Open Access
REVIEW
A Review on Selenium Function under Oxidative Stress in Plants Focusing on ROS Production and Detoxification
1 Horticulture and Crop Research Department, Kermanshah Agricultural and Natural Resources Research and Education Center (AREEO), Kermanshah, Iran
2 Department of Agricultural Biotechnology, Faculty of Agriculture, Lorestan University, Khorramabad, Iran
* Corresponding Author: Armin Saed-Moucheshi. Email:
(This article belongs to the Special Issue: Selenium, Silicon and their Nanoparticles-mediated Environmental Stress Tolerance in Crop Plants)
Phyton-International Journal of Experimental Botany 2023, 92(7), 1921-1941. https://doi.org/10.32604/phyton.2023.027810
Received 13 December 2022; Accepted 03 January 2023; Issue published 29 May 2023
Abstract
One of the main reasons of the annual reduction in plant production all around the world is the occurrence of abiotic stresses as a result of an unpredicted changes in environmental conditions. Abiotic stresses basically trigger numerous pathways related to oxygen free radicals’ generation resulting in a higher rate of reactive oxygen species (ROS) production. Accordingly, higher rate of oxygen free radicals than its steady state causes to oxidize various types of molecules and compartments within the plants’ cells and tissues. Oxidative stress is the result of high amount free radicals of oxygen interfering with different functions leading to undergo significant changes from molecular to phenotypic levels. In response to oxidative stress, plants deploy different enzymatic and non-enzymatic antioxidant mechanisms to detoxify extra free radicals and get back to a normal state. Applying some specific treatments have shown to significantly affect the antioxidant capacity and efficiency of the stressed cells and compartments. One of such reportedly effective treatments is the utilization of selenium (Se) element in stressed plants. Over the past years some different experiments evaluated the probable effect or efficiency of Se regarding its impact on plant under oxidative stress. Accordingly, based on the recent studies, Se has a significant role in plant responses to abiotic stresses probably due to its ability to improve the plants’ tolerance to oxidative stress. The significant influences of Se, and its related components such as nano-selenium, in plants under oxidative stress rooting from abiotic stresses, along with the new finding pertaining to its metabolism and translocation mechanisms inside the plant cells under oxidative stress condition are clearly explained in this review. However, there are still lack of a comprehensive explanation related to the precise mechanism of Se in plants under oxidative stress.
Keywords
Water, nutrients, and gas elements (oxygen and carbon) that are provided by the environment are the most important components required for plant growth and production. In addition, an efficient environment is able to provide light, temperature, and humidity at proper rates (as it simplified in Fig. 1). Providing a proper rate of such requirements would lead plants to perfectly grow up to their genetic potential. Quite the contrary, if one of the requirements alters from a proper rate, the plant would respond by reducing their growth and production. The changes in environmental condition that results in negative responses of a plant and less plant production, is referred to as an environmental stress condition [1]. There are different types of environmental stresses, e.g., drought and salinity stresses, that have been globally affecting plant production, and negatively impacted the human nutritional values that are provided by plants.
Figure 1: A very simple portrait of plant requirements to germinate and grow: changing each these requirements would lead to alter the conditions inside the plants
Different environmental stresses have shown similarities in their impacts on the plants in some molecular and biochemical, physiological, and phenotypic levels [2,3]. One of the most common impacts of environmental stresses is to increase the production of free oxygen radicals leading to indirect oxidative stress. In response, the plants have developed various types of antioxidative defense mechanisms to reduce the rate of free radicals and go back to their steady state level [4,5].
Numerous studies [3,5–8] have indicated that the plants responses to the same stresses could be altered under diverse conditions. Some conditions can improve, or put down, the efficiency of antioxidant mechanisms and other systemic defenses in the plants. As it was mentioned, application of some treatments on the plants or their environment under stress condition can ameliorate, otherwise decrease, their tolerance to different types of stresses. Different treatments have been frequently tested on the various plants to study their efficacy regarding the plant responses to stresses and their tolerance rates [7–9]. Application of nutritional elements and plant hormones as treatment on different plant parts have commonly been examined [10]. However, some of these elements, such as selenium (Se), have obtained less attention comparing to others. Selenium is not a macro element (which is required in great quantities in plant), but instead its availability in different rates has various impacts on the plants. In the study of Dai et al. [11], high content of this element in plants led to nutritional toxicity in rice; while in the study of Perez-Millan et al. [12] application of its proper amount resulted in higher tolerance of pepper under cadmium stress condition.
In this review we attempt to provide an overview to understand the molecular biology and physiological mechanisms along with the other internal processes involved in the plant response to oxidative stress; and illustrate the different impacts of Se on plant production focusing on its pros and cons under abiotic and oxidative stresses.
2 Abiotic and Environmental Stresses Association with Oxidative Stress
Oxygen is the most significant element on earth that has led to produce and develop living organisms on this planet. Oxygen molecule with atomic number of 8, includes in the same group as Se and S (Sulfur), carries two unpaired and free electrons with a short distance (orbital) from the nucleus of the atom making them so active, and passionate, to attract other electrons wherever they find and share their space as two separate couples. All the important roles of oxygen in all living organism comes back to its chemical activity to bond with other elements or compounds.
Hydrogen (H), which has one free electron, can share its electron with one of the free ones in the oxygen atoms. By sharing two electrons from on oxygen atom with two electrons from two H atoms, the water molecule (H2O) would be produced. H2O molecules that are absorbed by the plants’ root from the soil, would be mainly transported to the leaves to be used in Calvin-cycle as electron donors and produce hydrocarbon molecules [4]. There are also other compounds of oxygen that are produced in plant organelles such as mitochondria and peroxisomes in line with photosynthetic compartments. A group of such oxygen compounds that are normally produced and used in plant organelles are reactive oxygen species, or ROS in short. ROS contains some highly reactive compounds, because of their free electron(s), such as hydrogen peroxide (H2O2), singlet oxygen (1O2), hydroxyl radical (OH•), and superoxide (O2−). These products are comparable to a double-edged sword having both advantages and disadvantages in plants [13]. ROSs under normal state could be used as signaling molecules and for neutralizing some extra or unrequired proteins and/or other compounds within the plant cells. Though, under excessive generation of ROS in plants, these oxygen compounds are able to damage almost all organelles of a plant cell. Enhanced production of ROS, can endanger cellular life by triggering some events such as the initiation of programmed cell death (PCD) mechanism, protein oxidation, lipid peroxidation, enzyme inhibition, and direct nucleic acid damage [14,15]. Exceeding the ROS generation from its steady state causes oxidative stress to happen in plants [16]. The name oxidative stress is derived from the name of oxygen, as the main part of all ROS compounds that cause oxidation of the other cellular compounds [17].
The main source of ROS generation are chloroplast compartments that use carbon dioxide (CO2) H2O2 as input and produce carbohydrates as output. However, environmental conditions can change the situations inside the chlorophyll molecules causing it to fail to do its role properly. Under the condition of unbalanced utilization of energy (inflated electron from H2O) in chlorophyll photosystems, the exceeded rate of electrons would be probably transported to O2 and result in ROS generation. Therefore, the ROS photoproduction can change under environmental and abiotic stresses (mentioned in Fig. 2) [18].
Figure 2: Depiction of different types of abiotic stresses in plants causing indirect oxidative stress
3 The Pros and Cons of Oxidants and Antioxidants in Plants
In plants, a steady state exists in which there is a balance between ROS generation and deactivation caused by antioxidant systems. Antioxidants are like catalyzers that deactivate and scavenge ROS by catalyzing some reactions that transfer the ultra-electron of ROS to other compounds [16]. The antioxidant defensing systems are available in almost all plant cellular compartments and organs indicating the importance of ROS detoxification for cellular survival [8,19]. On the other hand, the defense systems are also available in the apoplast [20]. By surpassing ROS generation from the overall antioxidative potential of plant under abnormal conditions, oxidative stress happens which induces adverse impacts on plant growth and development (Fig. 3).
Figure 3: Depicting the results of balance and imbalance generation and detoxification of ROS
By occurring abiotic stresses leading to unbalanced state of ROS generation and utilization, plant responds via activating various stress signaling pathways that trigger the activation of antioxidative mechanisms to cope with the stress condition. There are two main types of antioxidative mechanisms in plants containing non-enzymatic and enzymatic counterparts (Fig. 4). Some phenolic compounds and alkaloids, non-protein amino acids, α-tocopherol, glutathione (GSH), and ascorbate (AsA) are among the efficient non-enzymatic mechanisms in plants; while, superoxide dismutase (SOD), ascorbate peroxidase (APX), catalase (CAT), peroxidases (POX), monodehydroascorbate reductase (MDHAR), glutathione S-transferase (GST), glutathione peroxidase (GPX), glutathione reductase (GR), and dehydroascorbate reductase (DHAR) are the most important enzymatic antioxidants (Table 1) [4,21].
Figure 4: Different enzymatic and non-enzymatic antioxidants and their effect under oxidative stress
According to different reports, such as Xiao et al. [32] and Wang et al. [33], Ascorbate (AsA) plays an important role in plant stress tolerance. Ascorbate mainly synthesizes in higher plants’ cytosol largely from d-glucose, and it is able to react with OH., H2O2, O2•− and 1O2. In AsA-GSH cycle, APX utilizes two AsA molecules and reduce H2O2 to H2O and leads to the formation of monodehydroascorbate (MDHA), which is a short life radical that can be deactivated by AsA. AsA involves in zeaxanthin synthesis which scatters the additional light energy in thylakoid membranes resulting in avoiding oxidative damage [34]. In thylakoid membranes, α-tocopherol, along with tocopherol types, is available in high amounts. The evidences from scientific studies [35] suggest that tocopherols have an important role as antioxidant components. Tocopherols are involved restricting the degree of lipid peroxidation by transforming the lipid peroxyl radicals (LOO•) to hydroperoxides. In addition, tocopherols are able to efficiently quench 1O2 in chloroplasts and it has been verified that one α-tocopherol molecule detoxifies up to 120 1O2 molecules via transferring energy [36].
As an enzymatic antioxidant, SOD is the defense frontline against oxidants. It reduces O2•− to H2O2 and O2. There are three types of SOD based upon the type of the metal ion that are attached to its active site: iron (FeSOD), manganese (MnSOD), and copper/zinc (Cu/ZnSOD). FeSOD is mainly localized in the chloroplasts of some higher plants, MnSOD are usually available in the mitochondria matrix and peroxisomes, and Cu/ZnSOD largely exists in chloroplasts and cytosol [16]. H2O2, that generates from either the direct effect of abiotic stresses or from the SOD activation, can be converted to H2O and O2 by CAT [3]. CAT is generally localized in glyoxysomes and peroxisomes and one of its molecules is able to reduce about 6,000,000 molecules of H2O2 in one minute. However, there are different studies in which the activities of CAT molecules have been altered by different abiotic stresses [8,15,16]. Another crucial player in plant’s antioxidant system and oxidative stress tolerance associated with the abiotic stress is GR. It can either join the AsA in AsA-GSH cycle or act separately under oxidative stress conditions. In addition to its role in accelerating the H2O2 scavenging, GR sustains the higher proportion of GSH per Glutathione disulfide (GSSG) in plant cells and gives a balance to GSH pool (Fig. 5). Consequently, GSH can also be used by GPX, with many different isozymes, for reducing H2O2 and lipid hydroperoxides (LOOHs) [37]. Furthermore, GPX is able to repair the lipid peroxidation of cell membrane, and it plays an effective role under oxidative stress as well [38].
Figure 5: Glutathione (GSH) activity in removal of free radicals in plants
4 Potential Roles of Selenium in the Regulation of the Antioxidant Defense in Response to Abiotic Stresses
4.1 An Overview on Selenium As a Natural Element
As the main dietary source for both human and animals, plants require different macro and micro nutrients to grow and provide healthy products. According to Fan et al. [39], there are at least 17 crucial elements, both micro and macro nutrients, required for growth and development of plants. Micro nutrients such as copper (Cu), zinc (Zn), and iron (Fe) are the elements that are required in small quantities while macro nutrients including nitrogen (N), phosphorous (P), potassium (K), and sometimes calcium (Ca) are those with more essential roles and higher required amounts [40]. In addition to the requirement of plants to the aforementioned essential minerals, that are crucial for their survival and obtaining a minimum growth and development, there are some other minerals that are required for plants to reach their optimal growth and development. On the other hand, plants are sessile organisms without any mobilization or transportation and they commonly face various environmental stresses [3,16]. Subsequently, the minerals that are required for providing the optimal conditions for plants might play significant roles in plants’ tolerance to environmental stresses. These beneficial elements are able to assist the plants to cope with the unpredicted harsh conditions. Selenium, along with silicon, are among such beneficial minerals that help crops to achieve their potential developments and cope with the different environmental stresses [41,42].
The word selenium, was firstly coined in 1817 by a Swedish scientist, Jons Jacob Berzelius [43], and it is derived from ‘selene’ which is a Greek word meaning ‘moon’, as in English the word ‘selenophile’ indicates someone who loves the moon. In the periodic table, Se is placed in the 16th elemental group (column), known as chalcogens or oxygen family, and fourth period (row); mostly dedicating to the non-metalloids with a 34 atomic number and an atomic weight of 79. There are five naturally stable isotopes of Se (74Se, 76Se, 77Se, 78Se, 80Se) and its most abundant isotope, occurring in almost 50% Se cases in nature, is 80Se. Generally, the properties of Se are intermediate between sulfur (S: in above row of Se in the periodic table) having an atomic number of 16, and tellurium (Te: in the above row of Se in the periodic table) with an atomic number of 52. In addition, Se shows some chemical and physical similarity to arsenic (As: at the fourth row of the periodic table next to Se) with 16 atomic number. However, according to Bodnar et al. [43], S is the most similar element in properties to Se. In nature, selenite (Se2−), and other probable pure compounds of Se are rare and it normally occurs as sulfides of cupper (Cu), gold (Au), lead (Pb), sodium (Na) as well as Cu pyrites [44,45].
The available matters on earth show different size and molecular diameters which can be used for their classification. According to different literatures [46,4,18], the particle of matters sizing from 1 and 100, generally up to 500 nanometers (nm) in diameter are known as nanoparticles (ultrafine particles), and the particles with 1–1000 micrometers (µm) are considered as microparticles (Fig. 6). Additionally, since the diameter size of an atom is ordinarily less than 1 nm, the particles around such size are less common but the can be called atom clusters [47]. While the nanoparticles are commonly occurring in nature, the content of microparticles on earth surface is exceeding the content of nanoparticles. There are various reports about the difference between the properties of micro and nanoparticles [48] mainly as the results of their altered molecular or atomic structures. Nanoparticles are a significant part of the atmospheric pollution, and recently, main components of numerous manufacturing products. Various methods have been introduced for nanoparticle manufacturing containing chemical precipitation, gas condensation, attrition, ion implantation, hydrothermal, and pyrolysis and synthesis [49]. The production and application of nanoparticles have resulted in advancing a branch in science known as nanotechnology. Nowadays, nanoparticles are artificially created from either solid or liquid materials containing metals, dielectrics, and semiconductors such as Se. Among different fields of science, nanoparticles have been commonly used and tested in agriculture [9]. However, studies on the effect of Se nanoparticles, and even its normal form, or Se microparticles, have rarely been studied on different plants and conditions.
Figure 6: A portrait comparison between different size of atom along with nano and micro particles
Even though Se, as a beneficial element in plant, has important roles in plant metabolisms and responses to different environmental conditions [50], and its availability in human and animal dietary obtaining by consumption of plant products is substantial, there is a lack of comprehensive reports and studies showing, and explaining, the different faces of this element in plants.
4.2 Regulation of Antioxidant Defense Mechanism under Abiotic Stress
Although it has not been verified that Se is required as an essential element in plants, numerous studies confirmed that its low concentrations in plant organelles have beneficial effects on plants. Selenium mainly contributes to overall plant growth and development under both normal and stress conditions. Several reports have shown that Se is more important in plants under abiotic stress in comparison to normal conditions [51,52].
According to some references, about 50% or more of the annual yield reduction of crops is due to the influence of abiotic stresses [53]. Subsequently, coping with and managing the negative effects of abiotic stresses have been amongst the greatest challenges for plant scientists within the last decades. According to numerous recent studies [54], Se is able to get involved in stress mechanisms and responses of plants under abiotic stress conditions. These studies verified that Se is able to increase the resistance of plants to various abiotic stresses (Table 2) such as excessive and deficient levels of nutritional elements, salinity, drought, etc. Iqbal et al. [55] confirmed that Se has the ability of enhancing the drought stress by regulating the water status in the cells and organelles of plants. Additionally, it has been stated that the main effect of Se under abiotic stresses is to get involved in ROS generation and detoxification pathways causing higher resistance of plant to oxidative stress triggered by abiotic stresses. Selenium can be absorbed by plant roots via sulfur transporters, as the result of its similarity to S, and then is transferred to the leaves where the photosynthetic compartments are the main sources of ROS generation (Fig. 7). There are still high demands to study the impacts of Se exogenous application on plants under abiotic stresses, i.e., nutrient deficiencies and toxicity, ozone stress, flooding stress, freezing stress, along with low light intensity to indicate the precise mechanism of plant responses.
Figure 7: A simple picturing of the overall pathway of selenium in plants from the roots to the leaves
Previous studies such as Zhou et al. [51] confirmed the association of GPX activity with Se concentration and removal of H2O2 from the plant organelles; later on, several studies verified the correlation of Se with the overall antioxidant capacity of the plants and higher antioxidant defense potential in plants under abiotic stresses [11]. In the study of Hasanuzzaman et al. [65], treating rapeseed plants with Se, therefore enhancing the levels of Se in the plants, clearly led to increase both the GSH activity and its available levels in this species. Banerjee et al. [66] demonstrated that under heavy metal toxicity in rye grass, Se was able to mediate the detoxification of superoxide radicals in cooperation with SOD. It has also been revealed that GSH is able to cooperate in regulating the levels of methylglyoxal (MG) and enhances the oxidative stress tolerance of plants. Glyoxalase pathways are involved in tolerance mechanisms of plants under abiotic stresses mainly by detoxification of MG that causes oxidative stress. In this process, Glyoxalase I (Gly I) and II (Gly II) enzymes are involved, and GSH assists the Gly I enzyme to detoxify the MG [67].
The Se contents in plants differs between various species. This content is directly associated with the capacity and ability of the plant species in both uptaking and accumulating the Se from the soil, and with the Se content along with the presence/absence and the level of other elements in the soil where the plant has developed [43]. Therefore, there are competition and some cooperation between different elements with Se that might lead to nutritional imbalances and oxidative stress in plants. Furthermore, based on plant species and its ability to use and maintain the content of Se in cells (Fig. 8), higher levels of this elements than those that are required for a normal growth and development can lead to Se toxification and occurrence of oxidative stress.
Figure 8: Different classes of plants regarding the accumulation of selenium in their tissue (data from Gupta et al. [68])
Selenium is chemically similar to S and can be metabolized by S metabolic pathways. Several studies such as those of Pilon-Smits et al. [69] and White et al. [70] related to Arabidopsis thaliana have indicated that the numerous genes which are involved in S assimilation and uptake may also be accountable for the accumulation of Se. Therefore, the excessive content of Se can result in S deficiency or Se toxification. Under excessive Se conditions, content of phytohormones such as salicylic acid, jasmonic acid, and ethylene that are playing a significant role in Se tolerance is enhanced by signaling pathways related to ROS. Moreover, in the study of Mackowiak et al. [71] application of S fertilizer reduced Se uptake by some plants like wheat grass and alfalfa. The content of soil organic matter and microorganism activity in soil are significantly enhanced under S fertilizer application, while the soil pH decreases under such condition. Higher content of organic matter and microorganism activity in the soil, and lowering of the pH, can result in the increase of Se fixation in soil [72]. On the contrary, Govasmark et al. [73] and Teixeira et al. [74] verified that the N fertilization is able to increase the uptake of Se by the root system and change its translocation and accumulation in wheat plants under low Se conditions. On the flip side, application of N stimulates S metabolism and accumulation through increasing the content and activity of O-acetyl serine, which is the main regulator in S metabolism via cysteine synthesis mechanism in higher plants. Since Se and S are highly similar and they use the parallel metabolic pathways, N application is also positive effective in Se absorption and metabolizing it into selenoproteins. Furthermore, N fertilizers mostly are available in nitrate-N (NO3-N) and NO3− forms that are able to displace the Se anions from the soil surface particles resulting in a SeO42− concentration increase in soil solution and therefore promoting Se uptake in roots [75].
Arsenic (As), as a heavy metal, is a highly toxic pollutant in the environment and its excessive content than normal in the soil causes abiotic stress leading to oxidative stress in many plants [76]. An antagonistic relationship between As and Se has been reported that is mainly depending upon their chemical forms, their content in soil, and the plant genetic background. In the periodic table, As and Se are located relatively close to each other, and they have parallel chemical properties. Subsequently, Se can interfere in As uptake and transportation to the root using different pathways. Different studies confirmed that Se can compete with As in absorption by the root via different transportation mechanisms and decrease the over As in plants leading to lower heavy metal stress. In addition, Hu et al. [77] and Moulick et al. [78] reported that Se (IV) is able to significantly prevent As(III) and As(V) transport from root to shoot in rice. According to Zhou et al. [51], Se is able to decrease As transportation from soil to root and restrain its transfer from root to shoot, hindering its localization into the grain in rice resulting in a lower influence of heavy metal stress and plant survival (Fig. 9). There are two main reasons that Se limits the As transportation: (1) As(V) that is absorbed by plants can be reduced to As(III) in root cells. In Lewis acid-base reaction, As(III) can be chelated via glutathione (GSH) activity, that is directly under the influence of Se, or by means of phytochelatins (PCs). The chelated As(III) are ultimately stored in the vacuole organelles of the plant roots and they cannot be transported to the plant shoot anymore [79], (2) Se regulates As transportation pathway in the stem node phloem and constraints the transportation of As between the plant cells and tissues. Therefore, As is highly accumulated in the vascular bundle stem node. In addition, the stem node has a central role in As distribution and translocation from xylem to phloem [80]. This mechanism might be related to the development of apoplastic barriers in the endodermis by Se leading to even more restraining of the heavy metals translocation into the xylem and shoots [81].
Figure 9: The mechanism of translocation of selenium in plants and their main pathways
5 Molecular Biology of Selenium and Nano-Selenium in Plants under Oxidative Stress
Although there is a lack of studies related to the molecular mechanism of Se uptake and translocation under stress conditions, some researches on tobacco (Nicotiana tabacum) and rice (Oryza sativa) showed that inorganic phosphate (Pi) transporters might be capable of transporting selenite in the root [82]. In these studies, specific Pi transporters overexpression resulted in significant selenite uptake by plants from the substrate. There are some reports that the aquaporin family lactic acid channel NIP2;1 is capable of taking up selenite in plants [83,84]. This result is significant because selenite is the predominant form of available Se for most of the plants such as rice that are cultivated in the anoxic soil types [84]. In addition, there other organic forms and compounds of Se available in the agricultural soils in high proportions, one of which is selenoamino acids [85]. Plants are capable of taking up the amino acids selenomethionine (SeMet) and selenocysteine (SeCys) from the soil [86], possibly by means of amino acid transporters in roots. However, these compounds are the main pathway of absorbing Se via their different forms containing amino acids of methionine (Met) and cysteine (Cys) [44,87]. Studies in A. thaliana showed that amino acid permease (AAP) are compounds with specific roles in plants that might mediate the absorption of Met and Cys and influence the uptake of Se in root [88,89]. van Hoewyk et al. [90] illustrated that the expression of AtCpNifS in root may affect the binding and releasing of Se and Cys. It has been shown that overexpression of this gene is directly influencing the Se tolerance in plant cells [84].
It has been predicted that there are over 1600 transcriptional factors (TF) in Arabidopsis genome; a highly number of them are directly and indirectly involved in abiotic and oxidative stresses [84]. Under abiotic stresses, the proteins such as EIN1, EIN2 and EIN3 that are involved in the pathways of ethylene signaling are able to induce an important TF called ERF. ERF is a super family that contains many stresses responsive factors, around 120 TF, such as AP-binding elements, cold-binding factor (CBF), and dehydration responsive element binding-protein (DREB) [88]. Among these TFs, over 27 ERFs showed to get influenced by the treatment of the plant by selenate [91]. Most of these ERF genes were upregulated by maximum 15-fold (in AtERF92/ERF1) as the result of Se treatment. According to previous studies, Cbf1 is one of the significant ERFs directly involved in the drought, salt, cold, and oxidative stress tolerance in plants and its overexpression significantly increases the plant tolerance to these stresses [92]. Accordingly, by growing plants under normal availability of Se, the Cbf1 gene is upregulated, mostly in root tissues [93]. In Arabidopsis thaliana, Se treatments upregulated some other TFs than ERFs containing heat shock factor (HSF) TF families, Zinc transporters, WRKY, and Myb; all of which are responsive to abiotic stress conditions [91]. WRKY are important TFs that are also responding to ethylene confirming the interaction between Se levels in plants and the ethylene activity [94]. Furthermore, HSFA2, HSFA6a, and Zat12 that are involved in H2O2 generation and detoxification were positively upregulated by Se which clearly implies the significant roles of Se in oxidative stress and ROS signaling [44,87].
As the result of similarity between S and Se elements, under high levels of S in the plant cells, Se would show a low or no effects on the plants under both normal and abiotic stress conditions due to the competition between these two elements. Therefore, the plant applies some mechanisms to decrease the content of S and increase the uptake of Se in plants [95]. It has been reported that Se can upregulate the pathways active in S assimilation and regulate the abundance of its metabolites [96]. Treating the plants with selenate led to increase the encoding of SULTR1;1 and SULTR2;1 that are active sulfate transporters in roots resulting in a reduction of S uptake and transportation [89]. Additionally, these sulfate transporters are able to transport the S compounds into roots from other tissues and store them in root vacuoles. Adenosine phosphosulfate reductase (APR1, APR2 and APR3) genes and APS3 that are active in sulfur assimilation are also upregulated by available Se compounds in plant tissues [97]. One other mechanism of plants to regulate the S levels under Se availability is through the synthesizing the S-rich glucosinolates that assimilates the sulfur molecules [97]. There are important genes involved in the mechanisms such as MAM1 and BRAT4, as aminotransferase factors, that can contribute to glucosinolate biosynthesis via methionine-derived pathways [98].
6 Selenium Crosstalk with Other Signaling Molecules in Modulating Antioxidant Defense during Plant Response to Oxidative Stress
Selenium uptake and translocation in plant are largely influenced by the genetic background of plants. Se uptake could vary between plants of different species, or even between plant genotypes within the same species. There are also other factors that affect the Se uptake in plants like the plant physiological condition, its developmental stage, the activity levels of transporters in the membrane, physicochemical conditions of the soil and available content of other elements, and Se form and concentration in the soil [99].
In nature, there are different forms of Se available as (1) inorganic containing selenate (SeO42−), selenite (SeO32−), selenide (Se2−), elemental Se and (2) organic forms such as SeCys and SeMet (Fig. 10) [68,42]. Selenium predominant bioavailable form in agricultural soils is the selenate (SeO42−) which is also soluble in water [100]. Selenite and selenate are transported by different mechanisms in roots. The phosphate transporters are capable of transporting the selenite into the root tissue while the sulfate transporters are able to transport selenate molecules [101,102]. These available transporters are basically affected by the status of nutritional elements both within the plant and in the soil. In a competition between Se and S regarding the sulfate transporters, the elements that have higher concentrations in the soil will win, and the transporters will show high selectivity toward this element. There are two main sulfate transporters as SULTR1 and SULTR1;2 (Fig. 10) in Arabidopsis thaliana that are capable of transporting the selenate into the plant tissues [103]. Barberon et al. [104] reported that for selenate uptake to root tissue, SULTR1;2 is the predominant transporter. El Kassis et al. [105] illustrated that both sulfur starvation and phosphorous deficiency result in higher uptake of overall Se by the root system. On the other hand, in the different researches the transportation of Se into plant by both passive diffusion and active mechanisms has been reported.
Figure 10: The mechanism of selenium transportation under oxidative stress in plants
In addition, it has been verified that the different Se forms are also varied in terms of their mobilization within and between the tissues and cells and synthesizing the Se-compounds. The translocation of Se compounds from the root system to the shoot tissues is directly affected by Se loading rate in the root xylem and transpiration rate of the plant [106]. In the study of Kikkert et al. [101], the mobility of Se compounds within the plant was considered, and the results showed that selenate has the highest rate of mobility in wheat and canola plants. In this study selenite and SeCys showed roughly equal rate of mobility inside the plant tissues while their rate was lower than that of SeMet.
The main pathway of translocating the Se from the root cells to shoot and leaf tissues is through xylem. The rate of translocation in this organelle depends upon the provided form of Se from the soil. Se(VI) is the most abundant form of Se that can be easily translocated via the xylem pathway and then distributed among the phloem organs and organelles [107]. In addition to the Se(VI) form in xylem, other forms and compounds of Se such as MeSeCys, SeMet and SeOMet are available in lower concentrations that normally are assimilated and remain in root cells. AtALMT12, and some other members of the transporter family called ALMT, are acting in loading the Se(VI) form into the xylem sap. The available Se(VI) in the xylem may be transported to the phloem or, by the activity of SULTR transporter, placed in the mesophyll cells of roots and assimilated in the vacuole organelles. AtSULTR4;1 and AtSLUTR4;2 have been reported to be main factors responsible for efflux transporting of Se(VI) to the vacuoles [86]. Meanwhile, AtSULTR2;2 and AtSULTR1;3 have been suggested to be the main factors of translocating the Se(VI) into the phloem because their upregulation and higher encoding in Arabidopsis have increased the rate of translocation of Se(VI) from the xylem to phloem. After storing the Se(VI) in the leaves, they would reduce it into the Se(IV) form and will be then incorporated to the selenium organic compounds Se-methyl selenocysteine (MeSeCys) and SeMet, and redistributed to the tissues that might require them. Under oxidative stress the organic Se compounds are able to act as signal transduction agents and assess in regulating the responsive genes to stress conditions. In addition, some of the metabolized Se compound can contribute to the tolerance mechanism of plants, such as increasing the efficiency of antioxidants, and provide higher tolerance to oxidative stress caused by abiotic stimuli.
7 Conclusion and Future Prospective
Abiotic stresses caused by harsh and uncontrolled environmental stresses can critically reduce the plant yield and production. As a result, improving the stress tolerance of plants against such stresses based on appropriate knowledge related to their basic and molecular impact must be taken as a priority subject in the area of plant science. In this paper different aspects of abiotic stresses leading to oxidative stress in conjunction with the effect of selenium, as a nutrition element, were illustrated. Under abiotic stress condition, the generation of oxygen free radicals increases and the steady state of oxygen radicals is messed up which in turn it causes indirect oxidative stress in plants; however, the application of Se as an exogenous supply might be able to assist the plants to cope with such detrimental condition. Selenium is able to get involved in both mechanisms of non-enzymatic and enzymatic antioxidant systems rooting to an increase in the suitability of antioxidant pathways in plants. In addition, the different possible pathways of Se to affect plant production at molecular and cellular levels were also clearly explained in this paper. Literature review indicates that there is still a lack of comprehensive studies related to the impacts of Se under stress conditions, and there is a high requirement for more clarification of the mechanisms involved in Se transpiring and transporting in addition to the identification of related genes and factors transporters that are active in such pathways. The probable competition between Se uptake and transportation with other elements, especially As and S as examples, along with the suitable content of Se in both plant and the soil as to constrain the possible occurrence of Se toxicity are also calling for more studies.
Funding Statement: The authors received no specific funding for this study.
Author Contributions: The main materials and subjects related to this study were collected by A.S-M. The main text of the current study was prepared by A.S-M and was reviewed by E.R.M.
Availability of Data and Materials: All data generated or analyzed during this study are included in this published article.
Ethics Approval: No human or animal tissue of subject has been used in this study. Any method or refence that are used in this study have mentioned in the regarding section.
Conflicts of Interest: The authors declare that they have no conflicts of interest to report regarding the present study.
References
1. Ahmad, P., Tripathi, D. K., Deshmukh, R., Pratap Singh, V., Corpas, F. J. (2019). Revisiting the role of ROS and RNS in plants under changing environment. Environmental and Experimental Botany, 161(1), 1–3. [Google Scholar]
2. Ul Mushtaq, N., Alghamdi, K. M., Saleem, S., Shajar, F., Tahir, I. et al. (2022). Selenate and selenite transporters in Proso millet: Genome extensive detection and expression studies under salt stress and selenium. Frontiers in Plant Science, 13, 4846–4863. https://doi.org/10.3389/fpls.2022.1060154 [Google Scholar] [PubMed] [CrossRef]
3. Saed-Moucheshi, A., Pessarakli, M., Mozafari, A. A., Sohrabi, F., Moradi, M. et al. (2021). Screening barley varieties tolerant to drought stress based on tolerant indices. Journal of Plant Nutrition, 47, 1–12. https://doi.org/10.1080/01904167.2021.1963773 [Google Scholar] [CrossRef]
4. Saed-Moucheshi, A., Shekoofa, A., Pessarakli, M. (2014). Reactive oxygen species (ROS) generation and detoxifying in plants. Journal of Plant Nutrition, 37(10), 1573–1585. [Google Scholar]
5. Rasool, A., Shah, W. H., Mushtaq, N. U., Saleem, S., Hakeem, K. R. et al. (2022). Amelioration of salinity induced damage in plants by selenium application: A review. South African Journal of Botany, 147(2), 98–105. [Google Scholar]
6. Saed-Moucheshi, A., Pessarakli, M., Mikhak, A., Ostovar, P., Ahamadi-Niaz, A. (2017). Investigative approaches associated with plausible chemical and biochemical markers for screening wheat genotypes under salinity stress. Journal of Plant Nutrition, 40, 2768–2784. [Google Scholar]
7. Saed-Moucheshi, A., Heidari, B., Zarei, M., Emam, Y., Pessarakli, M. (2013). Changes in antioxidant enzymes activity and physiological traits of wheat cultivars in response to arbuscular mycorrhizal symbiosis in different water regimes. Iran Agricultural Research, 31, 35–50. [Google Scholar]
8. Saed-Moocheshi, A., Shekoofa, A., Sadeghi, H., Pessarakli, M. (2014). Drought and salt stress mitigation by seed priming with KNO3 and urea in various maize hybrids: An experimental approach based on enhancing antioxidant responses. Journal of Plant Nutrition, 37, 674–689. [Google Scholar]
9. Mozafari, A. A., Havas, F., Ghaderi, N. (2018). Application of iron nanoparticles and salicylic acid in in vitro culture of strawberries (Fragaria × ananassa Duch.) to cope with drought stress. Plant Cell, Tissue and Organ Culture (PCTOC), 132, 511–523. [Google Scholar]
10. Jindo, K., Canellas, L. P., Albacete, A., Figueiredo dos Santos, L.,Frinhani Rocha, R. L. et al. (2020). Interaction between humic substances and plant hormones for phosphorous acquisition. Agronomy, 10, 640–663. [Google Scholar]
11. Dai, Z., Rizwan, M., Gao, F., Yuan, Y., Huang, H. et al. (2020). Nitric oxide alleviates selenium toxicity in rice by regulating antioxidation, selenium uptake, speciation and gene expression. Environmental Pollution, 257(1), 113540. [Google Scholar] [PubMed]
12. Perez-Millan, R., Alfosea-Simon, M., Zavala-Gonzalez, E. A., Camara-Zapata, J. M., Martinez-Nicolas, J. J. et al. (2021). The addition of selenium to the nutrient solution decreases cadmium toxicity in pepper plants grown under hydroponic conditions. Agronomy, 11(10), 1905. [Google Scholar]
13. Saed-Moucheshi, A., Pakniyat, H., Pirasteh-Anosheh, H., Azooz, M. (2014). Role of ROS as signaling molecules in plants. In: Ahmad, P. (Ed.Reactive oxygen species, antioxidant network and signaling in plants, pp. 585–626. New York, USA: Springer Publication. [Google Scholar]
14. Tabarzad, A., Ayoubi, B., Riasat, M., Saed-Moucheshi, A., Pessarakli, M. (2017). Perusing biochemical antioxidant enzymes as selection criteria under drought stress in wheat varieties. Journal of Plant Nutrition, 40(17), 2413–2420. [Google Scholar]
15. Riasat, M., Kiani, S., Saed-Mouchehsi, A., Pessarakli, M. (2019). Oxidant related biochemical traits are significant indices in triticale grain yield under drought stress condition. Journal of Plant Nutrition, 42(2), 111–126. [Google Scholar]
16. Saed-Moucheshi, A., Sohrabi, F., Fasihfar, E., Baniasadi, F., Riasat, M. et al. (2021). Superoxide dismutase (SOD) as a selection criterion for triticale grain yield under drought stress: A comprehensive study on genomics and expression profiling, bioinformatics, heritability, and phenotypic variability. BMC Plant Biology, 21(1), 1–19. [Google Scholar]
17. Berni, R., Luyckx, M., Xu, X., Legay, S., Sergeant, K. et al. (2019). Reactive oxygen species and heavy metal stress in plants: Impact on the cell wall and secondary metabolism. Environmental and Experimental Botany, 161(1), 98–106. [Google Scholar]
18. Zahedi, M. B., Razi, H., Saed-Moucheshi, A. (2016). Evaluation of antioxidant enzymes, lipid peroxidation and proline content as selection criteria for grain yield under water deficit stress in barley. Journal of Applied Biological Sciences, 10, 34–51. [Google Scholar]
19. Riasat, M., Jafari, A. A., Saed-Mouchehsi, A. (2019). Effect of drought stress levels on seedling morpho-physiological traits of alfalfa (Medicago sativa) populations grown in glasshouse. Journal of Rangeland Science, 12, 201–216. [Google Scholar]
20. Yalcinkaya, T., Uzilday, B., Ozgur, R., Turkan, I., Mano, J. (2019). Lipid peroxidation-derived reactive carbonyl species (RCSTheir interaction with ROS and cellular redox during environmental stresses. Environmental and Experimental Botany, 165(2), 139–149. [Google Scholar]
21. Sarker, U., Oba, S. (2018). Catalase, superoxide dismutase and ascorbate-glutathione cycle enzymes confer drought tolerance of Amaranthus tricolor. Scientific Reports, 8(1), 1–12. [Google Scholar]
22. Karuppanapandian, T., Moon, J. C., Kim, C., Manoharan, K., Kim, W. (2011). Reactive oxygen species in plants: Their generation, signal transduction, and scavenging mechanisms. Australian Journal of Crop Science, 5, 709–725. [Google Scholar]
23. Racchi, M. L. (2013). Antioxidant defenses in plants with attention to Prunus and Citrus spp. Antioxidants, 2, 340–369. [Google Scholar] [PubMed]
24. Gillespie, K. M., Rogers, A., Ainsworth, E. A. (2011). Growth at elevated ozone or elevated carbon dioxide concentration alters antioxidant capacity and response to acute oxidative stress in soybean (Glycine max). Journal of Experimental Botany, 62, 2667–2678. [Google Scholar] [PubMed]
25. Ben Amor, N., Jiménez, A., Boudabbous, M., Sevilla, F., Abdelly, C. (2020). Chloroplast implication in the tolerance to salinity of the halophyte Cakile maritima. Russian Journal of Plant Physiology, 67, 507–514. [Google Scholar]
26. Samis, K., Bowley, S., McKersie, B. (2002). Pyramiding Mn-superoxide dismutase transgenes to improve persistence and biomass production in alfalfa. Journal of Experimental Botany, 53, 1343–1350. [Google Scholar] [PubMed]
27. Anjum, N. A., Ahmad, I., Mohmood, I., Pacheco, M., Duarte, A. C. et al. (2012). Modulation of glutathione and its related enzymes in plants’ responses to toxic metals and metalloids—A review. Environmental and Experimental Botany, 75, 307–324. [Google Scholar]
28. Hasanuzzaman, M., Nahar, K., Khan, M. I. R., Al Mahmud, J., Alam, M. M. et al. (2020). Regulation of reactive oxygen species metabolism and glyoxalase systems by exogenous osmolytes confers thermotolerance in Brassica napus. Gesunde Pflanzen, 72, 3–16. [Google Scholar]
29. Teotia, S., Singh, D. (2014). Oxidative stress in plants and its management. In: Approaches to plant stress and their management, pp. 227–253. New York, USA: Springer. https://doi.org/10.1007/978-81-322-1620-9_13 [Google Scholar] [CrossRef]
30. Kumar, R. R., Goswami, S., Singh, K., Rai, G. K., Rai, R. D. (2013). Modulation of redox signal transduction in plant system through induction of free radical/ROS scavenging redox-sensitive enzymes and metabolites. Australian Journal of Crop Science, 7, 1744–1751. [Google Scholar]
31. Kumar, A., Prasad, A., Pospíšil, P. (2020). Formation of α-tocopherol hydroperoxide and α-tocopheroxyl radical: Relevance for photooxidative stress in Arabidopsis. Scientific Reports, 10, 1–12. [Google Scholar]
32. Xiao, M., Li, Z., Zhu, L., Wang, J., Zhang, B. et al. (2021). The multiple roles of ascorbate in the abiotic stress response of plants: Antioxidant, cofactor, and regulator. Frontiers in Plant Science, 12, 598173. [Google Scholar] [PubMed]
33. Wang, Y., Zhao, H., Qin, H., Li, Z., Liu, H. et al. (2018). The synthesis of ascorbic acid in rice roots plays an important role in the salt tolerance of rice by scavenging ROS. International Journal of Molecular Sciences, 19(11), 3347. [Google Scholar] [PubMed]
34. Song, X. S., Hu, W. H., Mao, W. H., Ogweno, J. O., Zhou, Y. H. et al. (2005). Response of ascorbate peroxidase isoenzymes and ascorbate regeneration system to abiotic stresses in Cucumis sativus L. Plant Physiology and Biochemistry, 43(12), 1082–1088. [Google Scholar] [PubMed]
35. Sharma, P., Jha, A. B., Dubey, R. S., Pessarakli, M. (2012). Reactive oxygen species, oxidative damage, and antioxidative defense mechanism in plants under stressful conditions. Journal of Botany, 2012(1), 1–26. [Google Scholar]
36. Munné-Bosch, S., Weiler, E. W., Alegre, L., Müller, M., Düchting, P. et al. (2007). α-tocopherol may influence cellular signaling by modulating jasmonic acid levels in plants. Planta, 225(3), 681–691. [Google Scholar]
37. Kalia, R., Sareen, S., Nagpal, A., Katnoria, J., Bhardwaj, R. (2017). ROS-induced transcription factors during oxidative stress in plants: A tabulated review. In: Reactive oxygen species and antioxidant systems in plants: role and regulation under abiotic stress, pp. 129–158. New York, USA: Springer. https://doi.org/10.1007/978-981-10-5254-5_6 [Google Scholar] [CrossRef]
38. Pisoschi, A. M., Pop, A., Iordache, F., Stanca, L., Predoi, G. et al. (2021). Oxidative stress mitigation by antioxidants--An overview on their chemistry and influences on health status. European Journal of Medicinal Chemistry, 209(2015), 112891. [Google Scholar] [PubMed]
39. Fan, X., Zhou, X., Chen, H., Tang, M., Xie, X. (2021). Cross-talks between macro-and micronutrient uptake and signaling in plants. Frontiers in Plant Science, 12, 663477. [Google Scholar] [PubMed]
40. Marschner, H. (2011). Marschner’s mineral nutrition of higher plants. USA, NY: Academic Press. [Google Scholar]
41. Ligaba-Osena, A., Guo, W., Choi, S. C., Limmer, M. A., Seyfferth, A. L. et al. (2020). Silicon enhances biomass and grain yield in an ancient crop tef [Eragrostis tef (Zucc.) Trotter]. Frontiers in Plant Science, 11, 608503. [Google Scholar] [PubMed]
42. Ryant, P., Antošovský, J., Adam, V., Ducsay, L., Škarpa, P. et al. (2020). The importance of selenium in fruit nutrition. In: Fruit crops, pp. 241–254. New York, USA: Elsevier. https://doi.org/10.1016/B978-0-12-818732-6.00018-6 [Google Scholar] [CrossRef]
43. Bodnar, M., Konieczka, P., Namiesnik, J. (2012). The properties, functions, and use of selenium compounds in living organisms. Journal of Environmental Science and Health, Part C, 30(3), 225–252. [Google Scholar]
44. El-Ramady, H. R., Domokos-Szabolcsy, É., Abdalla, N. A., Alshaal, T. A., Shalaby, T. A. et al. (2014). Selenium and nano-selenium in agroecosystems. Environmental Chemistry Letters, 12(4), 495–510. [Google Scholar]
45. Attia, T. M. S., Elsheery, N. I. (2020). Nanomaterials: Scope, applications, and challenges in agriculture and soil reclamation. In: Sustainable agriculture reviews, vol. 41, pp. 1–39. New York, USA: Springer. [Google Scholar]
46. Dolai, J., Mandal, K., Jana, N. R. (2021). Nanoparticle size effects in biomedical applications. ACS Applied Nano Materials, 4(7), 6471–6496. [Google Scholar]
47. Levin, S., Sundén, H. (2021). Nanofluidics as a tool for parallelized single nanoparticle characterization: Fluorescence single nanoparticle catalysis and size determination through 1D Brownian motion. Nature Communications, 10, 4426–4439. https://www.nature.com/articles/s41467-019-12458-1 [Google Scholar]
48. Ma, X., Geiser-Lee, J., Deng, Y., Kolmakov, A. (2010). Interactions between engineered nanoparticles (ENPs) and plants: Phytotoxicity, uptake and accumulation. Science of the Total Environment, 408, 3053–3061. [Google Scholar] [PubMed]
49. Jamkhande, P. G., Ghule, N. W., Bamer, A. H., Kalaskar, M. G. (2019). Metal nanoparticles synthesis: An overview on methods of preparation, advantages and disadvantages, and applications. Journal of Drug Delivery Science and Technology, 53, 101174. [Google Scholar]
50. Luo, H., Xing, P., Liu, J., Pan, S., Tang, X. et al. (2021). Selenium improved antioxidant response and photosynthesis in fragrant rice (Oryza sativa L.) seedlings during drought stress. Physiology and Molecular Biology of Plants, 40, 1–10. [Google Scholar]
51. Zhou, X., Gao, A., Zhang, C., Xu, W., Shi, X. (2017). Exogenous selenium alleviates mercury toxicity by preventing oxidative stress in rice (Oryza sativa) seedlings. International Journal of Agriculture and Biology, 19, 1593–1600. [Google Scholar]
52. El-Badri, A. M., Batool, M., Wang, C., Hashem, A. M., Tabl, K. M. et al. (2021). Selenium and zinc oxide nanoparticles modulate the molecular and morpho-physiological processes during seed germination of Brassica napus under salt stress. Ecotoxicology and Environmental Safety, 225, 1126–1145. [Google Scholar]
53. Yadav, S., Modi, P., Dave, A., Vijapura, A., Patel, D. et al. (2020). Effect of abiotic stress on crops. In: Sustainable crop production, vol. 1, pp. 340–357. New York, USA: IntechOpen. https://doi.org/10.5772/intechopen.88434 [Google Scholar]
54. Hummel, M., Hallahan, B. F., Brychkova, G., Ramirez-Villegas, J., Guwela, V. et al. (2018). Reduction in nutritional quality and growing area suitability of common bean under climate change induced drought stress in Africa. Scientific Reports, 8, 1–11. [Google Scholar]
55. Iqbal, M., Hussain, I., Liaqat, H., Ashraf, M. A., Rasheed, R. et al. (2015). Exogenously applied selenium reduces oxidative stress and induces heat tolerance in spring wheat. Plant Physiology and Biochemistry, 94, 95–103. [Google Scholar] [PubMed]
56. Ahmad, Z., Waraich, E. A., Barutcular, C., Alharby, H., Bamagoos, A. et al. (2020). Enhancing drought tolerance in Camelina sativa L. and canola (Brassica napus L.) through application of selenium. Pakistan Journal of Botany, 52, 1927–1939. [Google Scholar]
57. Ikram, M., Raja, N., Javed, B., Mashwani, Z., Hussain, M. et al. (2020). Foliar applications of bio-fabricated selenium nanoparticles to improve the growth of wheat plants under drought stress. Green Processing and Synthesis, 9, 706–714. [Google Scholar]
58. Rady, M. M., Belal, H. E. E., Gadallah, F. M., Semida, W. M. (2020). Selenium application in two methods promotes drought tolerance in Solanum lycopersicum plant by inducing the antioxidant defense system. Scientia Horticulturae, 266, 109–190. [Google Scholar]
59. Desoky, E. S. M., Merwad, A. R., Abo El-Maati, M. F., Mansour, E., Arnaout, S. M. A. I. et al. (2021). Physiological and biochemical mechanisms of exogenously applied selenium for alleviating destructive impacts induced by salinity stress in bread wheat. Agronomy, 11(5), 926. [Google Scholar]
60. Badawy, S. A., Zayed, B. A., Bassiouni, S., Mahdi, A. H. A., Majrashi, A. et al. (2021). Influence of nano silicon and nano selenium on root characters, growth, ion selectivity, yield, and yield components of rice (Oryza sativa L.) under salinity conditions. Plants, 10(8), 1657–1678. [Google Scholar] [PubMed]
61. Karimi, R., Ghabooli, M., Rahimi, J., Amerian, M. (2020). Effects of foliar selenium application on some physiological and phytochemical parameters of Vitis vinifera L. cv. Sultana under salt stress. Journal of Plant Nutrition, 43, 2226–2242. [Google Scholar]
62. Shalaby, T. A., Abd-Alkarim, E., El-Aidy, F., Hamed, E. S., Sharaf-Eldin, M. et al. (2021). Nano-selenium, silicon and H2O2 boost growth and productivity of cucumber under combined salinity and heat stress. Ecotoxicology and Environmental Safety, 212(31), 1119–1132. [Google Scholar]
63. Sardar, R., Ahmed, S., Shah, A. A., Yasin, N. A. (2022). Selenium nanoparticles reduced cadmium uptake, regulated nutritional homeostasis and antioxidative system in Coriandrum sativum grown in cadmium toxic conditions. Chemosphere, 287(26), 1323–1332. [Google Scholar]
64. Das, S., Majumder, B., Biswas, A. K. (2021). Selenium alleviates arsenic induced stress by modulating growth, oxidative stress, antioxidant defense and thiol metabolism in rice seedlings. International Journal of Phytoremediation, 36, 1–15. [Google Scholar]
65. Hasanuzzaman, M., Hossain, M. A., Fujita, M. (2012). Exogenous selenium pretreatment protects rapeseed seedlings from cadmium-induced oxidative stress by upregulating antioxidant defense and methylglyoxal detoxification systems. Biological Trace Element Research, 149(2), 248–261. [Google Scholar] [PubMed]
66. Banerjee, A., Roychoudhury, A. (2019). Role of selenium in plants against abiotic stresses: Phenological and molecular aspects. Molecular Plant Abiotic Stress: Biology and Biotechnology, 10, 123–133. [Google Scholar]
67. Ghosh, A., Kushwaha, H. R., Hasan, M. R., Pareek, A., Sopory, S. K. et al. (2016). Presence of unique glyoxalase III proteins in plants indicates the existence of shorter route for methylglyoxal detoxification. Scientific Reports, 6(1), 1–15. [Google Scholar]
68. Gupta, M., Gupta, S. (2017). An overview of selenium uptake, metabolism, and toxicity in plants. Frontiers in Plant Science, 7, 2074. [Google Scholar] [PubMed]
69. Pilon-Smits, E. A. H., LeDuc, D. L. (2009). Phytoremediation of selenium using transgenic plants. Current Opinion in Biotechnology, 20(2), 207–212. [Google Scholar] [PubMed]
70. White, P. J., Bowen, H. C., Parmaguru, P., Fritz, M., Spracklen, W. P. et al. (2004). Interactions between selenium and sulphur nutrition in Arabidopsis thaliana. Journal of Experimental Botany, 55(404), 1927–1937. [Google Scholar] [PubMed]
71. Mackowiak, C. L., Amacher, M. C. (2008). Soil sulfur amendments suppress selenium uptake by alfalfa and western wheatgrass. Journal of Environmental Quality, 37(3), 772–779. [Google Scholar] [PubMed]
72. Liu, N., Wang, M., Zhou, F., Zhai, H., Qi, M. et al. (2021). Selenium bioavailability in soil-wheat system and its dominant influential factors: A field study in Shaanxi Province. China Science of the Total Environment, 770(8), 144–166. [Google Scholar]
73. Govasmark, E., Singh, B. R., MacLeod, J. A., Grimmett, M. G. (2008). Selenium concentration in spring wheat and leaching water as influenced by application times of selenium and nitrogen. Journal of Plant Nutrition, 31(2), 193–203. [Google Scholar]
74. Teixeira, L. S., Pimenta, T. M., Brito, F. A. L., Malheiros, R. S. P., Arruda, R. S. et al. (2021). Selenium uptake and grain nutritional quality are affected by nitrogen fertilization in rice (Oryza sativa L.). Plant Cell Reports, 40(5), 871–880. [Google Scholar] [PubMed]
75. Nothstein, A. K. (2016). Selenium transfer between kaolinite or goethite surfaces, nutrient solution and Oryza sativa. New York, USA: KIT Scientific Publishing. [Google Scholar]
76. Ashraf, S., Ali, Q., Zahir, Z. A., Ashraf, S., Asghar, H. N. (2019). Phytoremediation: Environmentally sustainable way for reclamation of heavy metal polluted soils. Ecotoxicology and Environmental Safety, 174(13), 714–727. [Google Scholar] [PubMed]
77. Hu, Y., Duan, G. L., Huang, Y. Z., Liu, Y. X., Sun, G. X. (2014). Interactive effects of different inorganic As and Se species on their uptake and translocation by rice (Oryza sativa L.) seedlings. Environmental Science and Pollution Research, 21(5), 3955–3962. [Google Scholar] [PubMed]
78. Moulick, D., Samanta, S., Sarkar, S., Mukherjee, A., Pattnaik, B. K. et al. (2021). Arsenic contamination, impact and mitigation strategies in rice agro-environment: An inclusive insight. Science of the Total Environment, 800(2), 149477. [Google Scholar] [PubMed]
79. Yang, X., Feng, Y., He, Z., Stoffella, P. J. (2005). Molecular mechanisms of heavy metal hyperaccumulation and phytoremediation. Journal of Trace Elements in Medicine and Biology, 18(4), 339–353. [Google Scholar] [PubMed]
80. Wan, Y., Camara, A. Y., Huang, Q., Yu, Y., Wang, Q. et al. (2018). Arsenic uptake and accumulation in rice (Oryza sativa L.) with selenite fertilization and water management. Ecotoxicology and Environmental Safety, 156(1), 67–74. [Google Scholar] [PubMed]
81. Chen, X., Li, Y., Jiang, L., Hu, B., Wang, L. et al. (2021). Uptake, accumulation, and translocation mechanisms of steroid estrogens in plants. Science of the Total Environment, 753, 141979. [Google Scholar] [PubMed]
82. Song, Z., Shao, H., Huang, H., Shen, Y., Wang, L. et al. (2017). Overexpression of the phosphate transporter gene OsPT8 improves the Pi and selenium contents in Nicotiana tabacum. Environmental and Experimental Botany, 137(3), 158–165. [Google Scholar]
83. Zhao, X. Q., Mitani, N., Yamaji, N., Shen, R. F., Ma, J. F. (2010). Involvement of silicon influx transporter OsNIP2;1 in selenite uptake in rice. Plant Physiology, 153(4), 1871–1877. [Google Scholar] [PubMed]
84. Trippe Iii, R. C., Pilon-Smits, E. A. H. (2021). Selenium transport and metabolism in plants: Phytoremediation and biofortification implications. Journal of Hazardous Materials, 404(285), 124–178. [Google Scholar]
85. El Mehdawi, A. F., Lindblom, S. D., Cappa, J. J., Fakra, S. C., Pilon-Smits, E. A. H. (2015). Do selenium hyperaccumulators affect selenium speciation in neighboring plants and soil? An X-ray microprobe analysis. International Journal of Phytoremediation, 17(8), 753–765. [Google Scholar] [PubMed]
86. Zhou, X., Yang, J., Kronzucker, H. J., Shi, W. (2020). Selenium biofortification and interaction with other elements in plants: A review. Frontiers in Plant Science, 11, 586421. [Google Scholar] [PubMed]
87. Adebayo, A. H., Yakubu, O. F., Bakare-Akpata, O. (2020). Uptake, metabolism and toxicity of selenium in tropical plants. In: Importance of selenium in the environment and human health, pp. 234–251. New York, USA: IntechOpen. [Google Scholar]
88. Schiavon, M., Santoro, V. (2022). Manipulation of selenium metabolism in plants for tolerance and accumulation. In: Selenium and nano-selenium in environmental stress management and crop quality improvement, pp. 325–340. New York, USA: Springer. [Google Scholar]
89. Szőllősi, R., Molnár, Á., Oláh, D., Kondak, S., Kolbert, Z. (2022). Uptake and metabolism of selenium in plants: Recent progress and future perspectives. In: Selenium and nano-selenium in environmental stress management and crop quality improvement, pp. 79–90. [Google Scholar]
90. van Hoewyk, D., Garifullina, G. F., Ackley, A. R., Abdel-Ghany, S. E., Marcus, M. A. et al. (2005). Overexpression of AtCpNifS enhances selenium tolerance and accumulation in Arabidopsis. Plant Physiology, 139(3), 1518–1528. [Google Scholar] [PubMed]
91. van Hoewyk, D., Takahashi, H., Inoue, E., Hess, A., Tamaoki, M. et al. (2008). Transcriptome analyses give insights into selenium-stress responses and selenium tolerance mechanisms in Arabidopsis. Physiologia Plantarum, 132, 236–253. [Google Scholar] [PubMed]
92. Lv, K., Li, J., Zhao, K., Chen, S., Nie, J. et al. (2020). Overexpression of an AP2/ERF family gene, BpERF13, in birch enhances cold tolerance through upregulating CBF genes and mitigating reactive oxygen species. Plant Science, 292, 110375. [Google Scholar] [PubMed]
93. Lv, C., Li, F., Ai, X., Bi, H. (2022). H2O2 participates in ABA regulation of grafting-induced chilling tolerance in cucumber. Plant Cell Reports, 41(4), 1115–1130. [Google Scholar] [PubMed]
94. Sharif, R., Liu, P., Wang, D., Jin, Z., Uzair, U. et al. (2021). Genome-wide characterisation and expression analysis of cellulose synthase genes superfamily under various environmental stresses in Cucumis sativus L. New Zealand Journal of Crop and Horticultural Science, 49(2–3), 127–150. [Google Scholar]
95. Feng, R., Zhao, P., Zhu, Y., Yang, J., Wei, X. et al. (2021). Application of inorganic selenium to reduce accumulation and toxicity of heavy metals (metalloids) in plants: The main mechanisms, concerns, and risks. Science of the Total Environment, 771(6), 144776. [Google Scholar] [PubMed]
96. Zhang, N. N., Zou, H., Lin, X. Y., Pan, Q., Zhang, W. Q. et al. (2020). Hydrogen sulfide and rhizobia synergistically regulate nitrogen (N) assimilation and remobilization during N deficiency-induced senescence in soybean. Plant, Cell & Environment, 43(5), 1130–1147. [Google Scholar]
97. Rao, S., Yu, T., Cong, X., Xu, F., Lai, X. et al. (2020). Integration analysis of PacBio SMRT-and Illumina RNA-seq reveals candidate genes and pathway involved in selenium metabolism in hyperaccumulator Cardamine violifolia. BMC Plant Biology, 20(1), 1–20. [Google Scholar]
98. Petersen, A., Hansen, L. G., Mirza, N., Crocoll, C., Mirza, O. et al. (2019). Changing substrate specificity and iteration of amino acid chain elongation in glucosinolate biosynthesis through targeted mutagenesis of Arabidopsis methylthioalkylmalate synthase 1. Bioscience Reports, 39(7), 121–137. [Google Scholar]
99. Fernández-Martínez, A., Charlet, L. (2009). Selenium environmental cycling and bioavailability: A structural chemist point of view. Reviews in Environmental Science and Bio/Technology, 8(1), 81–110. [Google Scholar]
100. Premarathna, L., McLaughlin, M. J., Kirby, J. K., Hettiarachchi, G. M., Stacey, S. et al. (2012). Selenate-enriched urea granules are a highly effective fertilizer for selenium biofortification of paddy rice grain. Journal of Agricultural and Food Chemistry, 60(23), 6037–6044. [Google Scholar] [PubMed]
101. Kikkert, J., Berkelaar, E. (2013). Plant uptake and translocation of inorganic and organic forms of selenium. Archives of Environmental Contamination and Toxicology, 65(3), 458–465. [Google Scholar] [PubMed]
102. Trippe Iii, R. C., Pilon-Smits, E. A. H. (2021). Selenium transport and metabolism in plants: Phytoremediation and biofortification implications. Journal of Hazardous Materials, 404(285), 124178. [Google Scholar]
103. Pandey, A. K., Gautam, A., Dubey, R. S. (2019). Transport and detoxification of metalloids in plants in relation to plant-metalloid tolerance. Plant Gene, 17, 100171. [Google Scholar]
104. Barberon, M., Berthomieu, P., Clairotte, M., Shibagaki, N., Davidian, J. C. et al. (2008). Unequal functional redundancy between the two Arabidopsis thaliana high-affinity sulphate transporters SULTR1;1 and SULTR1;2. New Phytologist, 180(3), 608–619. [Google Scholar] [PubMed]
105. El Kassis, E., Cathala, N., Rouached, H., Fourcroy, P., Berthomieu, P. et al. (2007). Characterization of a selenate-resistant Arabidopsis mutant. Root growth as a potential target for selenate toxicity. Plant Physiology, 143(3), 1231–1241. [Google Scholar] [PubMed]
106. Renkema, H., Koopmans, A., Kersbergen, L., Kikkert, J., Hale, B. et al. (2012). The effect of transpiration on selenium uptake and mobility in durum wheat and spring canola. Plant and Soil, 354(1–2), 239–250. [Google Scholar]
107. Shin, R., Moon, J. Y. (2021). Selenium transport, accumulation and toxicity in plants. In: Plant growth and stress physiology, pp. 237–259. New York, USA: Springer. [Google Scholar]
Cite This Article
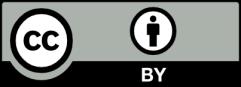
This work is licensed under a Creative Commons Attribution 4.0 International License , which permits unrestricted use, distribution, and reproduction in any medium, provided the original work is properly cited.