Open Access
ARTICLE
Clonal Transgenerational Effects Transmit for Multiple Generations in a Floating Plant
1 College of Life Science, Sichuan Normal University, Chengdu, 610101, China
2 Institute of Wetland Ecology & Clone Ecology, Taizhou University, Taizhou, 318000, China
3 School of Ecology and Nature Conservation, Beijing Forestry University, Beijing, 100083, China
4 College of Ecology and Environment, Chengdu University of Technology, Chengdu, 610059, China
* Corresponding Authors: Jin-Song Chen. Email: ; Fei-Hai Yu. Email:
Phyton-International Journal of Experimental Botany 2023, 92(5), 1589-1601. https://doi.org/10.32604/phyton.2023.027113
Received 14 October 2022; Accepted 22 November 2022; Issue published 09 March 2023
Abstract
Environmental conditions of a parent plant can influence the performance of their clonal offspring, and such clonal transgenerational effects may help offspring adapt to different environments. However, it is still unclear how many vegetative generations clonal transgenerational effects can transmit for and whether it depends on the environmental conditions of the offspring. We grew the ancestor ramets of the floating clonal plant Spirodela polyrhiza under a high and a low nutrient level and obtained the so-called 1st-generation offspring ramets of two types (from these two environments). Then we grew the 1st-generation offspring ramets of each type under the high and the low nutrient level and obtained the so-called 2nd-generation offspring ramets of four types. We repeated this procedure for another five times and analyzed clonal transgenerational effects on growth, morphology and biomass allocation of the 1st- to the 6th-generation offspring ramets. We found positive, negative or neutral (no) transgenerational effects of the ancestor nutrient condition on the offspring of S. polyrhiza, depending on the number of vegetative generations, the nutrient condition of the offspring environment and the traits considered. We observed significant clonal transgenerational effects on the 6th-generation offspring; such effects occurred for all three types of traits (growth, morphology and allocation), but varied depending on the nutrient condition of the offspring environment and the traits considered. Our results suggest that clonal transgenerational effects can transmit for multiple vegetative generations and such impacts can vary depending on the environmental conditions of offspring.Keywords
Environmental fluctuations may induce phenotype changes of organisms [1,2]. While the genotype of an individual and the environmental condition that the individual is currently experiencing can often determine its phenotype, the environmental conditions that its elders (parent, grandparent, grand-grandparent and so on) had experienced may also play an important role, a phenomenon termed as transgenerational effects, maternal effects or parental effects [3–5]. Transgenerational effects have been widely demonstrated in plant species [6–10], and can influence the phenotype of an offspring via influencing e.g., vegetative propagule size [11], seed quality [7], germination [12], flowering time [13], physiology [14], morphology [15], and growth [16].
Many plant species can reproduce by clonal growth [17–22], and some clonal plants rely mainly on clonal growth to sustain their populations [23–26]. Most of the epigenetic regulations on phenotype changes are not inherited to sexually produced offspring because it is reset during meiosis [27], but clonal growth has no meiotic process and thus bypasses epigenetic resetting [28–30]. In addition, as short-distance clonal reproduction results in predictable conditions for offspring, passing information across vegetative generations may confer benefits for the offspring of clonal plants [31]. In terms of resource provision, even the smallest clonal fragments that function as clonal propagules may provide significantly more resources than their sexual propagules [9]. Therefore, transgenerational effects may be particularly important in clonal plants [5–7,32], and may have a profound impact on their life cycle and population dynamics, which may further influence community structure and ecosystem function and stability [33–35].
While an individual (ramet) of clonal plants may produce offspring ramets of a number of vegetative generations within a single growing season [36,37], transgenerational effects on different generations of clonal offspring have been rarely considered [38,39]. If resource provision is the main mechanism underlying clonal transgenerational effects, as previously reported [40], then the magnitude of transgenerational effects on the offspring performance should decrease within increasing generations of clonal offspring. If epigenetic memory is the main mechanism underlying clonal transgenerational effects [41], then their magnitude could vary with clonal generations depending on the stability of the epigenetic memory [42]. Therefore, for a better understanding of clonal transgenerational effects, their roles in regulating the performance of clonal offspring of different generations should be investigated. So far, however, it is still unclear how many vegetative generations clonal transgenerational effects can transmit for and whether these effects are transient.
Transgenerational effects on the performance of offspring may vary depending on the environment where the offspring currently grow [8,31,40]. For instance, the offspring of Plantago lanceolata grown under high nutrient availability produced more carbohydrates in roots when their parents were grown under high than under low nutrient availability, but such transgenerational nutrient effects disappeared when the offspring were grown under low nutrient availability [43]. Similarly, the offspring of Polygonum persicaria grown under low light availability had larger leaf area, specific leaf area and biomass when their parents were grown under low than under high light availability, but such transgenerational light effects disappeared when the offspring were grown under high light availability [8]. In addition, transgenerational nutrient effects on the performance the offspring population of Alternanthera philoxeroides were stronger when the offspring were grown under high than under low nutrient availability [40]. Thus, transgenerational effects on offspring of different generations may also vary with the environmental conditions of the offspring. However, it is still unknown whether the number of vegetative generations that clonal transgenerational effects can persist for varies depending on the environmental conditions of the offspring.
To understand clonal transgenerational effects on offspring of different generations, we conducted a total of seven consecutive experiments with ancestor ramets and their six generations of offspring of the fast-growing clonal plant Spirodela polyrhiza. Thus, we could test whether clonal transgenerational effects regulate the performance of the 1st- to the 6th-generation offspring. An exponential increase of the number of treatments prevented us to test those effects on the performance of more generations (e.g., on the 7th-generaiton offspring). Thus, we specifically addressed the following questions: (1) How many vegetative generations can clonal transgenerational effects on the performance (i.e., growth, morphology and biomass allocation) of offspring transmit for, and can they transmit to the offspring ramets of up to the 6th generation? (2) Does the nutrient level of offspring influence the number of vegetative generations that transgenerational effects can transmit for?
Spirodela polyrhiza (L.) Schleiden (Lemnaceae) is a perennial, floating, clonal plant, and occurs in many temperate and tropical regions of the world [44]. Ramets (individuals) of S. polyrhiza are small with flat and obovate leaves of 5–10 mm long and 3-8 mm wide and roots of 3–5 cm long. This species can propagate through clonal growth, producing daughter fronds from parent fronds at the proximal end in two meristematic pocket regions. A ramet of S. polyrhiza commonly consists of two fronds and some roots [45–47]. The speed of clonal growth is very fast, and a parent ramet can produce an offspring ramet within 1 to 3 days (X-M Zhang personal observation). This species prefers to live in quiet water bodies such as slow-moving streams, ditches and pools. Due to its rapid clonal growth, this species can form a dense mat, covering the surface of the whole water body and creating a dark and anoxic environment that is hard for submerged plants and aquatic animals to survive [44]. Thus, the bloom of this species can have negative impacts on fisheries [48].
On 1 June 2020, we collected ramets of S. polyrhiza from a small area (about 2 m2) in a slow-moving stream in Taizhou (28°30′ N; 121°210′ E), Zhejiang Province, China. Because S. polyrhiza can vegetatively propagate very quickly, the ramets that were collected were very likely from the same clone. The collected ramets were vegetatively propagated in a greenhouse in the Jiaojiang campus of Taizhou University, Zhejiang Province, China. The plants were washed several times with double distilled water and then rinsed with 0.01 M NaClO for 30 s to reduce microbial and algal growth. The sterilized plants were cultivated with 10% of the Hoagland solution in plastic tanks (64 cm long × 42 cm wide × 14 cm deep). The Hoagland solution contained 945 mg/L Ca(NO3)2·4H2O, 506 mg/L KNO3, 80 mg/L NH4NO3, 136 mg/L KH2PO4, 493 mg/L MgSO4, 13.9 mg/L FeSO4·7H2O, and 18.7 mg/L EDTA·2Na [45].
A total of seven consecutive experiments were conducted with ramets of S. polyrhiza (Fig. 1). The 1st experiment started on 18 June 2020. A total of 192 similar-sized ramets (thereafter referred to as ancestor ramets) of S. polyrhiza, each having two fronds and some roots, were selected and grown under two nutrient levels (low vs. high, i.e., in 12.5% vs. 100% Hoagland solution). Each treatment had 12 replicates, yielding 24 pots. Every pot was initially grown with eight ramets of S. polyrhiza. The pots were each 17.5 cm in diameter and 10 cm in depth, containing 600 mL of either 12.5% or 100% Hoagland solution. The pots were randomly placed on a bench in the same greenhouse where the plants were cultivated. The nutrient solution in each pot was replaced every four days. The 1st experiment lasted for 17 days and ended on 05 July 2020 when the water surface of the pots in the high nutrient level was fully covered by the offspring ramets of S. polyrhiza. For simplicity, we named all offspring ramets produced during this period as the 1st-generation offspring ramets, which actually included the offspring ramets of several vegetative generations as the speed of clonal growth is rather fast in this species.
Figure 1: Experimental design. A total of seven consecutive experiments were conducted with ramets of S. polyrhiza. In the 1st experiment, ancestor ramets were subjected to two nutrient levels (high vs. low) and produced the so-called 1st-generation offspring ramets. In the 2nd experiment, the 1st-generation offspring ramets of each of the two types (from the high and low nutrient level of the ancestor ramets) were subjected to the same two nutrient levels, resulting in four treatments, and produced the so-called 2nd-generation offspring ramets. In the 3rd experiment, the 2nd-generation offspring ramets of each of the four types were subjected to the same two nutrient levels, resulting in eight treatments, and produced the 3rd-generation offspring ramets. A similar procedure was repeated for another four times
The 2nd experiment started on 05 July 2020. We selected 192 similar-sized, 1st-generation offspring ramets from each of the low- and the high-nutrient treatment of the 1st experiment. Then we subjected each type of the 1st-generation offspring ramets to the same two nutrient levels, i.e., placed eight ramets in a pot (17.5 cm in diameter and 10 cm in depth) containing 600 mL of either 12.5% or 100% Hoagland solution. Therefore, the 2nd experiment had four treatments, i.e., two nutrient treatments of the ancestor ramets in the 1st experiment crossed with two nutrient treatments of the 1st-generation offspring ramets in the 2nd experiment. Each treatment was replicated 12 times, resulting in 48 pots. All pots were randomly placed on the bench in the greenhouse and the nutrient solution in each pot was replaced every four days. The 2nd experiment lasted for 12 days. We named all offspring ramets produced during this period as the 2nd-generation offspring ramets.
For the 3rd experiment, the 2nd-generation offspring ramets of each of the four types were grown under the same two nutrient levels. Thus, the 3rd experiment had eight treatments, i.e., two nutrient treatments of the ancestor ramets in the 1st experiment, two nutrient treatments of the 1st-generation offspring ramets in the 2nd experiment and two nutrient treatments of the 2nd-generation offspring ramets in the 3rd experiment. Each treatment was replicated 12 times, resulting in 96 pots. Similarly, we named all offspring ramets produced during this period as the 3rd-generation offspring ramets. The 3rd experiment was maintained for 10 days, from 17 July to 27 July 2020.
A similar procedure was repeated for another four times (Fig. 1). Briefly, the 4th experiment consisted of 16 treatments on the 3rd-generation offspring ramets, the 5th experiment consisted of 32 treatments on the 4th-generation offspring ramets, the 6th experiment consisted of 64 treatments on the 5th-generation offspring ramets, and the 7th experiment consisted of 128 treatments on the 6th-generation offspring ramets. For the 4th experiment, each treatment was replicated six times, resulting in 96 pots; for the 5th experiment, each treatment was replicated four times, resulting in 128 pots; for the 6th experiment, each treatment was replicated four times, resulting in 256 pots; for the 7th experiment, each treatment was replicated three times, resulting in 384 pots. The 4th experiment was maintained for 9 days (from 27 July to 05 August 2020), the 5th experiment for 9 days (from 5 August to 14 August 2020), the 6th experiment for 10 days (from 14 August to 24 August 2020) and the 7th experiment for 10 days (from 24 August to 03 September 2020). During the experiments, the mean air temperature and relative humidity in the greenhouse were 27°C and 87%, respectively.
At the end of each experiment, we first counted the ramets in each pot. Then, we randomly selected 15 ramets from each pot, and measured their frond length, frond width and the longest root length. The remaining ramets were also harvested. All ramets were separated into fronds and roots, dried at 75°C for 24 h and weighed. For logistics reason, we did not measure physiological and biochemical traits because these traits are not as easy to measure as the growth and morphological traits.
We calculated total biomass and ramet number per initial ramet of S. polyrhiza in each pot, i.e., total biomass and ramet number of the whole pot divided by eight. Root to shoot ratio was calculated as root mass divided by frond mass, and mass per ramet as total biomass divided by total number of ramets. Then we quantified clonal transgenerational effects of the ancestor ramets on each measure of growth (total mass, frond mass, root mass and number of ramets), morphology (frond length, frond width, longest root length and mass per ramet) and allocation (root to shoot ratio) of the 1st-, 2nd-, 3rd-, 4th-, 5th- and 6th-generation offspring ramets, respectively.
Using the data collected from the 2nd experiment, we quantified clonal transgenerational effects of the ancestor ramets on the 1st-generation offspring ramets when they were grown under the nutrient level i (TEi) as: TEi = (Xi,H – Xi,L)/Xi,L, where Xi,H and Xi,L are the performance (e.g., biomass) of the 1st-generation offspring ramets derived from the ancestor ramets grown under the high and the low nutrient level, respectively, when the 1st-generation offspring ramets were grown under the nutrient level i, and i is either the high or the low nutrient level (Fig. 1). To quantify the transgenerational effects of the ancestor ramets on the 2nd-generation offspring ramets, we used the data collected from the 3rd experiment. We first averaged the data across the two nutrient treatments of the 2nd-generation offspring ramets for each of four treatment combinations, i.e., the two nutrient treatments of the ancestor ramets crossed with the two nutrient treatments of the 1st-generation offspring ramets (Fig. 1). Then, we used the same formula to calculate the transgenerational effects of the ancestor ramets on the 2nd-generation offspring ramets when the 1st-generation offspring ramets were subjected to the high and the low nutrient level, respectively. Using similar approaches with the same formula, we quantified the transgenerational effects of the ancestor ramets on the 3rd-, 4th-, 5th- and 6th-generation offspring ramets when the 1st-genration offspring ramets were subjected to the high and the low nutrient level, respectively. Significant positive and negative values of the calculated clonal transgenerational effects mean that the high nutrient level of the ancestor ramets promotes and suppresses the performance of their offspring ramets, respectively, compared to the low nutrient level of the ancestor ramets.
One-sample t-test was used to examine whether the clonal transgenerational effects on each growth, morphology and allocation measure of the offspring ramets of each of the six generations under each nutrient level were significantly different from zero. We also used two-way ANOVA to test the effects of offspring generation (from 1st to 6th generation) and nutrient level of the 1st-generation offspring ramets (high vs. low) on the clonal transgenerational effects on each measure. When a significant effect was detected, Duncan test was used for multiple comparisons. All analyses were done using SPSS Statistics 22.0 software (IBM Corp., Armonk, NY, USA).
3.1 Clonal Transgenerational Effects on Growth
Clonal transgenerational effects (TEs) of the nutrient condition of the ancestor ramets on all growth measures varied significantly among offspring generations, and such effects also depended on the nutrient level of the 1st-generation offspring ramets (significant effects of offspring generation and offspring generation × nutrient level; Table 1A, Fig. 2). For the 1st-generation offspring ramets of S. polyrhiza, we observed significant positive TEs on all four growth measures, except root mass when the 1st-generation offspring ramets were grown under high nutrients (Fig. 2). For the 2nd- and the 3rd-generation offspring ramets, there were no significant TEs on any of the growth measures under either nutrient level (Fig. 2). For the 4th-generation offspring, there were significant positive TEs on root mass and no significant TEs on total mass, frond mass or number of ramets when the 1st-generation offspring were grown under high nutrients, but there were significant negative TEs on all four growth measures when they were grown under low nutrients (Fig. 2). For the 5th-generation offspring ramets, there were significant negative TEs on total mass and frond mass, irrespective of the nutrient level of the 1st-generation offspring (Figs. 2A, 2B), but had no significant impacts on root mass or number of ramets (Fig. 2C). For the 6th-generation offspring ramets, TEs significantly positively influenced total mass and frond mass when the 1st-generation offspring were grown under low nutrients (Figs. 2A, 2B), positively influenced root mass when they were grown under high nutrients (Fig. 2C), but had no significant effects on number of offspring (Fig. 2D).
Figure 2: Transgenerational effects (TEs) on (A–C) biomass and (D) number of ramets of the 1st-, 2nd-, 3rd-, 4th-, 5th- and 6th-generation offspring ramets of S. polyrhiza when the 1st-generation offspring ramets were grown under the high and low nutrient level. Mean + SE are shown. Symbols (**p < 0.01; *p < 0.05 and #p < 0.1) indicate significant or marginally significant difference from zero; significant positive and negative values mean that the high nutrient level of the ancestor ramets promotes and suppresses the performance of their offspring ramets, respectively, compared to the low nutrient level of the ancestor ramets. Different lowercase letters indicate significant difference among the 12 treatment combinations of the six offspring generations and two nutrient levels
3.2 Clonal Transgenerational Effects on Morphology
Offspring generation, nutrient level and their interaction significantly affected TEs on frond length and frond width (all p < 0.05; Table 1B, Figs. 3A, 3B). Offspring generation and its interaction with nutrient level also significantly affected mass per ramet (Table 1B; Fig. 3D). TEs on length of the longest root were significantly influenced by the interaction effect of offspring generation and nutrient level, but not by the main effects (Table 1B; Fig. 3C).
Figure 3: Transgenerational effects (TEs) on (A) frond length, (B) frond width, and (C) the longest root length and (D) mass per ramet of the 1st-, 2nd-, 3rd-, 4th-, 5th- and 6th-generation offspring ramets of S. polyrhiza when the 1st-generation offspring ramets were grown under the high and low nutrient level. Mean + SE are shown. Symbols (**p < 0.01; *p < 0.05 and #p < 0.1) indicate significant or marginally significant difference from zero; significant positive and negative values mean that the high nutrient level of the ancestor ramets promotes and suppresses the performance of their offspring ramets, respectively, compared to the low nutrient level of the ancestor ramets. Different lowercase letters indicate significant difference among the 12 treatment combinations of the six offspring generations and two nutrient levels
When the 1st-generation offspring ramets were grown under high nutrients, we observed significant negative TEs on frond length of the 1st- and 2nd-generation offspring ramets and on frond width of the 1st-, 2nd- and 5th-generation offspring ramets (Figs. 3A, 3B). However, when the 1st-generation offspring ramets were grown under low nutrients, we found significant positive TEs on frond length of the 3rd- and 5th-generation offspring and significant negative TEs on frond width of the 6th generation offspring (Figs. 3A, 3B). When the 1st-generation offspring ramets were grown under high nutrients, TEs on length of the longest root were significantly negative for the 1st-generation offspring and significantly positive for the 2nd-, 4th- and 6th-generation offspring (Fig. 3C). By contrast, when the 1st-generation offspring were grown under low nutrients, TEs on length of the longest root were significantly positive for the 1st-generation offspring and significantly negative for the 5th- and 6th-generation offspring (Fig. 3C). TEs on mass per ramet were significantly negative for the 5th-generation offspring when the 1st-generation offspring were grown under high nutrients, and were significantly negative for the 1st-generation offspring and significantly positive for the 6th-generation offspring when the 1st-generation offspring were grown under low nutrients (Fig. 3D).
3.3 Clonal Transgenerational Effects on Allocation
When the 1st-generation offspring ramets were grown under high nutrients, TEs on root to shoot ratio were significantly negative for the 3rd-generation offspring ramets, significantly positive for the 4th- and 6th-generation offspring, and not significantly different from zero for the 1st-, 2nd- and the 5th-generation offspring (Fig. 4). However, when the 1st-generation offspring ramets were grown under low nutrients, we found no significant TEs on root to shoot ratio for any of the six generations offspring (Fig. 4).
Figure 4: Transgenerational effects (TEs) on root to shoot ratio of the 1st-, 2nd-, 3rd-, 4th-, 5th- and 6th-generation offspring ramets of S. polyrhiza when the 1st-generation offspring ramets were grown under the high and low nutrient level. Mean + SE are shown. Symbols (**p < 0.01; *p < 0.05 and #p < 0.1) indicate significant or marginally significant difference from zero; significant positive and negative values mean that the high nutrient level of the ancestor ramets promotes and suppresses the performance of their offspring ramets, respectively, compared to the low nutrient level of the ancestor ramets. Multiple comparison was not done as there was no significant effect of offspring generation, nutrient level or their interaction
While transgenerational effects on the performance of offspring have been widely reported in both clonal and non-clonal plants [5,6,43,49,50], little is known about whether they can transmit for several generations, particularly for clonal plants [38,39]. We found significant clonal transgenerational nutrient effects of the ancestor ramets of S. polyrhiza on growth (Figs. 2A–2C), morphology (Figs. 3B, 3C) and biomass allocation (Fig. 4) of their offspring ramets of up to the sixth generation. Because each generation that we defined in this study included actually several vegetative generations due to the fast clonal growth of this species, these results suggest that clonal transgenerational effects could be transmitted for a number of vegetative generations in the floating plant S. polyrhiza.
In previous studies on animals, it was found that the temperature of grandparents of marine sticklebacks (Gasterosteus aculeatus) significantly influenced the hatching success and body size of their offspring, suggesting that transgenerational effects can transmit for two generations in this fish [51]. Similarly, significant transgenerational salinity effects were observed on the net reproductive rate of the 2nd-genration offspring of Daphnia magna [52]. In a recent study, clonal fragmentation of grandparent ramets of the stoloniferous floating plant Pistia stratiotes decreased biomass of their offspring ramets but increased offspring number, suggesting that clonal transgenerational effects can also transmit for two vegetative generations [38]. Limited by the experimental setting (due to the exponential increase of the number of treatments with increasing vegetative generations; Fig. 1), we tested clonal transgenerational effects on the performance of offspring ramets of only six generations. Given that we could manage to test the effects for more generations, we may observe significant transgenerational effects on the offspring performance of more generations. Thus, clonal transgenerational effects can potentially transmit for more than six generations, which could have profound impacts on the dynamics and long-term persistence of clonal plant populations [30,47].
However, the number of vegetative generations that clonal transgenerational effects of S. polyrhiza could transmit for varied depending on the nutrient condition of the 1st-generation offspring ramets. For instance, clonal transgenerational effects on frond mass were transmitted to up to the 6th-generation offspring when the 1st-generation offspring were grown under the low nutrient level, but to only the 5th-generation offspring when they were grown under the high nutrient level (Fig. 2B). Transgenerational effects have been commonly shown to vary with offspring environmental conditions such as nutrient availability [40,43], light intensity [8], and soil water content [5], salinity [13], herbivory [14] and heavy metal contamination [31]. Our findings proved for the first time that the number of vegetative generations that clonal transgenerational effects can transmit for also depends on the environmental conditions of the offspring.
In S. polyrhiza, the direction and magnitude of clonal transgenerational effects varied greatly with vegetative offspring generations and also depended on the nutrient conditions where the 1st-generation offspring ramets were grown. We observed significant positive, negative or neutral (no) transgenerational effects on offspring growth, morphology and allocation of S. polyrhiza (Figs. 2–4). Environmental changes may induce changes in epigenetic modification such as DNA methylation, and these modifications still retain a degree of reversibility [2,5]. Thus, the possible explanation is that transgenerational effects are limited by epigenetic stability, as some of epigenetic modifications are reset across generations [53]. For instance, epigenetic changes of Arabidopsis thaliana induced by exposure to heat and salt stress over multiple generations can lead to reversible, transgenerational phenotype changes [13].
Transgenerational effects of plants to environmental stress can transmit across generations via different mechanisms [54]. In addition to epigenetic modifications, environmental conditions are known to affect the quantity and quality of resources provided by ancestors to their offspring, which may be critical for offspring survival and growth [3,4,55]. We observed significant positive transgenerational nutrient effects on growth of the 1st-generation offspring ramets, and such positive effects tended to decrease when the 1st-generaiton offspring were grown under low nutrients (Fig. 2). These results suggest that resource provision should have played a role in the clonal transgenerational effects of S. polyrhiza, as reported in other studies [9,38,46]. Surprisingly, we also observed negative transgenerational effects, suggesting that mechanisms other than resource provision should have also played an important role.
We conclude that clonal transgenerational effects can transmit for more than six vegetative generations and such impacts can vary depending on the environmental conditions of offspring. Future studies that integrate epigenetic and ecological approaches should be used to verify whether epigenetic modifications (such as DNA methylation) and resource provision are the main mechanisms responsible for the fluctuations in transgenerational effects across different generations and whether such fluctuations are adaptive. Our findings highlight the importance to test the roles of transgenerational effects across multiple generations. As S. polyrhiza is a tiny free-floating plant with very fast clonal regeneration [44,45], future studies should also consider other types of clonal plants (e.g., with large size and relatively slow regeneration rate) to test the generality of our findings.
Acknowledgement: We thank Si-Mei Yao for assistance with the experiment.
Funding Statement: This research was funded by NSFC (Grant 32071527).
Author Contributions: The authors confirm contribution to the paper as follows: study conception and design: Fei-Hai Yu, Jin-Song Chen; data collection: Xiao-Mei Zhang, Yu Jin; analysis and interpretation of results: Xiao-Mei Zhang, Wei Xue, Jun-Qin Gao, Ning-Fei Lei; draft manuscript preparation: Xiao-Mei Zhang, Fei-Hai Yu.
Conflicts of Interest: The authors declare that they have no conflicts of interest to report regarding the present study.
References
1. Mirouze, M., Paszkowski, J. (2011). Epigenetic contribution to stress adaptation in plants. Current Opinion in Plant Biology, 14(3), 267–274. https://doi.org/10.1016/j.pbi.2011.03.004 [Google Scholar] [PubMed] [CrossRef]
2. Thiebaut, F., Hemerly, A. S., Ferreira, P. C. G. (2019). A role for epigenetic regulation in the adaptation and stress responses of non-model plants. Frontiers in Plant Science, 10, 246. https://doi.org/10.3389/fpls.2019.00246 [Google Scholar] [PubMed] [CrossRef]
3. Germain, R. M., Caruso, C. M., Maherali, H. (2013). Mechanisms and consequences of water stress-induced parental effects in an invasive annual grass. International Journal of Plant Sciences, 174(6), 886–895. https://doi.org/10.1086/670691 [Google Scholar] [CrossRef]
4. Zas, R., Cendan, C., Sampedro, L. (2013). Mediation of seed provisioning in the transmission of environmental maternal effects in Maritime pine (Pinus pinaster Aiton). Heredity, 111(3), 248–255. https://doi.org/10.1038/hdy.2013.44 [Google Scholar] [PubMed] [CrossRef]
5. González, A. P. R., Dumalasová, V., Rosenthal, J., Skuhrovec, J., Latzel, V. (2016). The role of transgenerational effects in adaptation of clonal offspring of white clover (Trifolium repens) to drought and herbivory. Evolutionary Ecology, 31(3), 345–361. https://doi.org/10.1007/s10682-016-9844-5 [Google Scholar] [CrossRef]
6. Badyaev, A. V., Uller, T. (2009). Parental effects in ecology and evolution: Mechanisms, processes and implications. Philosophical Transactions of the Royal Society B: Biological Sciences, 364(1520), 1169–1177. https://doi.org/10.1098/rstb.2008.0302 [Google Scholar] [PubMed] [CrossRef]
7. Dechaine, J. M., Brock, M. T., Weinig, C. (2015). Maternal environmental effects of competition influence evolutionary potential in rapeseed (Brassica rapa). Evolutionary Ecology, 29(1), 77–91. https://doi.org/10.1007/s10682-014-9735-6 [Google Scholar] [CrossRef]
8. Baker, B. H., Berg, L. J., Sultan, S. E. (2018). Context-dependent developmental effects of parental shade versus sun are mediated by DNA methylation. Frontiers in Plant Science, 9, 1251. https://doi.org/10.3389/fpls.2018.01251 [Google Scholar] [PubMed] [CrossRef]
9. Dong, B. C., Alpert, P., Yu, F. H. (2019). Transgenerational effects of herbivory and soil nutrients transmitted via vegetative reproduction in the clonal plant Alternanthera philoxeroides. Perspectives in Plant Ecology Evolution and Systematics, 41, 125498. https://doi.org/10.1016/j.ppees.2019.125498 [Google Scholar] [CrossRef]
10. Dong, B. C., Yu, F. H., Roiloa, S. R. (2019). Editorial: Ecoepigenetics in clonal and inbreeding plants: Transgenerational adaptation and environmental variation. Frontiers in Plant Science, 10, 622. https://doi.org/10.3389/fpls.2019.00622 [Google Scholar] [CrossRef]
11. Charpentier, A., Anand, M., Bauch, C. T. (2015). Variable offspring size as an adaptation to environmental heterogeneity in a clonal plant species: Integrating experimental and modelling approaches. Journal of Ecology, 100(1), 184–195. https://doi.org/10.1111/j.1365-2745.2011.01899.x [Google Scholar] [CrossRef]
12. Aarssen, L. W., Burton, S. M. (1990). Maternal effects at four levels in Senecio vulgaris (Asteraceae) grown on a soil nutrient gradient. American Journal of Botany, 77(9), 1231–1240. https://doi.org/10.1002/j.1537-2197.1990.tb13622.x [Google Scholar] [CrossRef]
13. Suter, L., Widmer, A. (2013). Environmental heat and salt stress induce transgenerational phenotypic changes in Arabidopsis thaliana. PLoS One, 8(4), e60364. https://doi.org/10.1371/journal.pone.0060364 [Google Scholar] [PubMed] [CrossRef]
14. Dong, B. C., Fu, T., Luo, F. L., Yu, F. H. (2017). Herbivory-induced maternal effects on growth and defense traits in the clonal species Alternanthera philoxeroides. Science of the Total Environment, 605–606, 114–123. https://doi.org/10.1016/j.scitotenv.2017.06.141 [Google Scholar] [PubMed] [CrossRef]
15. Vivas, M., Rolo, V., Wingfield, M. J., Slippers, B. (2019). Maternal environment regulates morphological and physiological traits in Eucalyptus grandis. Forest Ecology and Management, 432, 631–636. https://doi.org/10.1016/j.foreco.2018.10.016 [Google Scholar] [CrossRef]
16. Dong, B. C., Meng, J., Yu, F. H. (2019). Effects of parental light environment on growth and morphological responses of clonal offspring. Plant Biology, 21, 1083–1089. https://doi.org/10.1111/plb.13001 [Google Scholar] [PubMed] [CrossRef]
17. Song, Y. B., Yu, F. H., Keser, L. H., Dawson, W., Fischer, M. et al. (2013). United we stand, divided we fall: A meta-analysis of experiments on clonal integration and its relationship to invasiveness. Oecologia, 171(2), 317–327. https://doi.org/10.1007/s00442-012-2430-9 [Google Scholar] [PubMed] [CrossRef]
18. Douhovnikoff, V., Hazelton, E. L. (2014). Clonal growth: Invasion or stability? A comparative study of clonal architecture and diversity in native and introduced lineages of Phragmites australis (Poaceae). American Journal of Botany, 101(9), 1577–1584. https://doi.org/10.3732/ajb.1400177 [Google Scholar] [PubMed] [CrossRef]
19. Wang, Y. J., Muller-Scharer, H., van Kleunen, M., Cai, A. M., Zhang, P. et al. (2017). Invasive alien plants benefit more from clonal integration in heterogeneous environments than natives. New Phytologist, 216(4), 1072–1078. https://doi.org/10.1111/nph.14820 [Google Scholar] [PubMed] [CrossRef]
20. He, L. X., Xiao, X., Zhang, X. M., Jin, Y., Pu, Z. H. et al. (2021). Clonal fragments of stoloniferous invasive plants benefit more from stolon storage than their congeneric native species. Flora, 281, 151877. https://doi.org/10.1016/j.flora.2021.151877 [Google Scholar] [CrossRef]
21. Klimeš, L., Klimešova, J., Hendriks, R., van Groenendael, J. (1997). Clonal plant architecture: A comparative analysis of form and function. In: Kroon, H., Groenendael, J. (Eds.The ecology and evolution of clonal plants, pp. 1–29. Leiden: Backhuys Press. [Google Scholar]
22. Klimešova, J., Mudrák, O., Martinková, J., Lisner, A., Lepš, J. et al. (2021). Are belowground clonal traits good predictors of ecosystem functioning in temperate grasslands? Functional Ecology, 35(3), 787–795. https://doi.org/10.1111/1365-2435.13755 [Google Scholar] [CrossRef]
23. Dietz, H., Khler, A., Ullmann, I. (2002). Regeneration growth of the invasive clonal forb Rorippa austriaca (Brassicaceae) in relation to fertilization and interspecific competition. Plant Ecology, 158(2), 171–182. https://doi.org/10.1023/A:1015567316004 [Google Scholar] [CrossRef]
24. Weber, E. (2011). Strong regeneration ability from rhizome fragments in two invasive clonal plants (Solidago canadensis and S. gigantea). Biological Invasions, 13(12), 2947–2955. https://doi.org/10.1007/s10530-011-9977-y [Google Scholar] [CrossRef]
25. Zhang, L. M., Roiloa, S. R., Xue, W., Yu, F. H. (2022). Effects of temporal heterogeneity in nutrient supply on intra- and inter-genet competition of a clonal herb. Global Ecology and Conservation, 35, e02076. https://doi.org/10.1016/j.gecco.2022.e02076 [Google Scholar] [CrossRef]
26. Wang, M. Z., Li, H. L., Li, J. M., Yu, F. H. (2020). Correlations between genetic, epigenetic and phenotypic variation of an introduced clonal herb. Heredity, 124(1), 146–155. https://doi.org/10.1038/s41437-019-0261-8 [Google Scholar] [PubMed] [CrossRef]
27. Paszkowski, J., Grossniklaus, U. (2011). Selected aspects of transgenerational epigenetic inheritance and resetting in plants. Current Opinion in Plant Biology, 14(2), 195–203. https://doi.org/10.1016/j.pbi.2011.01.002 [Google Scholar] [PubMed] [CrossRef]
28. Latzel, V., Klimešová, J. (2010). Transgenerational plasticity in clonal plants. Evolutionary Ecology, 24(6), 1537–1543. https://doi.org/10.1007/s10682-010-9385-2 [Google Scholar] [CrossRef]
29. Heard, E., Martienssen, R. A. (2014). Transgenerational epigenetic inheritance: Myths and mechanisms. Cell, 157(1), 95–109. https://doi.org/10.1016/j.cell.2014.02.045 [Google Scholar] [PubMed] [CrossRef]
30. Douhovnikoff, V., Dodd, R. S. (2015). Epigenetics: A potential mechanism for clonal plant success. Plant Ecology, 216(2), 227–233. https://doi.org/10.1007/s11258-014-0430-z [Google Scholar] [CrossRef]
31. Huber, M., Gablenz, S., Hofer, M. (2021). Transgenerational non-genetic inheritance has fitness costs and benefits under recurring stress in the clonal duckweed Spirodela polyrhiza. Proceedings of the Royal Society B: Biological Sciences, 288(1955), 20211269. https://doi.org/10.1098/rspb.2021.1269 [Google Scholar] [PubMed] [CrossRef]
32. Portela, R., Dong, B. C., Yu, F. H., Barreiro, R., Roiloa, S. R. et al. (2020). Trans-generational effects in the clonal invader Alternanthera philoxeroides. Journal of Plant Ecology, 13(1), 122–129. https://doi.org/10.1093/jpe/rtz043 [Google Scholar] [CrossRef]
33. Donohue, K. (2009). Completing the cycle: Maternal effects as the missing link in plant life histories. Philosophical Transactions of the Royal Society B: Biological Sciences, 364(1520), 1059–1074. https://doi.org/10.1098/rstb.2008.0291 [Google Scholar] [PubMed] [CrossRef]
34. Wolf, J. B., Wade, M. J. (2009). What are maternal effects (and what are they not)? Philosophical Transactions of the Royal Society B: Biological Sciences, 364(1520), 1107–1115. https://doi.org/10.1098/rstb.2008.0238 [Google Scholar] [PubMed] [CrossRef]
35. Li, C., Wang, T., Zhang, M., Xu, J. (2018). Maternal environment effect of warming and eutrophication on the emergence of curled pondweed, Potamogeton crispus L. Water, 10(9), 1285. https://doi.org/10.3390/w10091285 [Google Scholar] [CrossRef]
36. Piqueras, J., Klimeš, L., Redbo-Torstensson, P. (1999). Modelling the morphological response to nutrient availability in the clonal plant Trientalis europaea L. Plant Ecology, 141(1/2), 117–127. https://doi.org/10.1023/A:1009845014687 [Google Scholar] [CrossRef]
37. Bam, S., Ott, J. P., Butler, J. L., Xu, L. (2022). Belowground mechanism reveals climate change impacts on invasive clonal plant establishment. Scientific Reports, 12, 2860. https://doi.org/10.1038/s41598-022-06918-w [Google Scholar] [PubMed] [CrossRef]
38. Adomako, M. O., Alpert, P., Du, D. L., Yu, F. H. (2021). Effects of fragmentation of clones compound over vegetative generations in the floating plant Pistia stratiotes. Annals of Botany, 127(1), 123–133. https://doi.org/10.1093/aob/mcaa150 [Google Scholar] [PubMed] [CrossRef]
39. Zhang, L. M., Roiloa, S. R., Zhang, J. F., Yu, W. H., Qiu, C. Y. et al. (2022). Clonal parental effects on offspring growth of different vegetative generations in the aquatic plant Pistia stratiotes. Frontiers in Plant Science, 13, 890309. https://doi.org/10.3389/fpls.2022.890309 [Google Scholar] [PubMed] [CrossRef]
40. Dong, B. C., van Kleunen, M., Yu, F. H. (2018). Context-dependent parental effects on clonal offspring performance. Frontiers in Plant Science, 9, 1824. https://doi.org/10.3389/fpls.2018.01824 [Google Scholar] [PubMed] [CrossRef]
41. Rendina Gonzalez, A. P., Preite, V., Verhoeven, K. J. F., Latzel, V. (2018). Transgenerational effects and epigenetic memory in the clonal plant Trifolium repens. Frontiers in Plant Science, 9, 1677. https://doi.org/10.3389/fpls.2018.01677 [Google Scholar] [PubMed] [CrossRef]
42. Shi, W., Chen, X., Gao, L., Xu, C. Y., Ou, X. et al. (2018). Transient stability of epigenetic population differentiation in a clonal invader. Frontiers in Plant Science, 9, 1851. https://doi.org/10.3389/fpls.2018.01851 [Google Scholar] [PubMed] [CrossRef]
43. Latzel, V., Janecek, S., Dolezal, J., Klimešova, J., Bossdorf, O. (2014). Adaptive transgenerational plasticity in the perennial Plantago lanceolata. Oikos, 123(1), 41–46. https://doi.org/10.1111/j.1600-0706.2013.00537.x [Google Scholar] [CrossRef]
44. Zhang, L. M., Jin, Y., Yao, S. M., Lei, N. F., Chen, J. S. et al. (2020). Growth and morphological responses of duckweed to clonal fragmentation, nutrient availability, and population density. Frontiers in Plant Science, 11, 618. https://doi.org/10.3389/fpls.2020.00618 [Google Scholar] [PubMed] [CrossRef]
45. Jin, Y., Zhang, Q., Zhang, L. M., Lei, N. F., Chen, J. S. et al. (2021). Distinct responses of frond and root to increasing nutrient availability in a floating clonal plant. PLoS One, 16(10), e0258253. https://doi.org/10.1371/journal.pone.0258253 [Google Scholar] [PubMed] [CrossRef]
46. Jin, Y., Chen, J. S., Luo, F. L., Huang, L., Lei, N. F. et al. (2022). Effects of descendent phenotypic diversity mediated by ancestor environmental variation on population productivity of a clonal plant. Diversity, 14(8), 616. https://doi.org/10.3390/d14080616 [Google Scholar] [CrossRef]
47. Luo, F. L., Wang, M. Z., Dong, B. C., Chen, Y. H., Yu, F. H. (2022). Clonal and sexual parental effects and their mechanisms. Acta Ecologica Sinica, 42(16), 6474–6486. https://doi.org/10.5846/stxb202107211968 [Google Scholar] [CrossRef]
48. Scheffer, M., Szabo, S., Gragnani, A., Van Nes, E. H., Rinaldi, S. et al. (2003). Floating plant dominance as a stable state. Proceedings of the National Academy of Sciences, 100(7), 4040–4045. https://doi.org/10.1073/pnas.0737918100 [Google Scholar] [PubMed] [CrossRef]
49. Li, K. N., Chen, J. S., Wei, Q., Li, Q., Lei, N. F. (2018). Effects of transgenerational plasticity on morphological and physiological properties of stoloniferous herb Centella asiatica subjected to high/low light. Frontiers in Plant Science, 9, 1640. https://doi.org/10.3389/fpls.2018.01640 [Google Scholar] [PubMed] [CrossRef]
50. Puy, J., de Bello, F., Dvorakova, H., Medina, N. G., Latzel, V. et al. (2021). Competition-induced transgenerational plasticity influences competitive interactions and leaf decomposition of offspring. New Phytologist, 229(6), 3497–3507. https://doi.org/10.1111/nph.17037 [Google Scholar] [PubMed] [CrossRef]
51. Shama, L. N., Wegner, K. M. (2014). Grandparental effects in marine sticklebacks: Transgenerational plasticity across multiple generations. Journal of Evolutionary Biology, 27(11), 2297–2307. https://doi.org/10.1111/jeb.12490 [Google Scholar] [PubMed] [CrossRef]
52. Jeremias, G., Barbosa, J., Marques, S. M., De Schamphelaere, K. A. C., Van Nieuwerburgh, F. et al. (2018). Transgenerational inheritance of DNA hypomethylation in Daphnia magna in response to salinity stress. Environmental Science & Technology, 52(17), 10114–10123. https://doi.org/10.1021/acs.est.8b03225 [Google Scholar] [PubMed] [CrossRef]
53. Richards, C. L., Alonso, C., Becker, C., Bossdorf, O., Bucher, E. et al. (2017). Ecological plant epigenetics: Evidence from model and non-model species, and the way forward. Ecology Letters, 20(12), 1576–1590. https://doi.org/10.1111/ele.12858 [Google Scholar] [PubMed] [CrossRef]
54. Herman, J. J., Sultan, S. E. (2011). Adaptive transgenerational plasticity in plants: Case studies, mechanisms, and implications for natural populations. Frontiers in Plant Science, 2, 102. https://doi.org/10.3389/fpls.2011.00102 [Google Scholar] [PubMed] [CrossRef]
55. Roach, D. A., Wulff, R. D. (1987). Maternal effects in plants. Annual Review of Ecology and Systematics, 18(1), 209–235. https://doi.org/10.1146/annurev.es.18.110187.001233 [Google Scholar] [CrossRef]
Cite This Article
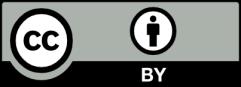
This work is licensed under a Creative Commons Attribution 4.0 International License , which permits unrestricted use, distribution, and reproduction in any medium, provided the original work is properly cited.