands due to hydroxyl and carbonate groups. The C-O bond is represented by the bands at 1417 and 866 cm−1. Ca-O bonds are represented by wide and strong bands at 427 and 533 cm−1 (Fig. 1B).
Studying the absorption spectra is considered the simplest and most direct method for investigating the band structure of the prepared CaO NPs. Fig. 1C shows the absorbance of CaO NPs as a function of the wavelength. It is obvious that the prepared CaO NPs interact with the incident light and declare a maximum absorption at 203 nm which confirms the nanoscale size of the prepared samples. Additionally, the absorption is decreased by increasing the wavelength of the incident light.
3.2 Growth and Physiological Responses of C. chodatii to Different Levels of CaO NPs
3.2.1 Growth and Chlorophyll Content
Fig. 2 represents the traits used for estimating the growth of C. chodatii under different concentrations of CaO NPs (0, 20, 40, 60, 80, and 100 µg mL−1) for 6 days (the end of the logarithmic phase). In this regard, the dry mass of Coccomyxa and biomass productivity recorded on the sixth day of exposure to CaO NPs, significantly enhanced as the concentration of CaO NPs was increased reaching a maximal value at 60 µg mL−1 CaO NPs. However, at the higher levels, the dry matter and biomass productivity were lower than that at 60 µg mL−1 CaO NPs recorded significant increment of dry mass by 23% and 19% relative to control at the levels of 80 and 100 µg mL−1 CaO NPs, respectively (Fig. 2A). The OD of algal cultures recorded at 750 nm was followed daily until reaching the stationary phase; the algal culture grown at 60 and 100 µg mL−1 CaO NPs exhibited the highest OD until the 4th day. However, on the 6th day of CaO NPs exposure, the optical density of 60 µg mL−1 CaO NPs recorded the highest value followed by 100 µg mL−1 CaO NPs compared to control (Fig. 2B).
Figure 2: The growth of Coccomyxa chodatii in response to different levels CaO NPs in terms of (A) dry mass (mg mL−1 culture) and biomass productivity (mg day−1), (B) the daily record of optical density (OD), (C) the daily record of chlorophyll (a+b), (D) linear regression between OD and biomass, and (E) calcium content (µg mL−1 culture) of algal cultures grown under different levels of CaO NPs (0, 20, 40, 60, 80, and 100 µg mL−1) for 6 days. Each histogram represents the mean of three biological replicates ± SE. The different letters indicated significantly different at p < 0.05. For the optical density and total chlorophyll, the capital letters indicated the effect of different treatments within each sampling time and lower case letters indicated the effect of the different sampling dates within each treatment
The Chl (a+b) content of the algal cells under various CaO NPs concentrations (during the first six days of the NPs exposure) was shown in Fig. 2C. The chlorophyll content increased significantly with intensifying the level of CaO NPs, recording the highest value at 100 µg mL−1 CaO NPs on the first four days of exposure. Interestingly, on the 6th day, the level of 60 µg mL−1 CaO NPs exhibited the highest chlorophyll content (Fig. 2C) similar to the results recorded for OD (Fig. 2B). Furthermore, using the linear regression between OD and biomass recorded in Fig. 2D recommended the fitting between dry weight and biomass where R2 = 0.9043.
The calcium content of algal cultures represented in Fig. 2E showed highly significant accumulation of Ca as the concentration of nanoparticles increased in the medium. It is obvious that the highest levels 80 and 100 µg mL−1 CaO NPs have the highest content of Ca and the lowest was for the control plants.
3.2.2 Photosynthetic Oxygen Evolution (PN), Dark Respiratory Oxygen Uptake (RD), and PN/RD
The net photosynthetic oxygen evolution (PN) decreased significantly compared to zero CaO NPs-received cultures at the levels 20 and 40 µg mL−1 CaO NPs. By increasing the level of CaO NPs in the algal culture media, the PN promoted highly significantly relative to the control, recording the highest value at 80 µg mL−1 CaO NPs (Fig. 3).
Figure 3: Net photosynthetic activity (PN), dark respiration (RD), and PN/RD of Coccomyxa chodatii grown at various levels of CaO NPs (0, 20, 40, 60, 80, and 100 µg mL−1). Different letters of columns or lines for the same series are revealing significant differences at p < 0.05
Regarding to the respiratory oxygen uptake (RD) of C. chodatii grown under different levels of CaO NPs, a positive significant effect was denoted for most levels except for 60 µg mL−1 CaO NPs compared to the control. The highest RD was recorded for the level of 40 µg mL−1 CaO NPs. But, the level of 60 µg mL−1 CaO NPs decreased the RD level relative to 0 µg mL−1 CaO NPs–received cultures. Furthermore, the ratio of PN/RD exhibited various responses to CaO NPs application compared to control, a decrement of PN/RD was recorded at the levels of 20 and 40 µg mL−1 CaO NPs, non-significant change of this ratio for 80 and 100 µg mL−1 CaO NPs, while highly significant increase of PN/RD at 60 µg mL−1 CaO NPs was found in the present work (Fig. 3).
Proteins and amino acids: Figs. 4A and 4B present the soluble protein and amino acid contents of C. chodatii treated with different concentrations of CaO NPs where unsteady increasing patterns were recorded compared to the control. In Fig. 4A, the highest protein values were recorded at the levels 20 and 80 µg mL−1 CaO NPs compared to control, while 60 µg mL−1 CaO NPs stabilized protein content around the control. Fig. 4B shows that the amino acids content was significantly increased than the control via CaO NPs treatments. However, the level of 60 µg mL−1 CaO NPs induced the highest accumulation of amino acids content and the lowest were denoted for 100 µg mL−1 CaO NPs compared to control.
Figure 4: Proteins (A), amino acids (B), sugars (C), and lipids (D) contents of Coccomyxa chodatii grown at various levels of CaO NPs (0, 20, 40, 60, 80, and 100 µg mL−1). Different letters of columns are revealing significant differences at p < 0.05
Sugar content: The sugar contents data clearly demonstrated significant accumulation trend in response to the application of CaO NPs, and the highest accumulation was recorded at the level of 60 µg mL−1 CaO NPs with increasing percentage of 107% compared to control (Fig. 4C). Notwithstanding, the accumulation of sugars for the rest CaO NPs levels exhibited increasing percentages of 48%, 80%, 80%, and 66% for the levels 20, 40, 80, and 100 µg mL−1 CaO NPs, respectively compared to the control.
Lipid production: Fig. 4D showed the lipid accumulation production of C. chodatii with different concentrations of CaO NPs (20, 60, 80, and 100 µg mL−1), while 40 µg mL−1 exhibited lowest values than the control. The optimal concentration of CaO NPs for lipid productivity was 60 µg mL−1 with increasing percentage of 65% compared to control. The highest levels of CaO NPs also improved the lipid productivity, but with fewer efficacies recording 38% and 23% increasing trend for the levels of 80 and 100 µg mL−1 CaO NPs, respectively compared to control.
3.2.4 Hydrogen Peroxide and Lipid Peroxidation
Hydrogen peroxide (H2O2) content recorded in Fig. 5A, found to be statistically increased regardless the level of CaO NPs applied with a significant increase at 20, 40, 60, 80, and 100 µg mL−1 CaO NPs with average percent increase around 21% relative to control. On the other hand, the sign of oxidative damage of increasing the reactive oxygen species in terms of lipid peroxidation was non-significantly changed for the level of 100 µg mL−1 CaO NPs. While lipid peroxidation reduced significantly for the rest levels recording highly significant decrements at 40 and 60 µg mL−1 CaO NPs (Fig. 5B) with reduction percentages of 33% and 41%, respectively compared to control, while 20 and 80 exhibited less reduction percentages.
Figure 5: Hydrogen peroxide (A) and lipid peroxidation (B) of Coccomyxa chodatii grown for 6 days at various levels of CaO NPs (0, 20, 40, 60, 80, and 100 µg mL−1). Different letters are revealing significant differences at p < 0.05
Catalase: Fig. 6A showed the catalase (CAT) activity of C. chodatii at different concentrations of CaO NPs. CAT activity increased whatever the level used compared with the control. The highest activity was recorded at 40 µg mL−1 CaO NPs with increasing percentage of 71%, followed by the levels of 100 and 80 µg mL−1 CaO NPs recording increasing percentage of 60% and 26%, respectively compared to control. The levels of 20 and 60 µg mL−1 CaO NPs showed the lowest increment compared to the other CaO NPs levels.
Figure 6: The activities of catalase (A), guaiacol peroxidase (B), ascorbate peroxidase (C), and superoxide dismutase (D) of Coccomyxa chodatii grown at various levels of CaO NPs (0, 20, 40, 60, 80, and 100 µg mL−1). Different letters are revealing significant differences at p < 0.05
Guaiacol peroxidase: Guaiacol peroxidase (POD) activity presented in Fig. 6B unsteadily increased whatever the level of CaO NPs used, with highly raising values at 20 and 80 µg mL−1 CaO NPs (by about 84% compared to control), followed by the levels 60 and 100 µg mL−1 CaO NPs (with increasing percentage of 69% and 52%, respectively compared to control). However, the level of 40 µg mL−1 CaO NPs exhibited non-significant change of POD activity compared to 0 µg mL−1 CaO NPs.
Ascorbate peroxidase: Ascorbate peroxidase (APX) activity denoted irregular significant enhancement in response to the addition of CaO NPs (Fig. 6C). In this regard, the highest activities were recorded for the levels 40 and 60 µg mL−1 with increasing percentages of 51% and 64%, respectively compared to the control. On the other hand, the levels 20, 80, and 100 µg mL−1 exhibited lesser APX activity, but still higher than the control.
Superoxide dismutase: The activity of superoxide dismutase (SOD) was found to be regularly enhanced with the application of CaO NPs with a paramount elevation level at 60 µg mL−1 CaO NPs with enhancing value of 72% over that of control (Fig. 6D). Afterwards, the increase was reduced to be comparable to that of the level 20 µg mL−1 CaO NPs.
4.1 Characterization of the Synthesized CaO NPs
The synthesized nanoscale CaO NPs was found to be spherical with diameters ranging from 15.5–41.7 nm. The purity of the prepared CaO NPs was confirmed by FTIR spectroscopy, where obvious bands of O-H, C-O, and Ca-O bonds were detected. In this respect, Park et al. [40] reported that the strong band at 3634 cm−1 corresponds to the O-H bonds from the remaining hydroxide. Furthermore, UV–Vis spectroscopy recommended the nanometery preparation of CaO NPs, where the maximum absorption of the synthesized material was recorded at 238 nm as was reported by Yousef et al. [23].
4.2 Physiological Responses of C. chodatii to Different Levels of CaO NPs
With the continued implementation of nanoparticles in various fields during current and future life, the trials to determine the positive impacts of nanoparticles on model organisms such as algae are of crucial importance. Microalgae cultures are currently the subject of intensive research aimed at enhancing the synthesis and optimizing the production of useful compounds. It has been reported that metal oxide NPs would steadily liberate the metal ion, hence affecting algal biology [10,11]. Thus, we assumed in the present investigation that CaO NPs when dissolve in the medium, their constituent material will be liberated, which could have a phyco-impact depending on the concentration applied. In conformity, the exposed algal cells’ to CaO NPs exhibited high content of cellular calcium compared to the control. Such accumulation of Ca on C. chodatii could be linked to various growth and regulatory mechanisms induced by CaO NPs. Calcium plays a major role in the plant development such as increasing chlorophyll synthesis, signal transduction, pathogenic resistance, and stress tolerance [41]. In this regard, the use of CaO NPs has a positive effect on increasing the growth parameters of C. chodatii in terms of optical density, growth productivity, and dry mass acquisition in a concentration-dependent manner. Interestingly, the linear regression between dry and optical density of algal cultures revealed their compatible fitting under different CaO NPs concentrations. Calcium was also reported to be crucial for algal growth, as Cholrella sp. [42]. Positive effects of increasing calcium were reported for Cholrella vulgaris and S. obliquus in the study of Gorain et al. [43]. In addition, Nguemezi et al. [41] noted that TiO2-NPs enhanced algal growth. Also, Hartmann et al. [44] found that titanium oxide NPs promote the growth of Pseudokirchneriella subcapitata at low concentrations. On the other hand, the phycotoxic impacts of nanoparticles were recorded in other studies [5,8,45,46].
Algae change their metabolism to adjust their biochemical composition and photosynthetic pigments depending on their growing conditions. To shed light on the metabolic changes occurring inside C. chodatii, the cells were analyzed for biomolecules such as carbohydrates, amino acids, proteins, lipids, and photosynthetic pigment contents. The chlorophyll content is an indicator of the growth situation of the algal cells; the application of CaO NPs enhanced the chlorophyll content of C. chodatii parallel to growth improvements and increased intracellular Ca content. Similarly, Middepogu et al. [47] reported that the content of photosynthetic pigments of Chlorella pyrenoidosa exposed to various concentrations of TiO2 nanoparticles concurrently increased. This matched the results obtained with Chlamydomonas reinhardtii treated with copper oxide nanoparticles [48]. Also, Husseini et al. [49] reported that CaCl2 enhanced the chlorophyll a, b, and carotenoids of C. vulgaris under gamma irradiation. The accumulation of pigment by NPs may be attributed to NP-induction of ROS can target certain pigments, causing them to be changed to chlorophyll a [50]. The increases in the chlorophyll content help algae to produce more light harvesting complexes to receive higher levels of light energy, which stimulates photosynthesis and thereby the growth of algae. It has been reported that in photosynthetic systems, NPs can improve the efficiency of chemical energy production [51].
Consequently, the highest concentrations of CaO NPs instigated the photosynthetic oxygen evolution of Coccomyxa, in the present study, compared to that of the control. Several investigations stated the ability of NPs to promote the photosynthetic attributes of microalgae [52–54]. It has been reported that the positive effects of NPs on the photosynthetic performance might ascribed to their widened spectral region for energy capturing [55] and higher chlorophyll content [56]. In the present study, CaO NPs increased the contents of chlorophyll and calcium of Coccomyxa, such increment of Ca2+, may affects the enzymatic functions of Ca2+-binding proteins in the chloroplast, hence regulating the oxygen evolution of photosystem II (PSII), improving the photosynthetic electron transfer and photoprotection mechanisms which may increase of the photosynthetic oxygen evolution [57]. Wang et al. [58] stated that Ca2+ could prevent the photoinhibition of the PSII subunits’ reaction centers by up-regulation of ROS where PSII requires metal cluster containing calcium (e.g., Mn4CaO5 cluster) for water oxidation. As the calcium content of algal cultures is increased due to CaO NPs exposure in the present work, the enhancement of chlorophyll and photosynthetic oxygen evolution could be recommended by the aforementioned interpretations.
The respiratory oxygen uptake, herein, recorded higher values in all cultures grown in CaO NPs concentrations than the control, except for the level of 60 µg mL−1 CaO NPs which was lower than that in the control. However, the mechanism of the different levels of CaO NPs showed concentration dependent responses with relation to net photosynthetic activity. The concentration 20 and 40 µg mL−1 exhibited high dark respiration, and low net photosynthetic activity compared to control. In this case, dark respiration is needed for maintaining the growth of cells, because it produces reducing substrates (e.g., NADPH and NADH), most of the usable energy (ATP), and carbon skeleton from organic components [59]. The inhibition of dark respiration to a lesser degree for the level 60 µg mL−1 than the other CaO NPs with high level of net photosynthetic capacity might be presumably attributed to a higher demand for energy and carbon skeletons which could be compensated by high net photosynthetic activity, thus PN/RD was higher than the control. The physiological interpretation of the inhibition of dark respiration may be ascribed to the presence of metabolites from photosynthesis-related metabolites (such as ATP and NADPH), working on respiratory enzymes as respiratory regulators as well as reducing the need for respiratory energy [60]. On the other hand, the higher levels of CaO NPS; 80 and 100 µg mL−1 enhanced both the net photosynthetic activity and dark respiration where balance in PN/RD compared to control was observed. It has been reported that calcium has crucial influence on the activity of various enzymes- and complexes-related to respiration. Also, it has been reported that the enhancement of calcium content of the cytoplasm, can improve calcium content in the mitochondrial matrix, hence it seems possibly that Ca2+ can be associated to the dark respiration response [61].
With regard to the metabolic products in response to CaO NPs, sugars were accumulated considerably by CaO NPs and the level of 60 µg mL−1 CaO NPs exhibited the highest soluble sugars compared to the other doses. This went in parallel with high net photosynthetic activity and chlorophyll content. Such accumulation of sugars could reveal increasing need for the carbohydrate use for energy production [61]. The accumulation of sugars could be adaptive response to NPs application where acting as osmotic molecules to increase absorbency and up-regulate water metabolism in algal species [62]. Others reported the importance of sugar accumulation as storage molecules to protect algal cells [63,64]. Marchello et al. [63] found that exposing C. sorokiniana to TiO2 NPs doubled carbohydrate values compared to the control, and a similar increase in sugars was reported also by Huang et al. [64] when Skeletonema costatum exposed to Ag NPs. Another role of sugar accumulation as signaling molecules, initiating the production of specific ROS scavengers, or acting as direct ROS scavengers [65]. Also, Mohamed [66] demonstrated that the water-soluble forms of sugars play role in scavenging ROS and free radicals in microalgal cells subjected to various stresses.
The first response to any change in the environment can be monitored by the alteration of lipid content, which affects the physiological state of cells [67]. The exposure of Coccomyxa to different levels of CaO NPs has a profound effect on increasing lipid content. These modifications could be one of the algae’s adaptive or defensive mechanisms in response to CaO NPs, as reported for the S. consortium following AgNPs treatment [64]. The high accumulation of lipids may be ascribed to two mechanisms; (i) altering of organic components of the cell (such as proteins and carbohydrate) to lipid [68], and (ii) utilization of inorganic carbon to de novo production of lipids [69]. In this respect, calcium is a prevalent secondary messenger, act as a signal transduction to environmental stimuli, the enhanced level of cytosolic Ca2+ up-regulate the neutral lipid production in green algae [70]. Gorain et al. [43] reported accumulation of lipids content in C. vulgaris under increased Ca2+ level. Also, as the concentration of Ca2+ intensified in C. vulgaris media, lipid production was enhanced under salinity conditions, aiding the microalga in resisting stressful environments [43,71]. Intriguingly, at the level of 60 µg L−1 CaO NPs; the highest level of lipids was accompanied with high carbohydrates, high efficiency of photosynthetic activity, and PN/RD, without change of proteins compared to control revealing that at this level, the highest carbon to nitrogen ratio could drive Coccomyxa metabolism towards lipid production. In this sense, the increase in the C level induced gene expression related to glycolysis and the Calvin cycle to improve the precursor of lipid production [72]. These lipids are precursors for phospholipids which are essential components for the membranous organelles and the endoplasmic reticulum [73], thus high efficient cells. A similar rise in cellular carbohydrate and total lipid content was reported for Chlorella vulgaris cultivated in sweet sorghum bagasse hydrolysate [74].
Importantly, CaO NPs enhanced the accumulation of lipid and carbohydrate from C. Chodatii without compromising biomass productivity. Thus, the production of lipids was not at the expense of dry matter acquisition, which is a beneficial mechanism in biofuel production. The effects of NPs induce an increase in the total biomass, accumulation of carbohydrate, and lipid content has been reviewed by Dev Sarkar et al. [75]. In other works, the application of nanoparticles and stressful environments could change the metabolism of many algal species for lipid biosynthesis [76–78]. In the present study, at the level of 60 CaO NPs, algal growth, carbohydrates, and lipids has been increased approximately by 30%, 107%, and 65%, respectively compared to control. To our knowledge, the effect of CaO NPs on algae and lipids production has not studied before especially on Coccomyxa or other algae and the recorded percentage of lipids at the optimal level was relatively high compared to other nanoparticles. In this respect, Kadar et al. [79] stated that adding Fe NPs to Tetraselmis suecica boosted the lipids content by 41.9%. He et al. [76] reported the enhancement of lipids by 18.5% and 39.6% due to the exposure of Scenedesmus obliquus to MgO NPs and Fe2O3 NPs, respectively. Rana et al. [80] reported that using Fe2O3 nanoparticles increased the growth and lipids of Chlorella pyrenoidosa by 33.75% and 15.2%, respectively. Recently, Hasnain et al. [81] recorded that lipid content highly significantly accumulated in Oedogonium sp., Ulothrix sp., Cladophora sp., and Spirogyra sp. by 60%, 56%, 58%, and 63%, respectively when exposed to AgNPs. Comparatively, using CaO NPs is a powerful tool increase the growth and lipids contents as a basic study for the production of biofuel using a save and ecofriendly nanoparticles. It is worth mentioning that increasing the lipids level, without affecting the algal growth rate or more likely increasing both as in the present study, takes the advent of obtaining the economic viability of algae-based biofuel production [64].
The protein pool of Coccomyxa under the various levels of CaO NPs was improved compared to the control except for the level 60 µg mL−1. The accumulation of proteins content is a general phenomenon of suitable conditions for algal growth and may be attributable to de novo synthesis of protein production by high availability of amino acids. In this regard, the CaO NPs application increased the amino acid content, which is a precursor of proteins and is probably a sign of stimulated carbohydrate metabolism. Such enhancement of protein content in response to CaO NPs could induce stimulation of well-structured photosynthetic proteins and enzymes related to chlorophyll biosynthesis. Also, the accumulation of proteins stimulates protein derivatives such as antioxidant enzymes and molecular chaperons, which are important agents in defensive mechanisms [76] to protect algal cells from abiotic stress [82]. Furthermore, the enhancement of protein could be linked to the synthesis of heat shock proteins [83] and the stimulation of mitochondrial enzymes, such as alternative oxidase and uncoupling proteins [84], which prevent ROS production. Similar increase in protein content was recorded for Nannochloropsis oculata, which was enhanced after exposure to ZnO- and Fe2O3-NPs [6]. A study by He et al. [76] recommended the accumulation of proteins in the cells of Scenedesmus obliquus exposed to Fe2O3-NPs. Thus, the stimulation of proteins, amino acids, thereby chlorophyll are probably crucial reasons for the improved C. chodatii growth under CaO NPs. Thus, CaO NPs stimulated the growth and metabolism of the intracellular environment of C. chodatii by enhancing cellular metabolism. Contrary to our results, reduction in the protein content of Phaeodactylum tricornutum in response to TiO2-NPs and CeO2-NPs [83] and Nannochloropsis oculata in response to CuO-NPs [6].
Oxidative stress markers in the microalga Coccomyxa in response to CaO NPs were also addressed in the present work. Reactive oxygen species are generated in the thylakoid membrane during photosynthesis [85–87]. Hydrogen peroxide has been suggested to be the main species of ROS in algae. In the present work, CaO NPs induced accumulation of hydrogen peroxide; such accumulation was significant and in a similar manner whatever the level applied (recording 20%, in average, compared to control). It has been reported that H2O2 is a widely produced ROS under abiotic/biotic stresses and normal conditions. It can either be a toxic molecule causing irreversible damage to the plant cell or can be a secondary messenger regulating the antioxidative defense depends on concentration [88]. We suggested that under CaO NPs the increase in H2O2 compared to control was not toxic to the cell, rather a secondary messenger that activates the antioxidant system from one hand. On the other hand, ROS at particular concentration have the ability to breakdown natural organic compounds and release nutrients, which might promote algal development [71]. Qiao et al. [89] found that the enhancement of cytosolic Ca2+ level could suppress the oxidative damage of H2O2 under salt stress via modulation of antioxidants and mediating the expression of calmodulin and mitogen-activated protein kinase levels, causing up-regulation of Monoraphidium sp. biomass and enhancing lipids accumulation.
The oxidative stress-related accumulation of MDA is index of oxidation of side chains of polyunsaturated fatty acids causing lipid peroxidation in algal cell membranes. In this study, the oxidative damage of H2O2 to membrane lipids were found to be restricted by CaO NPs where significant reduction of lipid peroxidation was denoted whatever the level applied. Thus, CaO NPs has no oxidative damage on the applied cells and the increase of H2O2 has no apparent damage on C. chodatii. Thus, the use of CaO NPs could have a beneficial effect against peroxidation of membrane lipids. This could be associated with the role of Ca2+ in supporting the integrity of cell membrane phospholipids and proteins [90]. Hepler [91] stated that Ca2+ attached to phospholipids, maintains lipid bilayers, and hence allows structural stability to cellular membranes. Moreover, the rise in algal cellular lipid content can elicit the protection of cellular redox homeostasis by functioning as an electron sink [92]. Moreover, the rise in algal cellular lipid content can elicit the protection of cellular redox homeostasis by functioning as an electron sink [92]. Hartmann et al. [44] revealed that the application of CaCl2 reduced the lipid peroxidation of gamma-irradiated and non-radiated Chlorella vulgaris microalgae.
The homeostasis of ROS inside the algal cell depends on the efficacy of antioxidant power of antioxidant enzymes. Superoxide dismutase, catalase, ascorbate peroxidase, and guaiacol peroxidase are ROS-metabolizing agents that curtail the exacerbation of various ROS. Superoxide dismutase is a superoxide anion-scavenging enzyme that converts superoxide anion to hydrogen peroxide and water. The activities of catalase, ascorbate peroxidase, and guaiacol peroxidase are catabolic agents of hydrogen peroxide to oxygen and water. In the present investigation, the levels of CaO NPs effectively induced the activities of the four tested enzymes which were dose-depended. The highest activities of APX and SOD when C. chodatii exposed to 60 µg mL−1 CaO NPs, while that of CAT and POD were recorded for 40 and 20/80 µg mL−1 CaO NPs, respectively. Thus, one can say that the elevated level of H2O2 for CaO NPs treated cells acts as a signaling secondary messenger that triggers the activity of H2O2-metabolizing enzymes. This signifies their involvement in the up-regulation of ROS homeostasis; hence keep C. chodatii cells devoid from oxidative stress bursts. Thus, lipid peroxidation, in the present study, was reduced even when the recorded hydrogen peroxide was increased under CaO NPs compared to control. Therefore, the findings of the present study revealed that Ca2+ plays a role in enhancing the antioxidant system, maintaining membrane stability, and reducing oxidative damage, similar to that reported for Chlorella vulgaris under gamma irradiation stress [49]. Also, Amor et al. [93] found that the Ca2+ application stimulated high activities of antioxidant enzymes, such as SOD, CAT, and APX in photosynthetic organisms. Matouke et al. [94] observed a significant improvement in the activity of SOD, POD, and CAT in Chlorella ellipsoids exposed to TiO2-NPs. Thus, the application of CaO NPs has a beneficial role in the induction of antioxidant enzymes, which prevent the enhancement of toxic levels of reactive oxygen species and membrane dysfunction in C. chodatii. Gathering together, all these modifications of algal cell revealed the importance of CaO NPs in modulation the metabolism of C. chodatii to be more efficient than normally growing ones.
The present investigation clearly demonstrated the positive effect of CaO NPs on C. chodatii growth, chlorophyll contents, and photosynthesis. In addition, all biological traits estimated in this study and data gained at various CaO NPs levels (primary metabolites and antioxidants) indicated the promotion role of CaO NPs on C. chodatii, and they might be useful tools for future valuations of the positive effects of CaO NPs on other microalgae. Furthermore, no oxidative damage of CaO NPs on algal membranes raised the importance of this nanoparticle as safe agent for up-regulating the metabolic status of alga compared to normally grown cells. The results indicated the importance of the level of 60 µg mL−1 CaO NPs on lipid production, high stability of metabolic products, and the highest dry matter acquisition of C. chodatii. Further experiments should be performed to optimize lipid production as a biofuel source under different temperatures, pH values, and carbon sources and to detect the change in lipids composition. CaO NPs greatly prevented any symptoms of oxidative stress and greatly enhanced the antioxidant system. Thus, the role of CaO NPs under various abiotic stresses should be also evaluated in future work.
Acknowledgement: The authors would like to acknowledge Prof. Dr. Refat Abdel-basset (Professor of Plant Physiology in Botany and Microbiology Department, Faculty of Science, Assiut University) for providing the Computerized Clark Type Electrode System to Oxygen Monitoring.
Funding Statement: The authors received no specific funding for this study.
Conflicts of Interest: The authors declare that they have no conflicts of interest to report regarding the present study.
References
1. Keller, A. A., McFerran, S., Lazareva, A., Suh, S. (2013). Global lifecycle releases of engineered nanomaterials. Journal of Nanoparticle Research, 15, 1692. [Google Scholar]
2. Yang, X. Y., He, Q., Guo, F. C., Liu, X. B., Chen, Y. (2021). Translocation and biotoxicity of metal (oxide) nanoparticles in the wetland-plant system. Frontiers of Environmental Science & Engineering, 15(6), 138. [Google Scholar]
3. Pikula, K., Mintcheva, N., Kulinich, S. A., Zakharenko, A., Markina, Z. et al. (2020). Aquatic toxicity and mode of action of CdS and ZnS nanoparticles in four microalgae species. Environmental Research, 186, 109513. [Google Scholar] [PubMed]
4. Gomaa, M., Yousef, N. (2020). Optimization of production and intrinsic viscosity of an exopolysaccharide from a high yielding Virgibacillus salarius BM02: Study of its potential antioxidant, emulsifying properties and application in the mixotrophic cultivation of Spirulina platensis. International Journal of Biological Macromolecules, 149, 552–561. [Google Scholar] [PubMed]
5. Saxena, P., Saharan, V., Baroliya, P. K., Gour, V. S., Rai, M. K. et al. (2021). Mechanism of nanotoxicity in Chlorella vulgaris exposed to zinc and iron oxide. Toxicology Reports, 8, 724–731. [Google Scholar] [PubMed]
6. Fazelian, N., Yousefzadi, M., Movafeghi, A. (2020). Algal response to metal oxide nanoparticles: Analysis of growth, protein content, and fatty acid composition. BioEneregy Research, 13, 944–954. [Google Scholar]
7. Barreto, D. M., Tonietto, A. E., Amaral, C. D. B., Pulgrossi, R. C., Polpo, A. et al. (2019). Physiological responses of Chlorella sorokiniana to copper nanoparticles. Environmental Toxicology and Chemistry, 38, 387–395. [Google Scholar] [PubMed]
8. Barreto, D. M., Tonietto, A. E., Lombard, A. T. (2021). Environmental concentrations of copper nanoparticles affect vital functions in Ankistrodesmus densus. Aquatic Toxicology, 23, 1105720. [Google Scholar]
9. Gunasekaran, D., Chandrasekaran, N., Jenkins, D., Mukherjee, A. (2020). Plain polystyrene microplastics reduce the toxic effects of ZnO particles on marine microalgae Dunaliella salina. Journal of Environmental Chemical Engineering, 8(5), 104250. [Google Scholar]
10. Ahmed, I., Zhang, B., Muneeb-Ur-Rehman, M., Fan, W. (2021). Effect of different shapes of Nano-Cu2O and humic acid on two-generations of Daphnia Magna. Ecotoxicology and Environmental Safety, 207, 111274. [Google Scholar] [PubMed]
11. Kong, Y., Sun, H., Zhang, S., Zhao, B., Zhao, Q. et al. (2021). Oxidation process of lead sulfide nanoparticle in the atmosphere or natural water and influence on toxicity toward Chlorella vulgaris. Journal of Hazardous Materials, 417, 126016. [Google Scholar] [PubMed]
12. Abdel-Basset, R., Bader, K. P. (1999). Effects of stress conditions and calcium on the light induced hydrogen gas exchange in Oscillatoria chalybea. Journal of Plant Physiology, 155, 86–92. [Google Scholar]
13. Hochmal, A. K., Schulze, S., Trompelt, K., Hippler, M. (2015). Calcium-dependent regulation of photosynthesis. Biochimic Biophysic Acta, 1847, 993–1003. [Google Scholar]
14. Ferraz, E., Gamelas, J. A. F., Coroado, J., Monteiro, C., Rocha, F. (2018). Eggshell waste to produce building lime: Calcium oxide reactivity, industrial, environmental and economic implications. Materials and Structures, 51, 115. [Google Scholar]
15. Bano, S., Pillai, S. (2020). Green synthesis of calcium oxide nanoparticles at different calcination temperatures. World Journal of Science Technology and Sustainable Development, 17, 283–295. [Google Scholar]
16. Abo-Zeid, Y., Williams, G. R. (2020). The potential anti-infective applications of metal oxide nanoparticles: A systematic review. Wiley Interdisciplinary Reviews: Nanomedicine and Nanobiotechnology, 12, e1592. [Google Scholar] [PubMed]
17. Canaparo, R., Foglietta, F., Limongi, T., Serpe, L. (2021). Biomedical applications of reactive oxygen species generation by metal nanoparticles. Materials, 14, 53. [Google Scholar]
18. Gandhi, N. (2021). Facile and eco-friendly method for synthesis of calcium oxide (CaO) nanoparticles and its potential application in agriculture. Saudi Journal of Life Science, 6, 89–103. [Google Scholar]
19. Meier, S., Moore, F., Morales, A., González, M. E., Seguel, A. et al. (2020). Synthesis of calcium borate nanoparticles and its use as a potential foliar fertilizer in lettuce (Lactuca sativa) and zucchini (Cucurbita pepo). Plant Physiology and Biochemistry, 151, 673–680. [Google Scholar] [PubMed]
20. Rivasseau, C., Farhi, E., Atteia, A., Couté, A., Gromova, M. et al. (2013). An extremely radioresistant green eukaryote for radionuclide bio-decontamination in the nuclear industry. Energy & Environmental Science, 6, 1230–1239. [Google Scholar]
21. Wang, C., Wang, Z., Luo, F. (2017). The augmented lipid productivity in an emerging oleaginous model alga Coccomyxa subellipsoidea by nitrogen manipulation strategy. World Journal of Microbiology and Biotechnology, 33, 160. [Google Scholar] [PubMed]
22. Abd El-Aziz, D. M., Yousef, N. M. H. (2017). Antimicrobial effects of calcium oxide nanoparticles and some spices in minced meat. ARC Journal of Animal and Veterinary Sciences, 3, 38–45. [Google Scholar]
23. Yousef, N. M. H., Mawad, A. M., Abeed, A. H. (2019). Enhancement the cellulase activity induced by endophytic bacteria using calcium nanoparticles. Current Microbiology, 76, 346–354. [Google Scholar] [PubMed]
24. Yousef, N. M. H., Nafady, N. A. (2014). Combining biological silver nanoparticles with antiseptic agent and their antimicrobial activity. International Journal of Pure and Applied Bioscience, 2, 39–47. [Google Scholar]
25. Rippka, R., Herdmann, M. (1993). Pasteur culture collection of cyanobacterial strains in axenic culture catalog of strains. In: Environment open journal of ecology, vol. 2, pp. 21–28. Paris, France: Institute Pasteur. [Google Scholar]
26. Biedermann, W., Schwarzenbach, G. (1948). The complexometric titration of alkaline earths and some other metals with eriochromeblack T. Chimia, 2, 56. [Google Scholar]
27. Marker, A. F. H. (1972). The use of acetone and methanol in the estimation of chlorophyll in the presence of phaeophytin. Fresh Water Biology, 2(4), 361–385. [Google Scholar]
28. Lowry, O. H., Rosebrough, N. J., Farr, A. L., Randall, R. G. (1951). Protein measurement with Folin phenol reagent. Journal of Biological Chemistry, 193(1), 265–275. [Google Scholar] [PubMed]
29. Moore, S., Stein, W. W. (1948). Amino acid free photometric ninhydrin method for use in chromatography of amino acids. Journal of Biological Chemistry, 176, 367–388. [Google Scholar] [PubMed]
30. Fales, F. W. (1951). The assimilation and degradation of carbohydrates by yeast cells. Journal of Biological Chemistry, 193, 113–118. [Google Scholar] [PubMed]
31. Schlegel, H. G. (1956). Die verwertung organischer sauren duch cholrella in licht. Planta, 47, 510–526. [Google Scholar]
32. Hara, A., Radin, N. S. (1978). Lipid extraction of tissues with a low toxicity solvent. Analytical Biochemistry, 90, 420–426. [Google Scholar] [PubMed]
33. Yu, C. W., Murphy, T. M., Lin, C. H. (2003). Hydrogen peroxide-induces chilling tolerance in mung beans mediated through ABA-independent glutathione accumulation. Functional Plant Biology, 30, 955–963. [Google Scholar] [PubMed]
34. Madhava Rao, K. V., Sresty, T. V. (2000). Antioxidative parameters in seedlings of pigeon pea (Cajanus cajan L. Millspaugh) in response to Zn and Ni stresses. Plant Science, 157, 113–128. [Google Scholar]
35. Misra, H. P., Fridovich, I. (1972). The role of superoxide anion in the autoxidation of epinephrine and a simple assay for superoxide dismutase. Journal of Biological Chemistry, 247, 3170–3175. [Google Scholar] [PubMed]
36. Aebi, A. (1984). Catalase in vitro. Methods Enzymology, 105, 121–126. [Google Scholar]
37. Matsumura, T., Tabayashi, N., Kamagata, Y., Souma, C., Saruyama, H. (2002). Wheat catalase expressed in transgenic rice can improve tolerance against low temperature stress. Physiologia Plantarum, 116(3), 317–327. [Google Scholar]
38. Tatiana, Z., Yamashita, K., Matsumoto, H. (1999). Iron deficiency induced changes in ascorbate content and enzyme activities related to ascorbate metabolism in cucumber root. Plant Cell Physiology, 40(3), 273–280. [Google Scholar]
39. Nakano, K., Asada, Y. (1981). Hydrogen peroxide is scavenged by ascorbate-specific peroxidase in spinach chloroplasts. Plant Cell Physiology, 22, 867–880. [Google Scholar]
40. Park, H., Park, H. J., Kim, J. A., Lee, S. H., Kim, J. K. (2011). Inactivation of Pseudomonas aeruginosa PA01 biofilms by hyperthermia using super paramagnetic nanoparticles. Journal of Microbiology Methods, 84(1), 41–45. [Google Scholar]
41. Nguemezi, J. K., Tatchago, V. (2010). Effects of fertilizers containing calcium and/or magnesium on the growth, development of plants and the quality of tomato fruits in the western highlands of Cameroon. International Journal of Agricultural Research, 5(10), 821–831. [Google Scholar]
42. Abdel-Kader, H. A. A. (2022). Short- and long-terms stimulatory impacts of calcium oxide nanoparticles (CaONPs) on the growth, photosynthesis and antioxidant enzymes of Chlorella sp. Assiut University Journal of Multidisciplinary Scientific Research, 1, 242–262. [Google Scholar]
43. Gorain, P. C., Bagchi, S. K., Mallick, N. (2013). Effects of calcium, magnesium and sodium chloride in enhancing lipid accumulation in two green microalgae. Environmental Technology, 34, 1887–1894. [Google Scholar] [PubMed]
44. Hartmann, N. B., Von der Kammer, F., Hofmann, T., Baalousha, M., Ottofuelling, S. (2010). Algal testing of titanium dioxide nanoparticles-testing considerations, inhibitory effects and modification of cadmium bioavailability. Toxicology, 269, 190–197. [Google Scholar] [PubMed]
45. Wang, X., Yang, X., Chen, S., Li, Q., Wang, Q. W. et al. (2015). Zinc oxide nanoparticles affect biomass accumulation and photosynthesis in Arabidopsis. Frontiers in Plant Science, 6, 1–9. [Google Scholar]
46. Nam, S. H., Kwak, J. K., An, Y. J. (2018). Quantification of silver nanoparticle toxicity to algae in soil via photosynthetic and flow-cytometric analyses. Scientific Reports, 8, 1–12. [Google Scholar]
47. Middepogu, A., Hou, J., Gao, X., Lin, D. (2018). Effect and mechanism of TiO2 nanoparticles on the photosynthesis of Chlorella pyrenoidosa. Ecotoxicology and Environmental Safety, 161, 497–506. https://doi.org/10.1016/jecoenv201806027 [Google Scholar] [CrossRef]
48. Melegari, S. P., Perreault, F., Costa, R. H., Popovic, R., Matias, W. G. (2013). Evaluation of toxicity and oxidative stress induced by copper oxide nanoparticles in the green alga Chlamydomonas reinhardtii. Aquatic Toxicology, 142–143, 431–440. https://doi.org/10.1016/j.aquatox.2013.09.015 [Google Scholar] [PubMed] [CrossRef]
49. Husseini, Z. N., Tafreshi, S. A. H., Aghaie, P., Toghyani, M. A. (2020). CaCl2 pretreatment improves gamma toxicity tolerance in microalga Chlorella vulgaris. Ecotoxicology and Environmental Safety, 192, 110261. [Google Scholar] [PubMed]
50. Chen, L., Zhou, L., Liu, L., Deng, S., Wu, H. et al. (2012). Toxicological effects of nanometer titanium dioxide (nano-TiO2) on Chlamydomonas reinhardtii. Ecotoxicology and Environmental Safety, 84, 155–162. [Google Scholar] [PubMed]
51. Govorov, A. O., Carmeli, I. (2007). Hybrid structures composed of photosynthetic system and metal nanoparticles: Plasmon enhancement effect. Nano Letters, 7, 620–625. [Google Scholar] [PubMed]
52. Serag, M. F., Kaji, N., Habuchi, S., Bianco, A., Baba, Y. (2013). Nanobiotechnology meets plant cell biology: Carbon nanotubes as organelle targeting nanocarriers. RSC Advances, 3(15), 4856–4862. [Google Scholar]
53. Xu, J., Luo, X., Wang, Y., Feng, Y. (2018). Evaluation of zinc oxide nanoparticles on lettuce (Lactuca sativa L.) growth and soil bacterial community. Environmental Science and Pollution Research International, 25(6), 6026–6035. [Google Scholar] [PubMed]
54. Giraldo, J. P., Landry, M. P., Faltermeier, S. M., McNicholas, T. P., Iverson, N. M. et al. (2014). Plant nanobionics approach to augment photosynthesis and biochemical sensing. Nature Materials, 13(4), 400–408. [Google Scholar] [PubMed]
55. Lambreva, M. D., Lavecchia, T., Tyystjärvi, E., Antal, T. K., Orlanducci, S. et al. (2015). Potential of carbon nanotubes in algal biotechnology. Photosynthesis Research, 125(3), 451–471. [Google Scholar] [PubMed]
56. Chen, J. E., Smith, A. G. (2012). A look at diacylglycerol acyltransferases (DGATs) in algae. Journal of Biotechnology, 162(1), 28–39. [Google Scholar] [PubMed]
57. Wang, Q., Yang, S., Wan, S., Li, X. (2019). The significance of calcium in photosynthesis. International Journal of Molecular Sciences, 20, 1353. https://doi.org/10.3390/ijms20061353 [Google Scholar] [PubMed] [CrossRef]
58. Wang, X., Zhang, J., Sun, W., Yang, W., Cao, J. et al. (2015). Anti-algal activity of palladium oxide-modified nitrogendoped titanium oxide photocatalyst on Anabaena sp. PCC 7120 and its photocatalytic degradation on Microcystin LR under visible light illumination. Chemical Engineering Journal, 264, 437–444. [Google Scholar]
59. Atkin, O. K., Bruhn, D., Hurry, V. M., Tjoelker, M. G. (2005). Evans review no. 2: The hot and the cold: Unravelling the variable response of plant respiration to temperature. Functional Plant Biology, 32(2), 87–105. [Google Scholar] [PubMed]
60. Tovar-Méndez, A., Miernyk, J. A., Randall, D. D. (2003). Regulation of pyruvate dehydrogenase complex activity in plant cells. European Journal of Biochemistry, 270(6), 1043–1049. [Google Scholar]
61. Miao, A. J., Schwehr, K. A., Xu, C., Zhang, S. J., Luo, Z. et al. (2009). The algal toxicity of silver engineered nanoparticles and detoxification by exopolymeric substances. Environmental Pollution, 157, 3034–3041. https://doi.org/10.1016/jenvpol200905047 [Google Scholar] [CrossRef]
62. Deng, X. Y., Cheng, J., Hu, X. L., Wang, L., Li, D. et al. (2017). Biological effects of TiO2 and CeO2 nanoparticles on the growth, photosynthetic activity, and cellular components of a marine diatom Phaeodactylum tricornutum. Science of the Total Environment, 575, 87–96. [Google Scholar] [PubMed]
63. Marchello, A. E., Barreto, D. M., Lombardi, A. L. (2018). Effects of titanium dioxide nanoparticles in different metabolic pathways in the freshwater microalga Chlorella sorokiniana (Trebouxiophyceae). Water, Air, and Soil Pollution, 229, 48. https://doi.org/10.1007/s11270-018-3705-5 [Google Scholar] [CrossRef]
64. Huang, J., Cheng, J., Yi, J. (2016). Impact of silver nanoparticles on marine diatom Skeletonema costatum. Journal of Applied Toxicology, 36, 1343–1354. https://doi.org/10.1002/jat3325 [Google Scholar] [CrossRef]
65. Bolouri-Moghaddam, M. R., Le Roy, K., Xiang, L., Rolland, F., van den Ende, W. (2010). Sugar signaling and antioxidant network connections in plant cells. FEBS Journal, 277, 2022–2037. [Google Scholar] [PubMed]
66. Mohamed, Z. (2008). Polysaccharides as a protective response against microcystin-induced oxidative stress in Chlorella vulgaris and Scenedesmus quadricauda and their possible significance in the aquatic ecosystem. Ecotoxicology, 17, 504–516. [Google Scholar] [PubMed]
67. Zinicovscaia, I., Chiriac, T., Cepoi, L., Rudi, L., Culicov, O. et al. (2017). Selenium uptake and assessment of the biochemical changes in Arthrospira (Spirulina platensis) biomass during the synthesis of selenium nanoparticles. Candian Journal of Microbiology, 63, 27–34. https://doi.org/10.1139/cjm-2016-0339 [Google Scholar] [PubMed] [CrossRef]
68. Rodolfi, L., Zittelli, G. C., Bassi, N., Padovani, G., Biondi, N. et al. (2009). Microalgae for oil: Strain selection, induction of lipid synthesis and outdoor mass cultivation in a low-cost photobioreactor. Biotechnology and Bioengineering, 102, 100–112. [Google Scholar] [PubMed]
69. Guarnieri, M. T., Nag, A., Smolinski, S. L., Darzins, A., Seibert, M. et al. (2011). Examination of triacylglycerol biosynthetic pathways via de novo transcriptomic and proteomic analyses in an unsequenced microalga. PLoS One, 6(10), e25851. [Google Scholar] [PubMed]
70. Chen, H., Hu, J., Qipao, Y., Chen, W., Rong, J. et al. (2015). Ca2+—Regulated cyclic electron flow supplies ATP for nitrogen starvation-induced lipid biosynthesis in green alga. Scientific Reports, 5, 15117. [Google Scholar] [PubMed]
71. Kim, S. C., Lee, D. K. (2005). Preparation of TiO2-coated hollow glass beads and their application to the control of algal growth in eutrophic water. Microchemistry Journal, 80, 227–232. [Google Scholar]
72. Nair, A., Chakraborty, S. (2020). Synergistic effects between autotrophy and heterotrophy in optimization of mixotrophic cultivation of Chlorella sorokiniana in bubble-column photobioreactors. Algal Research, 46, 101799. [Google Scholar]
73. Pancha, I., Chokshi, K., George, B., Ghosh, T., Paliwal, C. et al. (2014). Nitrogen stress triggered biochemical and morphological changes in the microalgae Scenedesmus sp. CCNM 1077. Bioresource Technology, 156, 146–154. https://doi.org/10.1016/jbiortech201401025 [Google Scholar] [CrossRef]
74. Arora, N., Philippidis, G. P. (2021). Insights into the physiology of Chlorella vulgaris cultivated in sweet sorghum bagasse hydrolysate for sustainable algal biomass and lipid production. Scientific Reports, 11, 1–14. [Google Scholar]
75. Dev Sarkar, R., Singh, H. B., Chandra, K. M. (2021). Enhanced lipid accumulation in microalgae through nanoparticle-mediated approach, for biodiesel production: A mini-review. Heliyon, 7(9), e08057. https://doi.org/10.1016/jheliyon2021e08057 [Google Scholar] [CrossRef]
76. He, M., Yan, Y., Pei, F., Wu, M., Gebreluel, T. et al. (2017). Improvement of lipid production by Scenedesmus obliquus triggered by low dose exposure to nanoparticles. Scientific Reports, 7(1), 1–12. [Google Scholar]
77. Shanab, S. M. M., Partila, A. M., Ali, H. E. A., Abdullah, M. A. (2019). Characterization and impact of silver nanoparticles on cell growth, lipid, carbohydrate and fatty acids of Chlorella vulgaris and Dictyochloropsis splendida. Beilstein Archives, 2019, 201991. [Google Scholar]
78. Xia, L., Ge, H., Zhou, X., Zhang, D., Hu, C. (2013). Photoautotrophic outdoor two-stage cultivation for oleaginous microalgae Scenedesmus obtusus XJ-15. Bioresource Technology, 144, 261–267. [Google Scholar] [PubMed]
79. Kadar, E., Rooks, P., Lakey, C., White, D. A. (2012). The effect of engineered iron nanoparticles on growth and metabolic status of marine microalgae cultures. Science of the Total Environment, 439, 8–17. [Google Scholar] [PubMed]
80. Rana, M. S., Bhushan, S., Sudhakar, D. R., Prajapati, S. K. (2020). Effect of iron oxide nanoparticles on growth and biofuel potential of Chlorella spp. Algal research, 49, 101942. [Google Scholar]
81. Hasnain, M., Munir, N., Abideen, Z., Dias, D. A., Aslam, F. et al. (2023). Applying silver nanoparticles to enhance metabolite accumulation and biodiesel production in new algal resources. Agriculture, 13(1), 73. [Google Scholar]
82. Sabatini, S. E., Juárez, A. B., Eppis, M. R. L., Bianchi, L., Luquetcm, M. et al. (2009). Oxidative stress and antioxidant defenses in two green microalgae exposed to copper. Ecotoxicology and Environmental Safety, 72, 1200–1206. [Google Scholar] [PubMed]
83. Deng, X. Y., Cheng, J., Hu, L., Wang, K., Gao, K. (2017). Biological effects of TiO2 and CeO2 nanoparticles on the growth, photosynthetic activity, and cellular components of a marine diatom Phaeodactylum tricornutum. Science of the Total Environment, 575, 87–96. [Google Scholar] [PubMed]
84. Nimptsch, J., Wunderlin, D. A., Dollan, A., Pflugmacher, S. (2005). Antioxidant and biotransformation enzymes in Myriophyllum quitense as biomarkers of heavy metal exposure and eutrophication in Suquía River Basin (Córdoba, Argentina). Chemosphere, 61, 147–157. [Google Scholar] [PubMed]
85. Dawood, M. F., Abu-Elsaoud, A. M., Sofy, M. R., Mohamed, H. I., Soliman, M. H. (2022). Appraisal of kinetin spraying strategy to alleviate the harmful effects of UVC stress on tomato plants. Environmental Science and Pollution Research, 29, 52378–52398. [Google Scholar] [PubMed]
86. Sheteiwy, M., Ulhassan, Z., Qi, W., Lu, H., AbdElgawad, H. et al. (2022). Association of jasmonic acid priming with multiple defense mechanisms in wheat plants under high salt stress. Frontiers in Plant Science, 26, 14. [Google Scholar]
87. Dawood, M. F. A., Sofy, M. R., Mohamed, H. I., Sofy, A. R., Abdel-Kader, H. A. A. (2022). N- or/and P-deprived Coccomyxa chodatii SAG 216-2 extracts instigated mercury tolerance of germinated wheat seedlings. Plant and Soil, 2022, 1–29. [Google Scholar]
88. Younes, N. A., Dawood, M. F., Wardany, A. A. (2019). Biosafety assessment of graphene nanosheets on leaf ultrastructure, physiological and yield traits of Capsicum annuum L. and Solanum melongena L. Chemosphere, 228, 318–327. [Google Scholar] [PubMed]
89. Qiao, T., Zhao, Y., Zhong, D. B., Yu, X. (2021). Hydrogen peroxide and salinity stress act synergistically to enhance lipids production in microalga by regulating reactive oxygen species and calcium. Algal Research, 53, 102017. [Google Scholar]
90. Pinto, E., Sigaud-kutner, T. C., Leitao, M. A., Okamoto, O. K., Morse, D. et al. (2003). Heavy metal-induced oxidative stress in algae. Journal of Phycolology, 39, 1008–1018. [Google Scholar]
91. Hepler, P. K. (2005). Calcium: A central regulator of plant growth and development. The Plant Cell, 17(8), 2142–2155. [Google Scholar] [PubMed]
92. Upadhyaya, H., Panda, S. K., Dutta, B. K., Kuntze, O. (2011). CaCl2 improves post drought recovery potential in Camellia sinensis (L). Plant Cell Reports, 30, 495–503. [Google Scholar] [PubMed]
93. Amor, N. B., Megdiche, W., Jiménez, A., Sevilla, F., Abdelly, C. (2010). The effect of calcium on the antioxidant systems in the halophyte Cakile maritima under salt stress. Acta Physiologiae Plantarum, 32(3), 453–461. [Google Scholar]
94. Matouke, M. M., Elewa, D. T., Abdullahi, K. (2018). Binary effect of titanium dioxide nanoparticles (nTiO2) and phosphorus on microalgae (Chlorella ellipsoides Gerneck, 1907). Aquatic Toxicology, 198, 40–48. [Google Scholar] [PubMed]
Cite This Article
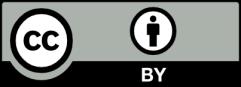
This work is licensed under a Creative Commons Attribution 4.0 International License , which permits unrestricted use, distribution, and reproduction in any medium, provided the original work is properly cited.