Open Access
ARTICLE
Impact of ZnSO and ZnO Nanoparticles on Seed Germination and Seedling Growth of Lettuce
1 Departamento de Horticultura, Universidad Autónoma Agraria Antonio Narro, Saltillo, 25315, México
2 Departamento de Materiales Avanzados, Centro de Investigación en Química Aplicada, Saltillo, 25294, México
3 Departamento de Agricultura Sustentable y Protegida, Universidad Tecnológica de Tehuacán, Tehuacán, 75859, México
* Corresponding Author: Nazario Francisco-Francisco. Email:
Phyton-International Journal of Experimental Botany 2023, 92(6), 1831-1840. https://doi.org/10.32604/phyton.2023.028085
Received 29 November 2022; Accepted 11 January 2023; Issue published 11 April 2023
Abstract
Micronutrient nanoparticles (NPs) are currently an option for chemical fertilization and biostimulation in crops. However, there is little information on the phytotoxic or biostimulatory effects of NPs at low concentrations of some elements, such as Zn. In this study, the effect of low concentrations of Zn oxide (ZnO) NPs on germination, growth variables, and nutritional attributes of lettuce (Lactuca sativa L.) was evaluated in comparison to Zn sulfate. Romaine lettuce seeds were treated with ZnSO4-- × 7H2O and ZnO NPs at Zn molar concentrations of 1 × 10−3, 5 × 10−3, 1 × 10−4, 5 × 10−4, 1 × 10−5, 5 × 10−5, 1 × 10−6, and 5 × 10−6. The seeds treated with ZnSO4− at 5 × 10−6 registered the highest radicle length, 73% more than the control treatment. The seeds treated with ZnSO4− at 5 × 10−3 registered the lowest values, with 50% less than the control treatment. ZnO NPs at 5 × 10−6 significantly increased content of chlorophyll A and B and total phenolics. These results indicate the possible existence of a mechanism related to the intrinsic nanoparticle properties, especially at low concentrations.
Keywords
Mineral micronutrients are essential for all living things [1]. Zinc is a micronutrient that is part of the catalytic and regulatory processes of animals and humans [1]. Zn is considered an essential micronutrient in plants because it plays a crucial role in many aspects of growth and development, however, excess Zn causes morphological, biochemical, and physiological disorders [2]. Zinc enrichment in plants has been regularly achieved through its application in ionic form in solid or liquid fertilizers as a chelated compound; more recently, its agricultural application incorporated in nanomaterials or the form of nanoparticles has been tested [3].
In addition to its value as a fertilizer and its well-known impact on growth and yield, Zn is an element that increases stress tolerance when applied to seeds, seedlings, or plants [2]. When used as a treatment for seed priming, Zn in ionic and nanometric forms is associated with improved germination and vigor responses, vegetative growth, accumulation of bioactive compounds, and stress tolerance [4,5]. The efficacy of inducing favorable responses to Zn treatments depends on the compound used, its concentration, and the form of application of the plant species used [6].
As with other elements, the biological impact of Zn in nanometric form seems to be greater than that obtained with ionic Zn. Therefore, there is interest in the development of fertilizers that contain different elements in nano form [7].
Zinc oxide (ZnO) nanoparticles (NPs) are among the most widely used in industry, with a range of applications such as personal care products, electrodes, biosensors, and solar cells [8]. Although many experiments have investigated the effect of ZnO-NPs at different stages of plant development [9], less information is available about the impact of ionic Zn compared to Zn NPs [10]. In a study in which ZnSO4− and NZnO were applied to Rosmarinus officinalis plants, no difference was found in the response of the plants to the two forms of Zn [11].
To our knowledge, little information is available about the differential impact of ionic Zn and NZnO when applied as seed priming treatments [12]. Seed treatment with ionic Zn or Zn-NPs is known to biostimulate the germination and growth of seedlings and plants [13,14,15]. In this sense, it is interesting to ask about the difference in the biostimulant impact on the lettuce seeds treated with the two forms of Zn.
In this study, the effect of priming lettuce (Lactuca sativa L.) seeds with ionic Zn and NZnO was evaluated. After seed treatment, germination variables, plant growth, mineral content, and photosynthetic pigment content were determined.
2.1 Characterization of ZnO NPs
ZnO NPs were synthesized using zinc acetate (Zn(CH3COO)2) as a precursor through a chemical hydrolysis reaction. The degree of crystallization of the ZnO NPs obtained was characterized by X-ray diffraction (XRD) in a Siemens model D-5000 diffractometer (SIEMENS, Munich, Germany) using KαCu radiation (25 mA, 35 kV) at room temperature in a sweep interval of 10° to 80° on the 2θ scale with a speed of 0.02 degrees/s. To analyze the functional groups, present in the NPs, a characterization was performed by Fourier transform infrared spectroscopy (FTIR) in a Shimadzu FTIR-8400 spectrophotometer in the range of 4,000 to 500 cm−1. To form the necessary tablets, the NPs were mixed with KBr. To observe the morphology and measure the size of the NPs, a FEI-Titan 80-300 kV transmission electron microscope (Cs-1.25 mm) operated at 300 kV was used. Using a WCIF ImageJ image analyzer, 400 particles were measured to obtain the dimensions of the synthesized nanoparticles.
2.2 Seed Germination of Lettuce
Lettuce seeds (L. sativa L.) var. Great Lakes (FAX seeds, Jalisco, México) were treated with ionic zinc (ZnSO4− * 7H2O, PM: 287.54 g mol−1) and ZnO NPs (PM: 81.38 g mol−1).
A total of 16 treatments were used for the present study, including Zn concentrations of 5 × 10−3, 1 × 10−3, 5 × 10−4, 1 × 10−4, 5 × 10−5, 1 × 10−5, 5 × 10−6 and 1 × 10−6 mol L−1 using ZnO NPs and ZnSO4− salt solution as a standard of zinc. The above treatments were equivalent to 327, 65.4, 32.7, 6.54, 3.27, 0.65, 0.327, and 0.065 mg Zn L−1, respectively. Stock solutions of ultrasonicated 5 × 10−3 mol L−1 ZnO-NPs and ZnSO4− were prepared in distilled water, and serial dilutions of the required concentrations of ZnO-NPs and ZnSO4− were prepared by using the formula:
C1×V1 = C2×V2C1×V1 = C2×V2 (1)
where: C1 and C2 represent the concentrations and V1 and V2 represent the volumes.
The seeds were kept imbibed in the different solutions for six hours in the dark and at room temperature, after which they were washed with distilled water. Seeds of the control treatment were dipped in distilled water. Sowing was carried out in Petri dishes previously sterilized with filter paper moistened with distilled water. The Petri dishes were established in a bioclimatic chamber (LAB-LINE) under 14 light hours at 25°C. Every day, the humidity level of the filter paper was verified, and if necessary, the humidity was recovered in all the Petri dishes. Seven days later, the germination percentage and the morphological aspects (hypocotyl and radicle length, seedling fresh and dry weight) were evaluated. The germination percentage (arc. sen. transformed), was measured using the following formula:
%Germination=Number of germinated seedsNumber of total seeds×100%Germination=Number of germinated seedsNumber of total seeds×100 (2)
A completely randomized design was used. There were three replicates for each treatment. Each Petri dish (representative of one replicate) had 25 seeds. Hypocotyl and radicle length were measured in cm by using a standard scale. Fresh and dry seedling weight was determined with an analytical balance and expressed in g.
To record the impact of Zn2+ and NZnO NPs on the bromatological, nutraceutical, and mineral contents of plants, the second set of seedlings were treated with the treatment that showed the best results in germination: 5 × 10−6 mol L−1 Zn2+ from ZnO NPs and ZnSO4−, plus a control (distilled water). The seeds were sown in polystyrene trays with 200 cavities containing perlite and peat moss in a 1:1 ratio. Two seeds were placed per cavity (total of 400 seeds), to keep the seedling that the best growth. Thirty days after emergence, the seedlings were transplanted into black polyethylene containers with a capacity of 5 L with the above-mentioned substrate mixture. The seedlings were established in a multitunnel greenhouse with a polyethylene plastic cover at an average temperature of 25°C. The treatments were applied to seedlings one, fifteen, and thirty days after transplanting. The containers were irrigated with a nutrient solution [16] at 25% concentration. 0.25 to 0.5 L of solution per plant was applied daily.
Sixty-four days after transplanting and once the lettuce reached maturity, evaluations of the fresh and dry weights of the plants were carried out. For this, adult plants were randomly selected from each treatment. The plants were collected and weighed fresh on an analytical balance. Subsequently, the head was separated from the lateral leaves and weighed fresh to determine the yield per plant. The complete plants were placed in paper bags and dried in a dehydrating oven at 70°C until reaching constant weight. Fresh tissue samples from the heads of lettuce were taken from the remaining five plants. To do this, a punch was used to obtain a representative sample of the tissues of the head. The samples were freeze-dried to determine the chlorophyll A, chlorophyll B, total chlorophyll, ascorbic acid contents and the total phenol content using the techniques described by Kim and coworkers [17].
With the described punch method, other leaf samples were collected from lettuce heads to determine the contents of Cu, Mn, Zn2+, Na+, K+, Mg2+, Ca2+, and P. N was quantified by the Kjeldahl method with digestion fast proposed by the Association of Official of Agricultural Chemist (AOAC) [18]. The content of P was determined by the ammonium metavanadate colorimetric method [18]. The microelements were determined by wet digestion with nitric acid [19]. Minerals were measured with a Varian AA-1275 atomic absorption spectrophotometer. The bromatological analysis was performed using the AOAC technique [20]. With this, the percentage of total dry matter, percentage of nitrogen, percentage of ethereal extract, crude fiber, percentage of moisture, and percentage of nitrogen-free extract were measured. The arrangement of the treatments was carried out under a randomized complete design; each treatment had three repetitions, and each repetition had six to twelve plants.
All data were analyzed by one-way PROC ANOVA using the SAS system for Windows (Version 9.4, SAS Institute Inc., Cary, NC, USA). Germination percentage (arc. sen. transformed) and morphological aspects means comparison test was conducted by least significant difference (L.S.D) at the 5% level. Mean values were calculated for a sample size of three plates, and in each plate, there were 25 seeds. However, mineral content, bromatological, and nutraceutical data were tested by Tukey’s multiple comparison test (p < 0.05) and data presented are means ± S.D. (n = 6 to 12).
3.1 Characterization of ZnO NPs
Fig. 1 shows that the synthesized ZnO NPs presented a quasi-spherical morphology with the formation of agglomerates that are characteristic in the synthesis of metal nanoparticles and/or metal oxides. The synthesized nanoparticles had an average diameter of 26 nm.
Figure 1: Micrograph showing the quasi-spherical morphology of the ZnO NPs and histogram of the distribution of the diameter of the nanoparticles
On the other hand, the detection of the characteristic planes of zinc by means of X-ray diffractometry allowed us to identify the presence of ZnO (Fig. 2).
Figure 2: X-ray diffractogram of ZnO NPs
Fig. 3 shows the FTIR spectrum of the ZnO NPs, in which the presence of a broad band was observed at 410 cm−1. This band was attributed to NZnO.
Figure 3: FTIR spectrum of the ZnO NPs
3.2 Effect of ZnSO4 − and ZnO NPs on the Germination and Morphology of Lettuce Seedlings
Table 1 shows the effects of ZnSO4− and ZnO NPs on the germination and growth variables of lettuce seedlings. Seeds treated with ZnSO4− at 5 × 10−6 mol L−1 registered the highest radicle and hypocotyl length compared to the rest of the treatments, while the seeds treated with ZnSO4− at 5 × 10−3 mol L−1 registered the lowest values according to LSD’s test (p < 0.05). In general, no significant differences were registered between the treatments in the percentage of germination, fresh and dry weight of the lettuce seedlings. These results show that the seeds treated with ZnO NPs did not significantly change the germination and morphological variables of the lettuce seedlings from 10−3 to 10−6 mol L−1 in comparison to the control, while ZnSO4− at 10−6 mol L−1 improved the morphological variables but had a negative impact at concentrations higher than 10−4 mol L−1.
According to the above results, in which only positive effects were registered at 5 × 10−6 mol L−1 treatments in both forms of Zn, a second experiment was conducted to evaluate the effects on the nutraceutical, mineral, and bromatological content in adult lettuce plants. Tables 2 and 3 show that there was no significant difference in the mineral and bromatological content between the treatments, while Table 4 with nutraceutical content shows that the maximum content of chlorophyll A, chlorophyll B, and phenols were significantly higher with the treatment of ZnO NPs at 5 × 10−6 mol L−1 (0.327 mg Zn L−1), with superiority in 38%, 50% and 40%, respectively, compared to the control treatment, but without differences in comparison to ZnSO4−.
The results of this work show that Zn ion is an element that bioestimulates the lettuce seedlings. Similar effects have been found in other plant species. Awan et al. [21] reported a stimulation of root and shoot length in broccoli seedling (Brassica oleracea var. italic) treated with ZnO NPs synthesized from ZnSO4− at low concentration. García-López et al. [22] found no significant differences in the percentage of germination of chili seeds (Capsicum annuum L.) embedded between the different concentrations of ZnO NPs; however, they found significant effects on the vigor of seedlings that emerged with ZnO NPs treatment. The stimulation of the vigor of wheat and bean seedlings has been achieved with the application of low levels of the element Zn, more than in comparison with other ions [23]. The lack of germination response to ZnO NP exposure in our study is consistent with the suggestion by Margenot et al. [24] that seed germination of various species is insensitive to toxicity by nanoparticles compared to root growth, mainly due to the minimum exposure time and the protective cover of the seed. Additional factors that may influence the seed response to germination include seed thickness and composition [25]. The previous results suggest that the range of biostimulant action of the ZnO nanoparticles, as well as Zn ion from ZnSO4−, is located at low concentrations, according to our results, specifically at the 10−6 molar concentration range.
On the other hand, in this study, it was observed that concentrations higher than 10−4 of both treatments caused a slight reduction in root growth. Regarding the latter, research reports null effects on the growth of other plant species to the application of zinc nanoparticles [26]. Yang et al. [27] reported that the phytotoxicity of corn and rice plants to ZnO NPs depends on the NP itself but not on the Zn2+ that is released from suspensions of ZnO NPs. It has been proposed that seedling radicles are more sensitive to toxicity by metal-oxide nanoparticles, such as CuO NPs [24]; however, no significant negative effects were observed in this study at the handled concentrations of ZnO NPs in comparison to zinc from the ZnSO4− salt.
Likewise, there are reports of the effects of Zn nanoparticles on the vitamin content of various plant species. Awan et al. [21] reported a 100% increase in chlorophyll content in broccoli plants (B. oleracea var. italic) with ZnO NP treatments at concentrations of 200 µg L−1. Raliya et al. [28] reported a high chlorophyll content in tomato plants treated with ZnO NPs; however, Wang et al. [29] found that concentrations greater than 400 mg dm−1 ZnO NPs reduced the chlorophyll content in tomato plants. Treatments of Triticum aestivum with zinc nanoparticles (250–200 mg L−1) increased protein and chlorophyll content [30]. The stimulation of chlorophyll content in plants by Zn nanoparticles is attributable to the fact that plants differ in absorption, translocation, accumulation and storage of this microelement; for example, legumes accumulate higher Zn content than cereals [1]. The increase in phenolic content induced by the application of ZnO NPs has been attributed to the defense response of plants to stress factors. García-López et al. [22] reported an increase in the phenolic content of C. annuum when they were treated with zinc nanoparticles.
The results of this study suggest that there are no significant toxic effects of ZnO NPs in the 10−6 molar concentration range of the lettuce seedling growth variables. Additionally, low concentrations of ZnO NPs suggest the stimulation of a higher content of chlorophyll A and B and total phenol content in adult lettuce plants.
Acknowledgement: To the QFB Bertha Puente Urbina and QFB Jesús Alejandro Espinosa, who supported the synthesis and characterization of the nanoparticles.
Funding Statement: The authors received no specific funding for this study.
Author Contributions: The authors confirm contribution to the paper as follows: study conception and design: R.B.G.; data collection: R.B.G.; analysis and interpretation of results: N.F.F.; draft manuscript preparation: A.B.M. All authors reviewed the results and approved the final version of the manuscript.
Conflicts of Interest: The authors declare that they have no conflicts of interest to report regarding the present study.
References
1. Veena, M., Puthur, J. T. (2021). Seed nutripriming with zinc is an apt tool to alleviate malnutrition. Environmental Geochemistry and Health, 44(8), 2355–2373. https://doi.org/10.1007/s10653-021-01054-2 [Google Scholar] [PubMed] [CrossRef]
2. Balafrej, H., Bogusz, D., Abidine Triqui, Z., Guedira, A., Bendaou, N. et al. (2020). Zinc hyperaccumulation in plants: A review. Plants, 9(5), 562. https://doi.org/10.3390/plants9050562 [Google Scholar] [PubMed] [CrossRef]
3. Ahmed, R., Yusoff Abd Samad, M., Uddin, M., Quddus, M., Hossain, M. A. (2021). Recent trends in the foliar spraying of zinc nutrient and zinc oxide nanoparticles in tomato production. Agronomy, 11(10), 2074. https://doi.org/10.3390/agronomy11102074 [Google Scholar] [CrossRef]
4. Farooq, M., Almamari S. A. D., Rehman, A., Al-Busaidi, W. M., Wahid, A. et al. (2021). Morphological, physiological and biochemical aspects of zinc seed priming-induced drought tolerance in faba bean. Scientia Horticulturae, 281, 109894. https://doi.org/10.1016/j.scienta.2021.109894 [Google Scholar] [CrossRef]
5. Garza-Alonso, C. A., González-García, Y., Cadenas-Pliego, G., Olivares-Sáenz, E., Trejo-Téllez, L. I. et al. (2021). Seed priming with ZnO nanoparticles promotes early growth and bioactive compounds of Moringa oleifera. Notulae Botanicae Horti Agrobotanici Cluj-Napoca, 49(4), 12546. https://doi.org/10.15835/nbha49412546 [Google Scholar] [CrossRef]
6. Gomes, M. J. C., Martino, H. S. D., Tako, E. (2021). Zinc-biofortified staple food crops to improve zinc status in humans: A systematic review. Critical Reviews in Food Science and Nutrition, 1–13. https://doi.org/10.1080/10408398.2021.2010032 [Google Scholar] [PubMed] [CrossRef]
7. Babu, S., Singh, R., Yadav, D., Rathore, S. S., Raj, R. et al. (2021). Nanofertilizers for agricultural and environmental sustainability. Chemosphere, 292(5), 133451. https://doi.org/10.1016/j.chemosphere.2021.133451 [Google Scholar] [PubMed] [CrossRef]
8. Czyżowska, A., Barbasz, A. (2022). A review: Zinc oxide nanoparticles–friends or enemies? International Journal of Environmental Health Research, 32(4), 885–901. https://doi.org/10.1080/09603123.2020.1805415 [Google Scholar] [PubMed] [CrossRef]
9. Thounaojam, T. C., Meetei, T. T., Devi, Y. B., Panda, S. K., Upadhyaya, H. (2021). Zinc oxide nanoparticles (ZnO-NPsA promising nanoparticle in renovating plant science. Acta Physiologiae Plantarum, 43(10), 136. https://doi.org/10.1007/s11738-021-03307-0 [Google Scholar] [CrossRef]
10. Sturikova, H., Krystofova, O., Huska, D., Adam, V. (2018). Zinc, zinc nanoparticles and plants. Journal of Hazardous Materials, 349, 101–110. https://doi.org/10.1016/j.jhazmat.2018.01.040 [Google Scholar] [PubMed] [CrossRef]
11. Mohsenzadeh, S., Moosavian, S. S. (2017). Zinc sulphate and nano-zinc oxide effects on some physiological parameters of Rosmarinus officinalis. American Journal of Plant Sciences, 8(11), 2635–2649. https://doi.org/10.4236/ajps.2017.811178 [Google Scholar] [CrossRef]
12. Sharifi, R., Mohammadi, K., Rokhzadi, A. (2016). Effect of seed priming and foliar application with micronutrients on quality of forage corn (Zea mays). Environmental and Experimental Biology, 14(4), 151–156. https://doi.org/10.22364/eeb.14.21 [Google Scholar] [CrossRef]
13. Imran, M., Boelt, B., Mühling, K. H. (2018). Zinc seed priming improves salt resistance in maize. Journal of Agronomy and Crop Science, 204(4), 390–399. https://doi.org/10.1111/jac.12272 [Google Scholar] [CrossRef]
14. Haider, M. U., Hussain, M., Farooq, M., Nawaz, A. (2020). Optimizing zinc seed priming for improving the growth, yield and grain biofortification of mungbean (Vigna radiata (L.) wilczek). Journal of Plant Nutrition, 43(10), 1438–1446. https://doi.org/10.1080/01904167.2020.1730895 [Google Scholar] [CrossRef]
15. Tondey, M., Kalia, A., Singh, A., Dheri, G. S., Taggar, M. S. et al. (2021). Seed priming and coating by nano-scale zinc oxide particles improved vegetative growth, yield and quality of fodder maize (Zea mays). Agronomy, 11(4), 729. https://doi.org/10.3390/agronomy11040729 [Google Scholar] [CrossRef]
16. Steiner, A. A. (1961). A universal method for preparing nutrient solutions of a certain desired composition. Plant and Soil, 15(2), 134–154. https://doi.org/10.1007/BF01347224 [Google Scholar] [CrossRef]
17. Kim, K. T., Yoo, K. M., Lee, J. W., Eom, S. H., Hwang, I. K. et al. (2007). Pro-tective effect of steamed American ginseng (Panax quinquefolius L.) on V79-4 cells induced by oxidative stress. Journal of Ethnopharmacology, 111(3), 443–445. https://doi.org/10.1016/j.jep.2007.01.004 [Google Scholar] [PubMed] [CrossRef]
18. AOAC (1980). Official methods of analysis, 13th editionpp. 858–859. Washington DC: Association of the Official Agricultural Chemists. [Google Scholar]
19. Fick, K. R., Miller, S. M., Funk, J. D., McDowell, L. R., Houser, R. H. (1976). Methods of mineral anaysis for plant and animal tissues, 2nd edition. Gainesville, Florida: University of Florida, Institute of Food and Agriculture. [Google Scholar]
20. AOAC (1997). Official method of analysis association of official analytical chemist, 15th edition. Washington DC, USA: AOAC. [Google Scholar]
21. Awan, S., Shahzadi, K., Javad, S., Tariq, A., Ahmad, A. et al. (2021). A preliminary study of influence of zinc oxide nanoparticles on growth parameters of Brassica oleracea var italic. Journal of the Saudi Society of Agricultural Sciences, 20(1), 18–24. https://doi.org/10.1016/j.jssas.2020.10.003 [Google Scholar] [CrossRef]
22. García-López, J. I., Zavala-García, F., Olivares-Sáenz, E., Lira-Saldívar, R. H., Díaz Barriga-Castro, E. et al. (2018). Zinc oxide nanoparticles boosts phenolic compounds and antioxidant activity of Capsicum annuum L. during germination. Agronomy, 8(10), 215. https://doi.org/10.3390/agronomy8100215 [Google Scholar] [CrossRef]
23. El-Rasafi, T., Nouri, M., Bouda, S., Haddioui, A. (2016). The effect of Cd, Zn and Fe on seed germination and early seedling growth of wheat and bean. Ekológia, 35(3), 213–223. https://doi.org/10.1515/eko-2016-0017 [Google Scholar] [CrossRef]
24. Margenot, A. J., Rippner, D. A., Dumlao, M. R., Nezami, S., Green, P. G. et al. (2018). Copper oxide nanoparticle effects on root growth and hydraulic conductivity of two vegetable crops. Plant and Soil, 431(1), 333–345. https://doi.org/10.1007/s11104-018-3741-3 [Google Scholar] [CrossRef]
25. Zhang, W. H., Zhou, Y., Dibley, K. E., Tyerman, S. D., Furbank, R. T. et al. (2007). Nutrient loading of developing seeds. Functional Plant Biology, 34(4), 314–331. https://doi.org/10.1071/FP06271 [Google Scholar] [PubMed] [CrossRef]
26. Lin, D., Xing, B. (2007). Phytotoxicity of nanoparticles: Inhibition of seed germination and root growth. Environmental Pollution, 150(2), 243–250. https://doi.org/10.1016/j.envpol.2007.01.016 [Google Scholar] [PubMed] [CrossRef]
27. Yang, Z., Chen, J., Dou, R., Gao, X., Mao, C. et al. (2015). Assessment of the phytotoxicity of metal oxide nanoparticles on two crop plants, maize (Zea mays L.) and rice (Oryza sativa L.). International Journal of Environmental Research and Public Health, 12(12), 15100–15109. https://doi.org/10.3390/ijerph121214963 [Google Scholar] [PubMed] [CrossRef]
28. Raliya, R., Nair, R., Chavalmane, S., Wang, W. N., Biswas, P. (2015). Mechanistic evaluation of translocation and physiological impact of titanium dioxide and zinc oxide nanoparticles on the tomato (Solanum lycopersicum L.) plant. Metallomics, 7(12), 1584–1594. https://doi.org/10.1039/c5mt00168d [Google Scholar] [PubMed] [CrossRef]
29. Wang, X. P., Li, Q. Q., Pei, Z. M., Wang, S. C. (2018). Effects of zinc oxide nanoparticles on the growth, photosynthetic traits, and antioxidative enzymes in tomato plants. Biologia Plantarum, 62(4), 801–808. https://doi.org/10.1007/s10535-018-0813-4 [Google Scholar] [CrossRef]
30. Ramesh, M., Palanisamy, K., Babu, K., Sharma, N. K. (2014). Effects of bulk & nanotitanium dioxide and zinc oxide on physio-morphological changes in Triticum aestivum Linn. Journal of Global Bioscience, 3(2), 415–422. [Google Scholar]
Cite This Article
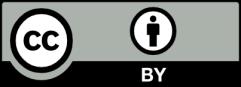
This work is licensed under a Creative Commons Attribution 4.0 International License , which permits unrestricted use, distribution, and reproduction in any medium, provided the original work is properly cited.