Open Access
ARTICLE
Response of Contrasting Rice Genotypes to Zinc Sources under Saline Conditions
1 Department of Soil and Environmental Sciences, Ghazi University, Dera Ghazi Khan, 32200, Pakistan
2 Institute of Soil and Environmental Sciences, University of Agriculture, Faisalabad, 38040, Pakistan
3 Department of Agronomy, University of Agriculture, Faisalabad, 38040, Pakistan
4 College of Agronomy, Northwest A&F University, Yangling, 712100, China
5 Pesticide Quality Control Lab, Multan, Punjab, 59300, Pakistan
6 Barani Agricultural Research Institute, Chakwal, 48800, Pakistan
7 Soil and Water Testing Laboratory for Research, Dera Ghazi Khan, 32200, Pakistan
8 Agronomic Research Institute, Ayub Agricultural Research Institute, Faisalabad, 38000, Pakistan
9 Department of Plant Sciences, Quaid-i-Azam University, Islamabad, 45320, Pakistan
10 Soil Fertility (Field), Multan, 59300, Pakistan
11 Department of Biology, College of Science, Princess Nourah bint Abdulrahman University, P.O.Box 84428, Riyadh, 11671, Saudi Arabia
12 Queensland University of Technology, Queensland, Australia
* Corresponding Authors: Muhammad Jan. Email: ; Sadam Hussain. Email:
(This article belongs to the Special Issue: Agricultural Intensification, Climate Change, and Food Security)
Phyton-International Journal of Experimental Botany 2023, 92(5), 1361-1375. https://doi.org/10.32604/phyton.2023.026620
Received 16 September 2022; Accepted 24 November 2022; Issue published 09 March 2023
Abstract
Abiotic stresses are among the major limiting factors for plant growth and crop productivity. Among these, salinity is one of the major risk factors for plant growth and development in arid to semi-arid regions. Cultivation of salt tolerant crop genotypes is one of the imperative approaches to meet the food demand for increasing population. The current experiment was carried out to access the performance of different rice genotypes under salinity stress and Zinc (Zn) sources. Four rice genotypes were grown in a pot experiment and were exposed to salinity stress (7 dS m−1), and Zn (15 mg kg−1 soil) was applied from two sources, ZnSO4 and Zn-EDTA. A control of both salinity and Zn was kept for comparison. Results showed that based on the biomass accumulation and K+/Na+ ratio, KSK-133 and BAS-198 emerged as salt tolerant and salt sensitive, respectively. Similarly, based on the Zn concentration, BAS-2000 was reported as Zn-in-efficient while IR-6 was a Zn-efficient genotype. Our results also revealed that plant growth, relative water content (RWC), physiological attributes including chlorophyll contents, ionic concentrations in straw and grains of all rice genotypes were decreased under salinity stress. However, salt tolerant and Zn-in-efficient rice genotypes showed significantly higher shoot K+ and Zn concentrations under saline conditions. Zinc application significantly alleviates the harmful effects of salinity by improving morpho-physiological attributes and enhancing antioxidant enzyme activities, and the uptake of K and Zn. The beneficial effect of Zn was more pronounced in salt-tolerant and Zn in-efficient rice genotypes as compared with salt-sensitive and Zn-efficient genotypes. In sum, our results confirmed that Zn application increased overall plant’s performance under saline conditions, particularly in Zn in-efficient and tolerant genotypes as compared with salt-sensitive and Zn efficient rice genotypes.Keywords
In plant functioning, Zn plays an essential role in protein synthesis and other metabolic events [1,2]. It has been reported that about 10% of the proteins in biological systems need Zn for their structural and functional integrity [3]. This element has also been indicated to be required as a cofactor in over 300 enzymes [4]. Under salinity stress, the production of reactive oxygen species (ROS) is well known [5–7] and the role of Zn in the detoxification of ROS in plant cells has been well demonstrated in previous studies [1,2]. In addition to being essential to plants, Zn is also a vital mineral nutrient for human beings. According to an estimate, more than 30% of the world population is affected by Zn deficiency which is associated with low dietary intake. Zinc deficiency is known to have serious adverse impacts on human health, especially in children, such as impairments in physical growth, immune system, and learning ability, and causing DNA damage and cancer development [8,9]. Increasing the Zn concentration of stable food crops is, therefore, an important humanitarian challenge.
The development of salt tolerance among cereal crops over the area of land is an important goal to feed the world’s increasing population [10,11]. Since, agricultural land is in decreasing trend, thus increasing yield per unit area is so important to meet the food demand and ensure global food security. Desertification and salinization sites are also increasing due to uneven rainfall distribution and the unavailability of freshwater for irrigation [12]. Salinity is among the major problems for plant cultivation with high occurrence in semiarid areas [13,14]. According to an estimate, about 800 million hectares of global land are affected by salt-alkalinization [15]. Furthermore, compared with ZnSO4, various responses of rice cultivars to different Zn sources such as ZnO, zinc-coated urea, Zn phosphate, and Zn-ethylenediaminetetraacetate (Zn-EDTA) have been well established in previous studies [16–18]. For soil application, both inorganic and organic Zn sources are commonly recommended. Although soil application of organic Zn sources including Zn-EDTA is more efficient than inorganic sources (such as ZnSO4) for rice crop [19], the prohibitively higher cost of Zn-EDTA deters its extensive use.
Cereal crops provide a major source of Zn for humans, especially those living in resource-poor areas. However, Zn contents of cereal-based foods are quite inadequate to meet per capita demand. The problem is especially acute for rice consumers due to the lowest Zn content in this crop when compared with other cereals [20]. Considerable variation in brown rice Zn has been found among different rice genotypes. According to a recent report by International Rice Research Institute, rice genotypes have average Zn content of 25.4 mg kg−1 grain, as compared with 35.0 mg Zn kg−1 in wheat (Triticum aestivum L.). Furthermore, rice-cultivated soils are distinguished with very low Zn concentrations which further, in turn, reduce Zn concentrations in grains [21,22]. Previous studies have reported that about 30% of the world’s cultivated soils are Zn deficient, of which, 50% of the soils are under cereal cultivation [23,24]. Several strategies such as conventional breeding, fertilizer management, seed treatments, and fortification have been suggested to improve Zn concentration in grains [22,25]. Rice genotypes respond differentially under Zn deficit soils [26–28], but the role of this genetic variation for Zn deficiency tolerance in grain Zn accumulation is not well studied. For wheat crop, it has been well established that variable genotype response is associated with the accumulation and expression of Zn-responsive genes [29]. As previously reported by Cakmak et al. [29], there is even an inverse relationship between high Zn-deficiency tolerance and grain Zn accumulation. Probably, grain Zn concentrations are diluted due to the higher grain yield capacity of the genotypes showing higher tolerance to soil Zn deficiency.
Keeping in view the above-discussed gaps, and beneficial effects of Zn on plant growth, a study was planned to (i) check the response of various rice cultivars to Zn sources, (ii) and evaluate the effectiveness of Zn sources in alleviating the adverse effects of salinity in different rice genotypes. Understanding morphological, physiological, and biochemical attribute changes in rice genotypes differing in salinity tolerance at different growth stages under various levels of Zn application and salinity stress is imperative for the efficient management of available resources. In this work, we hypothesized that Zn application could increase the plant performance under saline conditions by showing better growth of Zn in-efficient and tolerant genotypes relative to salt-sensitive and Zn efficient rice genotypes.
Before starting the actual experiment, sufficient numbers of seeds of four selected genotypes were sown in zinc-deficient soil where standing water conditions were maintained for a month. After that, uniform and healthy seedlings were selected and transplanted into the pots in which three seedlings were transplanted in each pot. The experiment was undertaken in a wire-house located at the Institute of Soil and Environmental Sciences, University of Agriculture Faisalabad.
Pots were arranged according to a completely randomized design and each treatment was replicated four times. Well-sieved soil, collected from the experimental field of the department, was used for pot filling where 12 kg of soil was used in each unit. Salinity (7 dSm−1) was established by considering a 50% economic threshold limit for rice crop, according to the standard protocol of Naralen et al. [30]. NaCl salt was used for salinity treatments. The calculated doses of NaCl salt and Zn from both sources (ZnSO4 and Zn-EDTA; 15 mg kg−1 soil of each source) were applied in the respective treated pots at the time of pot filling. A control without salt and Zn was kept for comparison. The recommended dose of N, P, and K (160, 90 and 60 kg ha−1, and 0.08, 0.04 and 0.03 g kg−1 soil, respectively) were fulfilled from urea, DAP and SOP. Full P and K and half N were applied on the 10th day after transplanting while the remaining N was divided into two equal splits and applied at 45 days after transplanting and the panicle initiation stage. All management practices were the same for all replicated pots.
Data on plant growth and physiological indices including the number of tillers per plant, the number of branches per panicle, plant height, and chlorophyll contents were determined at the physiological maturity stage. The collected samples were separated into different organs, straw, and panicles, and ionic concentrations of Na+ and K+ and Zn were determined. After measuring fresh weights, the collected seedling samples were oven dried at 75°C for 48 h, and their dry weights (DWs) were recorded. After grounding, the dried samples were digested in a di-acid (HNO3:HClO4 ratio of 2:1) mixture, according to the prescribed protocol. The ionic concentration of Na+ and K+ was determined by using Sherwood-410 Flame Photometer, for that, self-prepared standard solutions prepared from reagent grade salt of NaCl and KCl, respectively, were used. The Zn concentration in the digest was estimated by an atomic absorption spectrophotometer (PerkinElmer, 100 Analyst, Waltham, USA).
Relative water contents were determined according to the protocol of Sairam et al. [31]. Fresh leaves samples were soaked in water for 4 h, immediately after removal, turgid leaves were quickly dried with filter paper to remove surface water and immediately weighed to obtain a fully turgid weight (TW). These samples were oven dried at 65°C for 48 h to determine the dry weight. Relative water contents were determined by the following formula:
RWC=(FW−DW)(TW−DW) (1)
2.3.2 Agronomic and Physiological Efficiency
The physiological efficiency (PE), fertilizer recovery efficiency (RE), and agronomic efficiency (AE) were calculated according to the described method of Dobermann and Fairhurst [32], by using the below-described equations. Total Zn uptake by rice seedlings was referred to as Zn uptake by above-ground biomass (grain and straw) only.
RE=(Zn uptake by treated plants−Zn uptake by untreated plants)(Applied Zn to each treatment) (2)
PE=(GY of treated plants−GY under control)(Zn uptake by treated plants−Zn uptake under control) (3)
AE=(GY of treated plants−GY under control)(Applied Zn to each treatment) (4)
RE, Recovery efficiency (%); PE, Physiological efficiency; GY, Grain yield; AE, Agronomic efficiency
Recorded data on growth and yield and yield-related traits were subjected to MS excel for means calculation. The difference in mean values with control treatments was used for comparison. Graphs were prepared by using MS excel as well. A two-way ANOVA analysis technique was used for calculating the mean difference.
3.1 Plant Height and Agronomic Traits
The effect of salinity stress on rice growth and yield under different levels of Zn was examined in terms of plant height, the number of tillers per plant, and the number of branches per panicle (Fig. 1, Table 1). Salt stress caused a significant reduction in plant height, number of tillers per plant, and number of branches per panicle in all rice genotypes. Nonetheless, Zn application significantly (p < 0.05) increased these traits under non-saline as well as under salinity stress. However, there was a non-significant difference observed between the Zn sources. Among all genotypes, maximum plant height (79.89 cm), number of tillers per plant (11), and number of branches per panicle (10.22) were observed in rice genotype BAS-2000 while minimum plant height (62.67 cm), the number of tillers per plant (4) and number of branches per panicle (3.44) were observed BAS-198. Salinity stress induced a reduction in all these parameters with maximum reduction in BAS-198 relative to rice genotype KSK-133. Maximum values of these parameters were observed in the Zn-inefficient genotype (BAS-2000) and minimum in the salt-sensitive genotype (BAS-A98). However, Zn application increased all attributed in all rice genotypes while effective response towards Zn application was noted in rice genotype BAS-2000 (Table 2).
Figure 1: Influence of Zn sources (ZnSO4 and Zn-EDTA) and salinity stress on plant height, number of branches per plant, and number of tillers per plant of four rice genotypes. The values are the means of four replications. *p < 0.05
3.2 Chlorophyll and Relative Water Contents
Salt stress caused a significant reduction in chlorophyll and relative water contents in all rice genotypes where a prominent reduction was observed in BAS-198. However, the application of Zn increased these parameters under control as well as under saline conditions (Fig. 2). When compared the Zn sources, Zn-EDTA application increased the chlorophyll content and relative water content as compared to ZnSO4. Among rice genotypes, maximum chlorophyll content (44.20) and relative water content (77%) were observed for BAS-2000 while minimum chlorophyll content (27.41) and relative water content (33%) were observed for BAS-198. For interactive effect, maximum values were observed in the Zn-inefficient genotype (BAS-2000) and minimum in the salt-sensitive genotype (BAS-A98) (Fig. 2).
Figure 2: Influence of Zn sources (ZnSO4 and Zn-EDTA) and salinity stress on chlorophyll and relative water contents of four rice genotypes. The values are the means of four replications. *p < 0.05
Salt stress caused a significant reduction in grain and straw yield in all rice genotypes. Nonetheless, Zn application significantly increased the grain and straw yields under control as well as under saline conditions with higher values under control conditions (Fig. 3). The application of Zn increased these parameters under control and saline conditions. When compared the Zn sources, the Zn-EDTA application significantly increased the values of than ZnSO4 application. Among the genotypes, maximum grain yield (35.67 g) and straw yield (70.03 g) were observed in BAS-2000 while minimum grain yield (14.63 g) and straw yield (23.86 g) were observed in BAS-198. Salinity stress caused a significant reduction in BAS-198 followed by other genotypes. For their interactive influence, higher values were observed in the Zn-inefficient genotype (BAS-2000) and minimum in salt-sensitive genotype (BAS-98) (Fig. 3).
Figure 3: Influence of Zn sources and salinity stress on straw and grain yield of four rice genotypes. The values are the means of four replications. *p < 0.05
Salt treatment markedly increased Na+ and decreased K+ concentration in straw and grains of all rice genotypes (Fig. 4). Results showed that the extent of increased Na+ and decreased K+ concentrations were greater in rice genotype BAS-198 than in other genotypes under saline and control conditions. The maximum shoot Na+ concentration was recorded in rice genotype BAS-198 under salinity stress while the minimum was recorded in BAS-2000 under high Zn conditions (15 mg kg−1). An opposite trend was recorded for K+ concentration (Fig. 4). Rice genotype KSK-133 markedly sustained ionic concentrations than other genotypes under saline conditions. Results also showed that Zn significantly enhanced K+ concentration and reduced Na+ concentration in grains under both non-saline and saline conditions. Zn application at 15 mg kg−1 was found most effective in improving K+ concentrations in all rice genotypes. And, a better response to Zn application was observed in rice genotypes BAS-2000 under non-saline and KSK-133 under saline conditions.
Figure 4: Influence of Zn sources (ZnSO4 and Zn-EDTA) and salinity stress on straw and grain yield of four rice genotypes. The values are the means of four replications. *p < 0.05
Zn application significantly increased Zn concentration in both straw and grain under non-saline conditions compared to saline conditions in all rice genotypes (Fig. 5). Results showed that the extent of increase in shoot Zn concentration was greater in rice genotype BAS-2000 than in other genotypes under both saline and non-saline conditions. The maximum Zn concentration was recorded in rice genotype BAS-2000 when treated with Zn-EDTA while the minimum Zn concentration was recorded in rice genotype IR-6. Results regarding the uptake of Zn demonstrated that higher uptake of Zn was observed in straw while minimum uptake of Zn was observed in grains of all rice genotypes. Among the genotypes, KSK-133 recorded significantly higher values followed by BAS-2000 and BAS-198 under saline conditions. Zn application at 15 mg kg−1 was found most effective in improving Zn concentrations in all rice genotypes. However, a better response to Zn application was observed in rice genotypes BAS-2000 under non-saline and KSK-133 under saline conditions.
Figure 5: Influence of Zn sources (ZnSO4 and Zn-EDTA) and salinity stress on straw and grain yield of four rice genotypes. The values are the means of four replications. *p < 0.05
3.6 Effect of Zn Application on Zn Use Efficiencies
In this work, agronomic efficiency, apparent recovery efficiency, and physiological efficiency were evaluated for Zinc sources and cultivars. Zinc application significantly increased physiological efficiency, fertilizer recovery efficiency, and agronomic efficiency in all four rice genotypes (Table 3). Among cultivars, KSK-133 recorded higher values of these parameters followed by BAS-198. The maximum physiological efficiency (12,937.14 μg μg−1), fertilizer recovery efficiency (5.54 μg μg−1), and agronomic efficiency (361.05 μg μg−1) were recorded in rice genotype BAS-2000 treated with Zn-EDTA at 15 mg kg−1 while minimum physiological efficiency (6,178.32 μg μg−1), fertilizer recovery efficiency (1.15 μg μg−1) and agronomic efficiency (171.30 μg μg−1) were recorded in IR-6.
The genetic variations between the crop plants provide a precious tool in the selection of genotypes with desirable characteristics [33]. The present study, clearly demonstrated the differential response of four rice genotypes subjected to two Zn sources and salt stress. It is obvious that salinity stress reduced plant growth in terms of reduced RWC, chlorophyll content, agronomic traits, yield attributes and altered K+/Na+ ratios and Zn concentrations in four rice genotypes [34–37]. However, the addition of Zn significantly alleviated the harmful effects of salinity by improving growth parameters, Zn concentrations, and K+/Na+ ratios in four rice genotypes, as reported in previous studies [38–42]. The data also indicated that rice genotype BAS-2000 was relatively Zn-inefficient and showed a better response to Zn application as compared to IR-6 which was characterized as Zn-efficient under non-saline and saline conditions.
The results of the present work revealed that salinity caused a significant reduction in plant height, chlorophyll content, relative water content, and straw and grain yield in four rice genotypes. These results are in agreement with those of [34–37] who reported that salinity caused a reduction in plant growth and yield-related traits. This reduction in plant growth might be due to the ionic toxicity or decreased osmotic potential as well as low wall extensibility [38]. There are several reports on osmotic stress and ionic toxicity resulting from salt stress in rice genotypes [40–42]. The addition of Zn significantly reduced the lethal effects of NaCl and improved plant growth in rice genotypes. This was certified to the antagonistic effect of Zn with Na+. Similarly, the enhancement in plant growth and dry matter production was reported in rice by the addition of Zn in saline soil [43,44].
The level of salt-induced effects on RWC has been used as one of the imperative water relations attributes for assessing the degree of salt tolerance in rice [37], Brassica rapa [45], and pea [46]. In this work, salinity caused a significant reduction in RWC in all rice genotypes. Salt-tolerant rice genotype KSK-133 and Zn-inefficient genotype BAS-2000 showed higher RWC under saline conditions as compared to salt-sensitive rice genotype (BAS-198) and Zn-efficient IR-6. The decreases in RWC under salinity stress in rice genotypes were confirmed by the previous study of Munns [47]. Zinc plays an important role in rice genotype’s water relation by increasing the membrane stability, water relations, and photosynthetic activity, and helps the plants to absorb more water to reach turgidity [23,48,49] in C3 and C4 plants.
It is very clear from the results that salt stress leads to a significant reduction in leaf chlorophyll contents in all rice genotypes. A higher reduction in chlorophyll contents was observed in rice genotype BAS-198 relative to rice genotype KSK-133. These results are inconsistent with some earlier reports that showed a reduction in chlorophyll content in rice and wheat [50]. The decrease in chlorophyll content and leaf area due to the roots of some species, such as rice, being leaky and Na+ may be taken up apoplastically and salt concentration build-up in the apoplast and caused the dehydration of cells or disorder of chloroplast structure and associated proteins [51]. In rice, lower plant available Zn in soil results in causes leaf bronzing and poor tillering at the early growth stages, leading to delayed maturity and significant yield loss [32,52]. Zinc plays a key role in various plant metabolism processes, i.e., the development of cell walls, respiration, carbohydrate metabolism and gene expression and regulation [53]. Micronutrients also enhance plant productivity, leaf area and grain yield as a result of enhancing the enzymatic system of plants [54].
Salinity stress results in high accumulation of sodium (Na+) in rice genotype BAS-198 which could be one of the major reasons for its sensitivity to salt stress, while more K content in the case of rice genotype KSK-133 must have contributed towards its discriminating tolerance to salinity stress. The higher K+ uptake in rice genotype KSK-133 may be related to its selectivity of K+ over Na+. In another study, Zhu et al. [55] also demonstrated that salt-tolerant barley plants accumulated higher K+ due to selective absorption of K+ and by a preferential loading of K+ rather than Na+ into the xylem. However, salt-tolerant rice genotype exhibited a strong affinity for K+ over Na+ by maintaining a higher K+/Na+ ratio as compared to salt-sensitive rice genotype. Working with rice and barley crops, Carden et al. [56] reported that Zn application repressed Na+ transport in plants grown in salinized conditions, with concomitant improvement in plant growth. It was suggested that the plant’s tolerance response is characterized by a distinctly higher K+/Na+ ratio, which may be used as an indicator of tolerance or sensitivity in crop varieties [57]. Thus, it appears that the ideal rice genotype should possess not only the capability to retain K+ efficiently in plant roots under saline conditions but also a means of preventing Na+ accumulation in the shoot. Thus, further breeding efforts should be rigorous in identifying, characterizing, and localizing the genes encoding these two traits in the rice genome. Comparing the sources, in the case of the pot experiment, contradictory results were observed between ZnSO4 and Zn-EDTA.
In conclusion, salinity stress reduced plant growth by affecting plant agronomic traits, and yield attributes, reducing relative water contents and chlorophyll contents, decreasing Zn concentrations, and altering K+/Na+ ratios and efficiencies in all rice genotypes. The inhibitory effect of salt stress was more pronounced for rice genotype BAS-198 than KSK-133. However, the addition of Zn significantly alleviates the harmful effects of salinity by improving plant growth, photosynthetic and enzymatic activities, and enhancing Zn concentrations and K+/Na+ ratios in all rice genotypes. Zinc application (15 mg kg−1) was found to be more effective in alleviating the perilous effect of salinity compared to Zn deficient and saline conditions. Salt tolerant rice genotype (KSK-133) and Zn-inefficient genotype (BAS-2000) produced more biomass, less shoot Na+ concentrations, high shoot K+ concentrations, high Zn concentrations, and exhibited more chlorophyll contents and relative water content under salt stress and Zn sufficient conditions compared to salt-sensitive rice genotype (BAS-198) and Zn-efficient genotype (IR-6). Using resistant rice cultivars is the topic of our oncoming research under field conditions. These results from local varieties can offer ways to better understanding the effect of Zn sources on salinity tolerance and help us to select genotypes that can maintain salinity tolerance under variable Zn sources.
Funding Statement: This research was funded by Princess Nourah bint Abdulrahman University, Researchers Supporting Project Number (PNURSP2023R188), Riyadh, Saudi Arabia.
Author Contributions: Study conception and design: Muhammad Jan, Muhammad Anwar-Ul-Haq; data collection: Muhammad Jan, Sadam Hussain; analysis and interpretation of results: Muhammad Jan, Sadam Hussain, Muhammad Ashraf Sumrah; Formal analysis: Talha Javed, Ilyas Ahmad, Javed Iqbal, Muhammad Aslam, Izhar Ullah, Aqsa Hafeez; draft manuscript preparation: Muhmmad Jan, Sadam Hussain, Muhammad Tahir Akbar, Khadija Alharbi, Izhar Ullah, Marjan Aziz; Funding acquisition: Khadija Alharbi, Izhar Ullah. All authors reviewed the results and approved the final version of the manuscript.
Conflicts of Interest: The authors declare that they have no conflicts of interest to report regarding the present study.
References
1. Cakmak, I. (2000). Possible roles of zinc in protecting plant cells from damage by reactive oxygen species. New Phytology, 14(6), 185–205. [Google Scholar]
2. Zafar, M., Ahmed, S., Munir, M. K., Zafar, N., Saqib, M. et al. (2023). Application of zinc, iron and boron enhances productivity and grain biofortification of mungbean. Phyton-International Journal of Experimental Botany, 92(4), 983–999. [Google Scholar]
3. Andreini, C., Banci, L., Bertini, I., Rosato, A. (2006). Counting the zinc proteins encoded in the human genome. Journal of Proteome Resources, 4(5), 196–201. [Google Scholar]
4. Lee, S., Jeong, H. J., Kim, S. A., Lee, J., Guerinot, M. L. et al. (2010). OsZIP5 is a plasma membrane zinc transporter in rice. Plant Molecular Biology, 73(4), 507–517. [Google Scholar] [PubMed]
5. Cakmak, I., Marschner, H. (1988). Increase in membrane permeability and exudation in roots of Zn deficient plants. Journal of Plant Physiology, 132(3), 356–361. [Google Scholar]
6. Bailly, C., El-Maarouf-Bouteau, H., Corbineau, F. O. (2008). From intracellular signaling networks to cell death: The dual role of reactive oxygen species in seed physiology. Comptes Rendus Biologies, 3(31), 806–814. [Google Scholar]
7. Qin, J., Dong, W. Y., He, K. N., Yu, Y., Tan, G. D. et al. (2010). NaCl salinity-induced changes in water status, ion contents and photosynthetic properties of Shepherdia argentea (Pursh) Nutt. seedlings. Plant, Soil and Environment, 56(7), 325–332. [Google Scholar]
8. Keen, C. L., Gershwin, M. E. (1990). Zinc deficiency and immune function. Annual Review of Nutrition, 10(1), 415–431. [Google Scholar] [PubMed]
9. Black, R. E., Allen, L. H., Bhutta, Z. A., Caulfield, L. E., Deonis, M. et al. (2008). Maternal and child undernutrition: Global and regional exposures and health consequences. The Lancet, 371(9608), 243–260. [Google Scholar]
10. Shabbir, R., Singhal, R. K., Mishra, U. N., Chauhan, J., Javed, T. et al. (2022). Combined abiotic stresses: Challenges and potential for crop improvement. Agronomy, 12(11), 2795. [Google Scholar]
11. Rengasamy, P. (2006). World salinization with emphasis on Australia. Journal of Experimental Botany, 57(5), 1017–1023. https://doi.org/10.1093/jxb/erj108 [Google Scholar] [PubMed] [CrossRef]
12. Mahmood, U., Hussain, S., Hussain, S., Ali, B., Ashraf, U. et al. (2021). Morpho-physio-biochemical and molecular responses of maize hybrids to salinity and waterlogging during stress and recovery phase. Plants, 10(7), 1345. https://doi.org/10.3390/plants10071345 [Google Scholar] [PubMed] [CrossRef]
13. Hussain, S., Hussain, S., Ali, B., Ren, X., Chen, X. et al. (2021). Recent progress in understanding salinity tolerance in plants: Story of Na+/K+ balance and beyond. Plant Physiology and Biochemistry, 160(7), 239–256. https://doi.org/10.1016/j.plaphy.2021.01.029 [Google Scholar] [PubMed] [CrossRef]
14. Naz, M., Hussain, S., Ashraf, I., Farooq, M. (2022). Exogenous application of proline and phosphorus help improving maize performance under salt stress. Journal of Plant Nutrition, 40, 1–9. https://doi.org/10.1080/01904167.2022.2155541 [Google Scholar] [CrossRef]
15. Ray, S. K., Varadachari, C., Ghosh, K. (1993). Novel slow-releasing micronutrient fertilizers. 1. Zinc compounds. Industrial and Engineering Chemistry Research, 32(6), 1218–1227. https://doi.org/10.1021/ie00018a030 [Google Scholar] [CrossRef]
16. Chatterjee., A. K., Mandal., L. N. (1985). Zinc sources for rice in soil at different moisture regimes and organic matter levels. Plant and Soil, 87(2), 393–404. https://doi.org/10.1007/BF02181906 [Google Scholar] [CrossRef]
17. Kang, B. T., Okoro, E. G. (1976). Response of flooded rice grown on a vertisol from northern Nigeria to zinc sources and methods of application. Plant and Soil, 44(1), 15–25. https://doi.org/10.1007/BF00016951 [Google Scholar] [CrossRef]
18. Srivastava, A. K., Poi, S. C., Basu, T. K. (1992). Effect of chelated and non chelated zinc on growth and yield of rice. Indian Agriculturist, 36(1), 45–48. [Google Scholar]
19. Boawn, L. C. (1973). Comparison of zinc sulphate and Zn EDTA as zinc fertilizer sources. Soil Science Society of American Proceeding, 37(2), 111–115. https://doi.org/10.2136/sssaj1973.03615995003700010034x [Google Scholar] [CrossRef]
20. Juliano, B. O. (1993). Rice in human nutrition. In: Prepared in collaboration with FAO, Food and Agriculture Organization of the United Nations. Rome, Italy: Viale delle Terme di Caracalla. [Google Scholar]
21. Alloway, B. J. (2008). Zinc in soils and crop nutrition, 2nd editionParis, France: International Fertilizer Industry Association. [Google Scholar]
22. Cakmak, I. (2008). Enrichment of cereal grains with zinc, Agronomic or genetic biofortification. Plant Soil, 302(1–2), 1–17. https://doi.org/10.1007/s11104-007-9466-3 [Google Scholar] [CrossRef]
23. Graham, R., Senadhira, D., Beebe, S., Iglesias, C., Monasterio, I. (1999). Breeding for micronutrient density in edible portions of staple food crops. Conventional Plant Physiology, 106, 71–77. [Google Scholar]
24. Welch, R. M. (1993). Zinc concentrations and forms in plants for humans and animals. In: Robson, A. D. (Ed.) Zinc in soils and plants, pp. 183–195. Dordrecht, Netherlands: Springer. [Google Scholar]
25. Ahmad, N., Virk, A. L., Hussain, S., Hafeez, M. B., Haider, F. U. et al. (2022). Integrated application of plant bioregulator and micronutrients improves crop physiology, productivity and grain biofortification of delayed sown wheat. Environmental Science and Pollution Research, 29, 52534–52543. [Google Scholar] [PubMed]
26. Hoffland, E., Wei, C., Wissuwa, M. (2006). Organic anion exudation by lowland rice (Oryza sativa L.) at zinc and phosphorus deficiency. Plant and Soil, 283(1), 155–162. [Google Scholar]
27. Wissuwa, M., Ismail, A. M., Yanagihara, S. (2006). Effects of zinc deficiency on rice growth and genetic factors contributing to tolerance. Plant Physiology, 142(2), 731–741. [Google Scholar] [PubMed]
28. Gao, X. P., Zou, C. Q., Fan, X. Y., Zhang, F. S., Ellis, H. (2005). From flooded to aerobic conditions in rice cultivation: Consequences for zinc uptake. Plant and Soil, 280(1), 41–47. [Google Scholar]
29. Cakmak, I., Torun, A., Millet, E., Feldman, M., Fahima, A. et al. (2004). Triticum dicoccoides. An important genetic resource for increasing zinc and iron concentration in modern cultivated wheat. Soil Science and Plant Nutrition, 50(1), 1047–1054. [Google Scholar]
30. Narale, R. P., Subramanyam, T. K., Mukherjee, R. K. (1969). Influence of salinity on germination, vegetative growth, and grain yield of rice (Oryza sativa var. Dular). Agronomy Journal, 61(3), 341–344. [Google Scholar]
31. Sairam, R. K., Rao, K. V., Srivastava, G. C. (2002). Differential response of wheat genotypes to long term salinity stress in relation to oxidative stress, antioxidant activity and osmolyte concentration. Plant Science, 163(5), 1037–1046. [Google Scholar]
32. Dobermann, A., Fairhurst, F. (2000). Rice: nutritional disorders and nutrient management. Potash and Phosphate Institute and Potash & Phosphate Institute of Canada (PPI/PPIC) and International Rice Research Institute (IRRI), Singapore and Makati City, The Philippines. [Google Scholar]
33. Misra, N., Dwivedi, U. N. (2004). Genotypic difference in salinity tolerance of green gram cultivars. Plant Science, 166(5), 1135–1142. [Google Scholar]
34. Habib, N., Akram, M. S., Javed, M. T., Azeem, M., Ali, Q. et al. (2016). Nitric oxide regulated improvement in growth and yield of rice plants grown under salinity stress: Antioxidant defense system. Applied Ecological Environmental Resource, 14(5), 91–105. [Google Scholar]
35. Arif, M., Shehzad, M., Bashir, F., Tasneem1, M., Yasin, G. et al. (2012). Boron, zinc and microtone effects on growth, chlorophyll contents and yield attributes in rice (Oryza sativa L.) cultivar. African Journal of Biotechnology, 11(48), 10851–10858. [Google Scholar]
36. Yadi, R., Dastan, S., Yasari, E. (2012). Role of zinc fertilizer on grain yield and some qualities parameters in Iranian rice genotypes. Annals of Biological Research, 3(9), 4519–4527. [Google Scholar]
37. Suriya-arunroj, D., Supapoj, N., Toojinda, T., Vanavichit, A. (2004). Relative leaf water content as an efficient method for evaluating rice cultivars for tolerance to salt stress. Science Asia, 30, 411–415. [Google Scholar]
38. Grieve, C. M., Francois, L. E., Poss, J. A. (2001). Effect of salt stress during early seedling growth on phenology and yield of spring wheat. Cereal Research Communications, 29(1), 167–174. [Google Scholar]
39. Malik, N. J., Chamon, A. S., Mondol, M. N., Elahi, S. F., Faiz, S. M. A. (2011). Effects of different levels of zinc on growth and yield of red amaranth (Amaranthus sp.) and rice (Oryza sativa, Variety-BR49). Journal of the Bangladesh Association of Young Researchers, 1(1), 79–91. [Google Scholar]
40. Amirjani, M. R. (2010). Effect of NaCl on some physiological parameters of rice. European Journal of Agricultural Sciences, 39(1), 6–16. [Google Scholar]
41. Hossain, M. A., Hannan, M. A., Talukder, N. M., Hanif, M. A. (2008). Effect of different rates and methods of zinc application on the yield and nutritional qualities of rice cv. BR11. Journal of Agroforestry and Environment, 2(1), 1–6. [Google Scholar]
42. Roshandel, P., Flowers, T. (2009). The ionic effects of NaCl on physiology and gene expression in rice genotypes differing in salt tolerance. Plant and Soil, 315(1), 135–147. https://doi.org/10.1007/s11104-008-9738-6 [Google Scholar] [CrossRef]
43. Verma, T. S., Neue, H. U. (1984). Effect of soil salinity level and zinc application on growth, yield, and nutrient composition of rice. Plant and Soil, 82(1), 3–14. https://doi.org/10.1007/BF02220765 [Google Scholar] [CrossRef]
44. Arshad, A., Arshadullah, M., Mahmood, I. A., Hyder, S. I., Zaman, B. (2011). Response of rice to Zn application under salt affected soil in Hafizabad District. Pakistan Pakistan Journal of Biological Science, 54(2), 111–113. [Google Scholar]
45. Zayed, B. A., Salem, A. K. M., El Sharkawy, H. M. (2011). Effect of different micronutrient treatments on rice (Oryza sativa L.) growth and yield under saline soil conditions. World Journal of Agricultural Sciences, 7(2), 179–184. [Google Scholar]
46. Noreen, Z., Ashraf, M., Akram, N. A. (2010). Salt-induced regulation of some key antioxidant enzymes and physio-biochemical phenomena in five diverse cultivars of turnip (Brassica rapa L.). Journal of Agronomy and Crop Science, 196(4), 273–285. [Google Scholar]
47. Noreen, Z., Ashraf, M. (2009). Assessment of variation in antioxidative defense system in salt-treated pea (Pisum sativum) cultivars and its putative use as salinity tolerance markers. Journal of Plant Physiology, 166(16), 1764–1774. [Google Scholar] [PubMed]
48. Munns, R. (2002). Comparative physiology of salt and water stress. Plant Cell and Environment, 25, 239–250. [Google Scholar]
49. Brown., P. H., Cakmak, I., Zhang, Q. (1993). Form and function of zinc in plants. In: Zinc in soils and plants, pp. 90–106. Dordrecht: Kluwer Academic Publishers. [Google Scholar]
50. Marschner, H. (1995). Mineral nutrition of higher plants, 2nd editionLondon: Academic Press. [Google Scholar]
51. Shahbaz, M., Ashraf, M., Athar, H. U. R. (2008). Does exogenous application of 24-epibrassinolide ameliorate salt induced growth inhibition in wheat (Triticum aestivum L.)? Plant Growth Regulation, 55(1), 51–64. [Google Scholar]
52. Gong, H. J., Randall, D. P., Flowers, T. J. (2006). Silicon deposition in the root reduces sodium uptake in rice (Oryza sativa L.) seedlings by reducing bypass flow. Plant Cell and Environment, 29(3), 1970–1979. [Google Scholar]
53. Neue, H. U., Quijano, C., Senadhira, D., Setter, T. (1998). Strategies for dealing with micronutrient disorders and salinity in lowland rice systems. Field Crops Research, 56(1–2), 139–155. [Google Scholar]
54. Klug, A., Rhodes, D. (1987). Zinc fingers’: A novel protein motif for nucleic acid recognition. Trends in Biochemical Sciences, 12, 464–469. [Google Scholar]
55. Zhu, J. K. (2002). Salt and drought stress signal transduction in plants. Annual Review of Plant Biology, 53, 247–273. [Google Scholar] [PubMed]
56. Carden, D. E., Walker, D. J., Flowers, J. T., Miller, A. J. (2003). Single-cell measurements of the contributions of cytosolic Na+ and K+ to salt tolerance. Plant Physiology, 13(1), 676–683. [Google Scholar]
57. Aslam, M. A., Ranjha, A. M., Akhtar, J. (2000). Salinity tolerance of rice affected by zinc application. Pakistan Journal of Biological Sciences, 3(1), 2055–2057. [Google Scholar]
Cite This Article
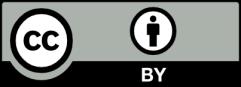
This work is licensed under a Creative Commons Attribution 4.0 International License , which permits unrestricted use, distribution, and reproduction in any medium, provided the original work is properly cited.