Open Access
ARTICLE
Subcellular Distribution and Chemical Forms of Cadmium in the Medicine Food Homology Plant Platycodon grandiflorum (Jacq.) A.DC.
College of Pharmacy, Shaanxi University of Chinese Medicine, Xianyang, 712046, China
* Corresponding Authors: Wenli Huang. Email: ; Yonggang Yan. Email:
(This article belongs to the Special Issue: Development of New Sensing Technology in Sustainable Farming and Smart Environmental Monitoring)
Phyton-International Journal of Experimental Botany 2023, 92(5), 1405-1420. https://doi.org/10.32604/phyton.2023.026525
Received 10 September 2022; Accepted 28 November 2022; Issue published 09 March 2023
Abstract
Although Platycodon grandiflorum (Jacq.) A.DC. is a renowned medicine food homology plant, reports of excessive cadmium (Cd) levels are common, which affects its safety for clinical use and food consumption. To enable its Cd levels to be regulated or reduced, it is necessary to first elucidate the mechanism of Cd uptake and accumulation in the plant, in addition to its detoxification mechanisms. This present study used inductively couple plasma-mass-spectrometry to analyze the subcellular distribution and chemical forms of Cd in different tissues of P. grandiflorum. The experimental results showed that Cd was mainly accumulated in the roots [predominantly in the cell wall (50.96%–61.42%)], and it was found primarily in hypomobile and hypotoxic forms. The proportion of Cd in the soluble fraction increased after Cd exposure, and the proportion of insoluble phosphate Cd and oxalate Cd increased in roots and leaves, with a higher increase in oxalate Cd. Therefore, it is likely that root retention mechanisms, cell wall deposition, vacuole sequestration, and the formation of low mobility and low toxicity forms are tolerance strategies for Cd detoxification used by P. grandiflorum. The results of this study provide a theoretical grounding for the study of Cd accumulation and detoxification mechanisms in P. grandiflorum, and they can be used as a reference for developing Cd limits and standards for other medicine food homology plants.Keywords
Supplementary Material
Supplementary Material FileHeavy metals are naturally occurring elements that are widely distributed throughout the Earth’s crust. However, recent industrialization and agricultural development have resulted in heavy metal pollution, which is a major public health concern [1]. These mineral elements normally enter the food chain via plant uptake and are progressively passed on to the final consumers, thereby leading to various health problems [2,3]. Of all the heavy metals, cadmium (Cd) is a particular cause for concern because high amounts of Cd are emitted from industries, and plants readily uptake the metal via their roots [4]. Cd is reported to be a carcinogenic substance, and long-term exposure to Cd can have adverse effects on human health; toxic effects on the lungs, kidneys, bones, and respiratory system have been reported, in addition to hypertension and cardiovascular, cerebrovascular, and kidney diseases [5–7].
Cd can be rapidly spread in the environment from various industrial sources, such as metal smelting, mining, battery manufacturing, textile printing, and pigment and plastic manufacturing [8,9]. Cd presents high mobility in soils and plants and readily accumulates at excess levels [10]. Exposure to Cd can interfere with all stages of plant development and inhibit seed germination and vegetative and reproductive growth [11]. Symptoms of Cd poisoning in plants include stunted growth, yellowing (leaf chlorosis), necrosis, wilting, a reduced photosynthetic rate, and respiratory depression. In addition, Cd can reduce plant biomass production and even result in plant death [12].
In the case of medicinal plants, heavy metals affect both their quality and safety for medicinal purposes [13], and numerous researchers have examined heavy metal contamination in plant tissues. For example, the study of Sarma et al. [14] assessed the accumulation of heavy metals in 88 medicinal plants, 44 of which showed Cd levels above the maximum permitted level (0.5 μg g−1) of the “European Pharmacopoeia”. Platycodon grandiflorum (Jacq.) A.DC. (P. grandiflorum) is an important plant used in Chinese traditional medicine [15], and it contains a variety of chemical constituents, such as saponins, flavonoids, phenolic acids, polysaccharides, and amino acids. These exert a wide range of pharmacological effects, and the plant is used as a cough suppressant, analgesic, and immunostimulant, and it is also known to have anti-inflammatory, antioxidant, antitumor, hepatoprotective, and anti-diabetic properties [16]. However, in addition to its medicinal use, P. grandiflorum has been used as a vegetable in east Asia for thousands of years [17], and both its seedlings and roots are edible and rich in amino acids, dietary fiber, vitamins, and essential trace elements, including calcium, zinc, potassium, and iron [18]. There is thus a broad market for P. grandiflorum in Korea, Japan, and Northeast China [19], where it is processed into pickles, salads, noodles, preserved fruits, and medicinal wine [17,20]. It is considered a “medicine food homology” plant and is included in the “Chinese Homologous Catalogue of Medicine and Food” [17].
In recent years, the public has become gradually aware of the benefits of healthy eating, and medicine food homology plants have become widely popular; therefore, the safety of such plants is paramount. However, numerous studies have reported the presence of excessive Cd levels in P. grandiflorum. For example, Li [21] analyzed the Cd contents of three batches of P. grandiflorum samples in Sichuan and found that two of the batches exceeded the Cd limit standard of 0.3 mg kg−1 established by the Green Trade Standards of Importing & Exporting Medicinal Plants & Preparations. Zhang [22] analyzed the Cd content of P. grandiflorum samples in the Yanbian area and found that the contents were higher than the national permissible Cd limit standard. Moreover, Zeng [23] analyzed the heavy metal content of 58 batches of P. grandiflorum samples from different areas in China; of these, 24 batches exceeded the Cd limit standard (>0.3 mg kg−1), with an unqualified rate of 41.4%, and the highest Cd content was found to be 1.365 mg kg−1. These studies show that excessive amounts of Cd beyond standard limits are commonly accumulated in P. grandiflorum. Related studies have also found that P. grandiflorum has a large Cd enrichment factor and the ability to actively absorb and enrich external Cd [24–26]. Therefore, its Cd contents severely affect the quality and safety of Platycodonis Radix, the root of P. grandiflorum. However, to design methods that control the Cd uptake of this important species, it is necessary to first gain a thorough understanding of its Cd accumulation and detoxification mechanisms.
The transport, toxicity, and biological validity of heavy metals are all closely related to their subcellular distribution and the chemical form in which they occur in plant tissues [27,28]. In addition, the subcellular distribution patterns and the chemical forms in which heavy metals exist in a particular plant’s tissues reflect its accumulation and detoxification processes [29,30]. Heavy metals can exist in different chemical forms in plants, including inorganic, water-soluble, pectate- and protein-integrated, undissolved phosphate, and oxalate forms [31], and converting heavy metals to non-toxic chemical forms is an important strategy used by plants to alleviate associated toxicity [32]. For example, the conversion of Cd into insoluble phosphate precipitates, pectates, and protein-bound forms is the primary means of reducing Cd mobility and toxicity in Koelreuteria paniculate (K. paniculate) [33]. To avoid Cd toxicity, plants have developed intracellular and extracellular mechanisms for metal detoxification, such as binding, precipitation in the cell wall, and/or compartmentalization in vacuoles [34,35]. In this respect, in Morus alba L. (M. alba), the combination of Cd and peptides and organo-ligands in the leaf vacuoles, or with proteins or cellulose in the cell walls of roots, may contribute to root tolerance of Cd stress [8]. Uddin et al. [4] proved that Cd in Sesuvium portulacastrum was primarily compartmentalized as a soluble fraction. However, in Solanum nigrum, the largest proportion of Cd was found to be segregated in the cell wall, and as the Cd concentration increased, the proportion of inorganic and water-soluble forms of Cd also increased, whereas insoluble Cd phosphates and Cd oxalate decreased [36].
Studying the subcellular distribution and chemical forms of Cd in P. grandiflorum is essential for revealing its Cd accumulation and detoxification mechanisms. However, little is known about the translocation, distribution, and chemical forms of Cd within P. grandiflorum. Therefore, to fill this knowledge gap, cell fractionation and chemical sequential extraction methods were used to investigate the subcellular distribution and chemical forms of Cd in P. grandiflorum. The results of this study suggest that root retention mechanisms, cell wall deposition, vacuole sequestration, and the formation of hypomobile and hypotoxic chemical forms are important Cd-detoxifying strategies in plants that accumulate excess Cd. Furthermore, these results can be used as a theoretical basis for understanding the accumulation and detoxification mechanism of Cd in P. grandiflorum, and they also provide valuable information for evaluating the safety of medicinal food homology plants.
2.1 Plant Material and Experimental Conditions
P. grandiflorum seedlings were collected from the medicinal plant garden at the Shaanxi University of Chinese Medicine. The seedlings were thoroughly washed and then grown hydroponically in Hoagland’s nutrient solution. After three months, they were exposed to concentrations of 0 μM (CK) and 200 μM Cd(NO3)2 Three replicates of each treatment were prepared, with 12 seedlings in each. Cd treatment concentrations arebased on preexperimental study results and described in the supplementary material. The plants were cultivated in a greenhouse with 12 h of light per day, a day/night temperature of 25/20°C, and the nutrient solution was renewed every 3 d. The plants were harvested after 0, 24 h, and 10 days of Cd exposure, and were noted as the Cd 0h group, Cd 24 h group, and the Cd 10 d group, respectively. The controls were recorded as the Cd 0 h-CK group, Cd 24 h-CK group, and Cd 10 d-CK group, respectively. When harvesting the plants, care was taken to keep the roots intact. The plants were then completely immersed in 20 mM Na2-EDTA for 20 min to eliminate Cd absorbed on the surface and subsequently washed with deionized water. Finally, the plants were divided into root, stem, and leaf tissues, all of which were then used for various research parameter assays.
Fresh samples of P. grandiflorum root, stem, and leaf tissues were frozen in liquid nitrogen and then crushed. The samples were homogenized in a pre-cooled extraction buffer (50 mM Tris-HCl, 250 mM sucrose, and 1.0 mM C4H10O2S2) and the homogenate was centrifuged at 3,000 rpm for 15 min, with the precipitate representing the cell wall fraction (FCW). The supernatant was taken and centrifuged at 12,000 rpm for 45 min, with the precipitate representing the organelle fraction (FCO) and the supernatant representing the soluble fraction (FS) [9]. All centrifugations were conducted at 4°C. The different fractions were digested with HNO3/H2O2 (3:1, v/v), and the Cd content of each fraction was determined using ICP-MS (iCAP RQ, Thermo Fisher Scientific, Bremen, Germany). ICP-MS measurement conditions and sample digestion methods are in the supplementary material.
2.3 Extraction of Different Cd Chemical-Forms
The chemical forms of Cd were analyzed using successive extraction by designated solutions in the following order: (1) 80% ethanol, extracting inorganic Cd (FE); (2) deionized water, extracting water-soluble organic Cd (FW); (3) 1 M NaCl, extracting pectate and protein-integrated Cd (FNaCl); (4) 2% acetic acid, extracting insoluble Cd-phosphate complexes (FHAc); (5) 0.6 M HCl, extracting Cd-oxalate (FHCl) and (6) the residual Cd (FR) [37]. The root, stem, and leaf tissues were frozen in liquid nitrogen and crushed, homogenized in extraction solution, diluted at a ratio of 1:10 (w/v) and shaken for 22 h at 25°C. The homogenate was then centrifuged at 5,000 g for 10 min, and the supernatant was collected. The precipitate was suspended twice in the extraction solution, shaken for 2 h at 25°C, and then centrifuged. The supernatants of the three centrifugations were pooled, and the solid residue was subjected to the next extraction in the indicated order using the same steps presented above. The five extraction solutions and the residue were dried to a constant weight, digested with HNO3/H2O2 (3:1, v/v), and the Cd content in each fraction was then determined using ICP-MS (iCAP RQ, Thermo Fisher Scientific, Bremen, Germany). ICP-MS measurement conditions and sample digestion methods are in the supplementary material
2.4 QA/QC Control and Statistical Analysis
Quality assurance and quality control (QA/QC) of Cd in P. grandiflorum was performed using the standard reference material of garlic powder (GBW10022) from the Institute of Geophysical and Geochemical Exploration in China, which had a Cd dry weight content of 0.0611 ± 0.0001 mg kg−1 that was consistent with the certified value of 0.062 ± 0.003 mg kg−1 Cd. SPSS statistical software (Version 25.0, SPSS Inc., Chicago, IL, USA) was used to analyze the data, and figures were generated using the GraphPad Prism 9 software (GraphPad Software, San Diego, CA, USA).
3.1 Cd Uptake in P. grandiflorum
Table 1 shows Cd concentration in leaves, stems and roots of P. grandiflorum at different sampling times after treatment initiation. TWO-WAY analysis of variance (3 types of tissues × 3 different times after initiation of the experiment) showed that that the interaction between different tissue types and sampling times had significant effects on Cd concentration (p < 0.01). In the Cd 0 h group, Cd concentrations in stems were significantly higher than those in leaves and roots. However, in the Cd 24 h group, Cd concentrations in roots were significantly higher than those in leaves and stems. In the Cd 10 d group, the concentrations of Cd in roots, stems and leaves were significantly different, with the highest concentration in roots, accounting for 60.89% of the total. The Cd concentrations in the same tissue were significantly different at different harvesting times. The Cd concentrations of the root and leaf tissues increased with increasing Cd exposure time, whereas it first decreased and then increased in the stem tissue. At the end of the experiment, the Cd levels in the root and leaf tissues of the Cd 24 h group increased by 3.2- and 11.3-fold, respectively, and Cd levels in the leaf, stem, and root tissues increased by 7.1-, 6.2-, and 50.8-fold in the Cd 10 d group, respectively.
The results of the subcellular distribution of Cd in different P. grandiflorum tissues after different Cd exposure times are shown in Fig. 1, Table S1 and Table 2. The results of the THREE-WAY analysis of variance (3 types of tissues × 3 subcellular fractions × 6 harvesting times) showed that different subcellular fractions had a significant effect on the proportion of Cd (p < 0.01), but the effect of different tissues and different sampling times on the proportion of Cd was not significant. Furthermore, the interaction effects between tissue type and subcellular distribution, sampling time and subcellular distribution, tissue type, subcellular fraction, and sampling time had a significant effect on the proportion of Cd (p < 0.01) (Table 3).
Figure 1: Subcellular distribution of Cd in root, stem, and leaf tissues of P. grandiflorum at different exposure times
In P. grandiflorum, the proportion of Cd in the cell wall (FCW) showed a trend of decreasing and then increasing with increasing exposure time, and the proportion of Cd in the cell wall was significantly lower than the other groups at 24 h exposure (p < 0.01), while there was no statistical difference between the other groups. The proportion of Cd in the organelles (FCO) decreased gradually with increasing exposure time and was significantly lower than that in the control group (p < 0.01). The proportion of Cd in soluble fraction (FS) showed a trend of increasing and then decreasing with increasing exposure time and was statistically different between the Cd 24 h group, Cd 10 d group and the control group (p < 0.05). At each harvest time, the cell wall contained the most Cd (50.96%–61.42%), followed by the organelles (26.91%–35.86%) and the soluble fraction (2.22%–22.13%), with statistical differences between the three subcellular fractions (p < 0.01) (Table 2).
In P. grandiflorum leaves, stems, and roots, Cd was predominantly distributed in the cell wall fraction, followed by the organelle and soluble fractions, and there was a statistical difference between the three subcellular fractions in the same tissue (p < 0.01) (Fig. 1). The proportion of Cd in the cell wall of the stem was significantly higher than the proportion of Cd in the cell wall of the leaves and roots (p < 0.05). There was no statistical difference in the proportion of Cd in organelles of leaves, stems and roots. The proportion of Cd in the soluble fraction of the stem was significantly lower than the proportion of Cd in the soluble fraction of the leaf and root (p < 0.05).
In addition, compared to the proportion of Cd in the soluble fraction of each tissue type in the control group of P. grandiflorum, the amounts increased significantly after 24 h and 10 d of Cd exposure (p < 0.01), with the leaf soluble fraction increasing by 2.3- and 1.2-fold, respectively, the stem soluble fraction increasing by 6.6- and 5.0-fold, respectively, and the root soluble fraction increasing 1.5- and 1.4-fold, respectively. However, compared to the control, the proportion of Cd in the organelle fraction decreased in both the Cd 24 h and Cd 10 d treatments in roots, stems and leaves of P. grandiflorum (Table 2).
The proportions and concentrations of each of the six chemical forms of Cd in the root, stem, and leaf tissues of P. grandiflorum at different exposure times is shown in Fig. 2, Table S2 and Table 4. The results of the THREE-WAY analysis of variance (3 types of tissues × 6 chemical forms × 6 harvesting times) indicated a significant effect of chemical form on the proportion of Cd (p < 0.01), while the effect of tissue type and harvesting time on the proportion of Cd was not significant. The interaction effects between tissue type and chemical form, harvesting time and chemical form, tissue type, chemical form, and harvesting time had a significant effect on the proportion of Cd (p < 0.01) (Table 5).
Figure 2: Chemical forms of Cd in root, stem, and leaf tissues of P. grandiflorum at different exposure times. The forms of Cd other than FR, FHCl and FHAc were found in negligle amounts. This is why they are not displayed in the figure
In P. grandiflorum, the proportion of pectate and protein-integrated Cd (FNaCl) showed a decreasing and then increasing trend with increasing Cd exposure time. The proportion of insoluble Cd phosphate (FHAc), on the other hand, showed an increasing and then decreasing trend. The proportion of Cd oxalate (FHCl) increased with increasing duration of Cd exposure and was significantly higher than that of the control group (p < 0.01). The proportion of the residual Cd (FR) decreased with increasing time of Cd exposure and was significantly lower than that of the control group (p < 0.01). At each harvest time, the residual Cd was significantly higher than the other chemical forms, accounting for 51.12%–80.69% of the total extraction of the six forms of Cd, followed by Cd oxalate (12.07%–37.16%) and insoluble Cd phosphate (6.66%–11.43%), while the other forms accounted for a negligible proportion of Cd (Table 4).
In the leaves of the P. grandiflorum, mainly the residual Cd was present, followed by Cd oxalate, insoluble Cd phosphate and pectate and protein-integrated Cd, while inorganic Cd (FE) and water-soluble Cd (FW) were not detected. In the stems of the P. grandiflorum, the proportions of different chemical forms of Cd were, in order, the residual Cd > Cd oxalate > insoluble Cd phosphate > pectate and protein-integrated Cd > water-soluble Cd > inorganic Cd. The proportions of water-soluble Cd, inorganic Cd and pectate and protein-integrated Cd were not statistically different, and the proportions of the residual Cd, Cd oxalate and insoluble Cd phosphate were statistically different (p < 0.01). The proportions of different chemical forms of Cd were in the same order in the roots of the P. grandiflorum as in the stems. However, only the proportions of the residual Cd and Cd oxalate were statistically different (p < 0.01), while the proportions of other chemical forms were not statistically different. Inorganic and water-soluble Cd were only detected in the stems and roots. The proportion of pectate and protein-integrated Cd was significantly higher in P. grandiflorum roots than in stems and leaves (p < 0.01). The proportions of insoluble Cd phosphate and Cd oxalate were significantly higher in the stems than in the leaves and roots of P. grandiflorum, while the proportions of residual Cd were significantly lower than in the leaves and roots (p < 0.01).
In addition, in leaf tissue, the proportions of Cd oxalate and insoluble Cd phosphate were significantly higher in the Cd 24 h and Cd 10 d groups than in the Cd 24 h-CK and Cd 10 d-CK groups, with proportions increasing by 2.4 and 2.1-fold, and 1.7 and 1.4-fold, respectively. In the root tissue, the proportion of Cd oxalate was higher in the Cd 24 h and Cd 10 d groups than in the control group, with their proportions increasing by 2.3 and 3.1-fold, respectively. The proportion of pectin- and protein-bound Cd in stems and insoluble Cd phosphate and Cd oxalate in roots increased with increasing Cd exposure time (Table 4).
4.1 Cd Accumulation in P. grandiflorum
The Cd contents of P. grandiflorum root tissues were higher than those in either the stem or leaf tissues, and they accounted for 60.89%–61.64% of the total Cd content of the entire plant. In addition, the Cd contents of the root tissues increased by up to 50.8-fold after the heavy metal stress treatment, and this increase was higher than that occurring in either stem or leaf tissues. These results imply that Cd preferentially accumulates in the roots of P. grandiflorum, and only small amounts are transferred to the stems and leaves. Roots are in direct contact with contaminated substrates and are the primary site for the uptake and accumulation of heavy metals in most plants [38], and a similar trend has been observed in plants such as Phragmites australis [39], K. paniculate [33], and Triarrhena sacchariflora (T. sacchariflora) [40]. Some plants accumulate large amounts Cd in the roots, thus reducing Cd toxicity in other sites. Dong et al. [41] showed that Canna indica L. tolerates Cd toxicity by sequestering Cd in root tissues; after exposure to Cd2+ for 45 d, the highest Cd concentration was observed in the root tissue, and this amount was 17- to 47-fold higher than that in the leaves, and 8-to 20-fold higher than that in the stem tissue. In addition, Huang et al. [8] reported that Cd complexing with root cell walls might contribute to Cd tolerance in M. alba L., as 53.27%–70.17% of accumulated Cd was found to be stored in the roots. These studies show that roots are an effective barrier to the transfer of Cd to the aboveground plant body parts [42], and reducing the impact of such heavy metals on the stems and leaves, which is a protective mechanism against heavy metal stress [43]. The results obtained here are thus in agreement with those of previous studies that the immobilization of large amounts of Cd in the roots of P. grandiflorum may be an important tolerance mechanism of this plant.
Once Cd enters a plant, it is selectively partitioned into subcellular components and bound to specific substances within the plant cells. Compartmentalization of toxic ions in different subcellular fractions is an important strategy used by plants to reduce toxicity [44]. Available studies have shown that plants generally store Cd through cell wall deposition and vacuole sequestration [29,36,45]. The experimental results obtained in this study revealed that Cd was mainly accumulated in the cell wall parts of the leaf, stem, and root tissues of P. grandiflorum (50.96%–61.42%; Fig. 1 and Table 2) and that Cd was mainly retained in the root cell walls. The cell wall is the first barrier protecting against heavy metal invasion into the cell and protects the protoplasm from heavy metal toxicity [46]. Heavy metal ions in the cell wall are usually in a bound state and are less toxic than those in the free state in the cytoplasm and organelles [47]. Similarly, Cd in Amaranthus hypochondriacus L. roots was found to be mainly distributed in the cell walls [48], and most of the Cd in K. paniculate was distributed in the cell wall fraction (45%–77%) [33]. Furthermore, in low- and high-Cd-accumulating wheat under Cd exposure, the subcellular distribution of Cd was primarily found in the cell wall [49], and the Cd content of subcellular components in the roots, stems, leaves, and fruits of three pepper varieties under different Cd treatments was reported to be the highest in cell walls [50]. The extent of heavy metal adsorption largely depends on the composition of the cell wall, in which polysaccharides (including cellulose, hemicellulose, and pectin) and proteins are the major components that provide many functional groups (such as carboxyl, hydroxyl, amino, and aldehyde groups), which allow metal cations to bind naturally to the plant [51–53]. These ligands can be involved in various reactions, including ion exchange, uptake, complexation, precipitation, and crystallization [54,55]. Therefore, immobilization of heavy metals in the cell wall can reduce the risk of heavy metal ions entering the protoplasts and can mitigate the effects of heavy metal stress on normal plant physiological activities to a certain extent, thus detoxifying plant tissues [56]. As mentioned earlier, most of the Cd was found to be distributed in the cell wall in this study, and this is likely to be one of the Cd-detoxification strategies of P. grandiflorum.
In addition to the cell wall, numerous studies have reported that vacuoles are important storage sites for heavy metals. Vacuoles can chelate and separate metal ions through the various metal ligands they contain (such as proteins, organic acids, and bases), reducing toxicity and protecting cells [52]. In this study, the proportion of Cd in soluble fractions (Fs) increased in the treated group compared to the control group by 1.4-fold to 6.6-fold (Fig. 1). The soluble fraction consists mainly of vacuoles, comprising up to 90% of the total cellular volume [57], and it is thus speculated that most of the Cd in P. grandiflorum enters the vacuoles after passing through the cell wall. This has been shown to occur in observations of Pistia stratiotes [58], and this strategy might further decrease the amount of Cd interfering with organelles. Previous reports have shown that the largest proportions of Cd in Agrocybe Aegerita [59], Phytolacca americana L. [45], and Sesuvium portulacastrum [4] were accumulated in the soluble fraction, and such results suggest that vacuoles are important Cd storage sites in these species. Similarly, in K. paniculata [33], Coptis chinensis Franch. [9], and Solanum nigrum [36], most of the Cd absorbed was found to be initially distributed in the cell wall fraction, but it later appeared to be distributed in the soluble fraction, which again suggests that vacuoles play an important role in alleviating Cd toxicity. It has been reported that there is a threshold for the barrier effect of cell walls on heavy metals and that heavy metals are transported to vacuoles when the amounts of heavy metals stored in the cell wall reaches saturation [60,61]. Therefore, it is speculated that Cd entering P. grandiflorum cells may first bind to the cell wall, and then if abundant Cd enters the cell under heavy metal stress, the vacuoles preferentially sequester the excess Cd, thus reducing its toxicity to the cell. The synergistic effect of cell wall deposition and vacuole sequestration may thus be an important mechanism for P. grandiflorum resisting Cd toxicity.
Heavy metals are absorbed by the roots and transported through the plant body to different organs and tissues in different chemical forms, especially those which render them unavailable to limit their movement within plant tissues, thereby reducing their toxicity [41]. Heavy metals generally have multiple chemical forms that differ in their ecological effectiveness and toxicity [54]. In this study, Cd in P. grandiflorum was mainly present in the extraction residues and as Cd oxalate and insoluble Cd phosphate, each accounting for 51.12%–80.69%, 12.07%–30.8%, and 6.66%–11.43% of the total extractable Cd, respectively, which indicates that Cd in P. grandiflorum is mainly present in low-mobility and low-toxicity forms. In addition, the percentage of HCl- and Hac-extracted Cd increased after Cd stress; this implies that P. grandiflorum may combine Cd into the less bioavailable Cd oxalate and insoluble Cd phosphate, which reduces the amount of free Cd entering the cytoplasm. Similar results have also been found in other plants. In the leaves and stem of M. alba L., most of the Cd absorbed was extracted by 2% HAc and 0.6 M HCl [8]. Similarly, in barley roots, the Cd forms extracted by 2% HAc and 0.6 M HCl were predominant, and they represented 36% and 33% of the total Cd content, respectively [62]. It is therefore inferred that higher proportions of Cd present in the low-mobility and low-toxicity forms in P. grandiflorum are responsible for its adaptation to Cd-enriched soils.
The results obtained in this study showed that exposure to Cd increased the proportion of Cd extracted by 0.6 M HCl in the root and leaf tissues, and a maximum 3-fold increase was seen compared to control plants. This suggests that the detoxification reaction of P. grandiflorum may involve binding Cd to oxalate to produce insoluble Cd complexes. Oxalic acid is an organic acid, and the chelation of organic acids with heavy metals is also an important heavy metal-detoxification mechanism in plants [63]. A previous study consistently found that when the organic acid concentrations increased, there was a significant statistical correlation between the organic acid levels and the heavy metal concentrations in plants (p < 0.001), and this was presumed to occur because they assist in chelating heavy metals in plants [64]. Organic acids can chelate heavy metal cations through their carboxyl groups (-COOH) to form a complex, which reduces the damage caused by heavy metals to plants [65]. Oxalate is a strong dicarboxylic acid anion and a good complexing agent for the binding of heavy metal cations [66,67]. Consistently, in the Cd-tolerant Lycopersicon esculentum variety ‘Micro-Tom’, Cd-induced oxalate was secreted in high concentrations in the root apex, where most of the Cd was also accumulated [68]. Xin et al. [40] found that Cd mainly exists in oxalate complexes, where it exhibits low activity; this limits symplastic transport and suppresses toxicity in T. sacchariflora seedlings. Similarly, Funneliformis mosseae was found to promote the conversion of inorganic and water-soluble forms of Cd to Cd-oxalate in Sphagneticola calendulacea shoots to improve their Cd tolerance [69]. It is thus considered that in P. grandiflorum, Cd2+ is chelated with oxalic acid to increase plant tolerance to Cd. Moreover, it has been shown that the acidic environment of the vacuole provides a favorable environment for the formation of metalorganic acid complexes [70].
Cd occurs in multiple chemical forms, and as shown in this study, different extraction solvents only extract their corresponding Cd chemical forms. The toxicity of different Cd chemicals differs; therefore, the total Cd content in plants does not accurately reflect its toxicity [9]. During production, Chinese medicinal materials are generally extracted by ethanol or water and the residue is then discarded; ethanol is a common extraction solvent used in factories and water is a solvent commonly used to decoct medicine. In P. grandiflorum, inorganic Cd forms (extracted with 80% ethanol) and water-soluble forms (extracted with d-H2O) were not detected. It is of note that the amount of Cd transferred to the human body is not the total amount of Cd that existed in the Chinese medicinal materials, but it is the amount that was dissolved in the extraction reagents. Therefore, heavy metal limits in Chinese traditional medicines should be based on their chemical form, valence, and other such factors that are associated with their actual toxicity, so that reasonable scientific standard limits can be set for the amounts of heavy metals contained in Chinese traditional medicines. However, for vegetables, the amount and type of Cd contained in the vegetable parts consumed by people are representative of the toxicity of the heavy metal element. As P. grandiflorum is a plant used for both medicinal and food purposes, corresponding heavy metal limit standards should be set according to the plant’s use and the specific valence and chemical form of the metal involved. In this respect, heavy metal allowances should vary according to the different uses of each medicinal and vegetable plant species, particularly for medicine food homology plants.
The subcellular distribution and chemical forms of Cd present in the leaf, stem, and root tissues of P. grandiflorum was characterized using ICP-MS. The results provide evidence that enriches the understanding of Cd transport, accumulation, and detoxification in P. grandiflorum. The data suggest that root retention mechanisms, cell wall deposition, vacuole sequestration, and the formation of hypomobile and hypotoxic chemical forms are important Cd-detoxifying strategies in plants such as P. grandiflorum, which accumulate Cd in excess. Future studies should focus on studying the dynamic uptake of Cd and associated Cd transporters. The results of this study also provide a theoretical foundation for assessing the Cd status in other medicine food homology plant species, and they provide a solid theoretical foundation for establishing appropriate Cd concentration standard limits for medicine and food homology plants.
Funding Statement: This work was supported by the Major Science and Technology Projects in Inner Mongolia Autonomous Region (No. 2019ZD005), the National Natural Science Foundation of China (No. 81903751); and by the Natural Science Basic Research Project of Shaanxi Science and Technology Department (No. 2019JQ-877); and by the Scientific Research Project of Shaanxi Administration of Traditional Chinese Medicine (No. 2019-ZZ-ZY018).
Author Contributions: The authors confirm contribution to the paper as follows: study conception and design: WenLi Huang, Yonggang Yan; data collection: Xiang Wang, Jing Gao, Liang Peng, Gang Zhang; analysis and interpretation of results: Jia An, Yajiang Jing, Jianping Huang, Qilong Wang; draft manuscript preparation: Jia An. All authors reviewed the results and approved the final version of the manuscript.
Conflicts of Interest: The authors declare that they have no conflicts of interest to report regarding the present study.
References
1. Uddin, M. M., Xie, B., Peng, G., Huang, L. (2020). Heavy metal pollution status, spatial distribution and associated ecological risks within sediments of Yundang Lagoon catchment in Xiamen, China, after 30 years continuous ecological rehabilitation and management. Human and Ecological Risk Assessment: An International Journal, 27(2), 465–482. https://doi.org/10.1080/10807039.2020.1731679 [Google Scholar] [CrossRef]
2. Aprile, A., de Bellis, L. (2020). Editorial for special issue “Heavy metals accumulation, toxicity, and detoxification in plants”. International Journal of Molecular Sciences, 21(11), 4103. https://doi.org/10.3390/ijms21114103 [Google Scholar] [PubMed] [CrossRef]
3. Fang, T., Lu, W., Cui, K., Li, J., Yang, K. et al. (2019). Distribution, bioaccumulation and trophic transfer of trace metals in the food web of Chaohu Lake, Anhui, China. Chemosphere, 218, 1122–1130. https://doi.org/10.1016/j.chemosphere.2018.10.107 [Google Scholar] [PubMed] [CrossRef]
4. Uddin, M. M., Chen, Z., Huang, L. (2020). Cadmium accumulation, subcellular distribution and chemical fractionation in hydroponically grown Sesuvium portulacastrum [Aizoaceae]. PLoS One, 15(12), e0244085. https://doi.org/10.1371/journal.pone.0244085 [Google Scholar] [PubMed] [CrossRef]
5. Guo, H., Jiang, J., Gao, J., Zhang, J., Zeng, L. et al. (2020). Evaluation of cadmium hyperaccumulation and tolerance potential of Myriophyllum aquaticum. Ecotoxicology and Environmental Safety, 195(17), 110502. https://doi.org/10.1016/j.ecoenv.2020.110502 [Google Scholar] [PubMed] [CrossRef]
6. Huang, G., Ding, C., Guo, F., Li, X., Zhou, Z. (2017). The role of node restriction on cadmium accumulation in the brown rice of 12 Chinese rice (Oryza sativa L.) cultivars. Journal of Agricultural and Food Chemistry, 65(47), 10157–10164. https://doi.org/10.1021/acs.jafc.7b03333 [Google Scholar] [PubMed] [CrossRef]
7. Oono, Y., Yazawa, T., Kawahara, Y., Kanamori, H., Kobayashi, F. (2014). Genome-wide transcriptome analysis reveals that cadmium stress signaling controls the expression of genes in drought stress signal pathways in rice. PLoS One, 9(5), e96946. https://doi.org/10.1371/journal.pone.0096946 [Google Scholar] [PubMed] [CrossRef]
8. Huang, R. Z., Jiang, Y. B., Jia, C. H., Jiang, S. M., Yan, X. P. (2018). Subcellular distribution and chemical forms of cadmium in Morus alba L. International Journal of Phytoremediation, 20(5), 448–453. https://doi.org/10.1080/15226514.2017.1365344 [Google Scholar] [PubMed] [CrossRef]
9. Huang, W., Bai, Z., Jiao, J., Yuan, H., Bao, Z. (2019). Distribution and chemical forms of cadmium in Coptis chinensis Franch. determined by laser ablation ICP-MS, cell fractionation, and sequential extraction. Ecotoxicology and Environmental Safety, 171, 894–903. https://doi.org/10.1016/j.ecoenv.2018.10.034 [Google Scholar] [PubMed] [CrossRef]
10. Li, J., Yu, J., Yan, C., Du, D., Liu, J. et al. (2018). Distribution correlations of cadmium to calcium, phosphorus, sodium and chloridion in mangrove Aegiceras corniculatum root tissues. Marine Pollution Bulletin, 126, 179–183. https://doi.org/10.1016/j.marpolbul.2017.10.074 [Google Scholar] [PubMed] [CrossRef]
11. Huybrechts, M., Cuypers, A., Deckers, J., Iven, V., Vandionant, S. et al. (2019). Cadmium and plant development: An agony from seed to seed. International Journal of Molecular Sciences, 20(16), 3971. https://doi.org/10.3390/ijms20163971 [Google Scholar] [PubMed] [CrossRef]
12. Shaari, N. E. M., Tajudin, M., Khandaker, M. M., Majrashi, A., Alenazi, M. M. et al. (2022). Cadmium toxicity symptoms and uptake mechanism in plants: A review. Brazilian Journal of Biology, 84(1), e252143. https://doi.org/10.1590/1519-6984.252143 [Google Scholar] [PubMed] [CrossRef]
13. Gyamfi, E. T. (2019). Metals and metalloids in traditional medicines (Ayurvedic medicines, nutraceuticals and traditional Chinese medicines). Environmental Science and Pollution Research, 26(16), 15767–15778. https://doi.org/10.1007/s11356-019-05023-2 [Google Scholar] [PubMed] [CrossRef]
14. Sarma, H., Deka, S., Deka, H., Saikia, R. R. (2011). Accumulation of heavy metals in selected medicinal plants. Reviews of Environmental Contamination and Toxicology, 214, 63–86. https://doi.org/10.1007/978-1-4614-0668-6_4 [Google Scholar] [PubMed] [CrossRef]
15. Nan, G., Meng, X., Song, N., Liu, Z., Liu, Y. et al. (2021). Uptake and distribution characteristic and health risk assessment of heavy metal(loid)s in Platycodon grandiflorum (Jacq.) A.DC. with growth from a medicinal herb garden of Xi’an, China. Biological Trace Element Research, 199(7), 2770–2778. https://doi.org/10.1007/s12011-020-02364-9 [Google Scholar] [PubMed] [CrossRef]
16. Huang, W., Zhou, H., Yuan, M., Lan, L., Hou, A. et al. (2021). Comprehensive characterization of the chemical constituents in Platycodon grandiflorum by an integrated liquid chromatography-mass spectrometry strategy. Journal of Chromatography A, 1654, 462477. https://doi.org/10.1016/j.chroma.2021.462477 [Google Scholar] [PubMed] [CrossRef]
17. Ji, M. Y., Bo, A., Yang, M., Xu, J. F., Jiang, L. L. et al. (2020). The pharmacological effects and health benefits of Platycodon grandiflorus–A medicine food homology species. Foods, 9(2), 142. https://doi.org/10.3390/foods9020142 [Google Scholar] [PubMed] [CrossRef]
18. Wang, D., Shu, Y., Zhao, X. L., Jing, L. I. (2018). Innovation and development value of medicinal, edible and appreciate Platycodon grandiflorum. Heilongjiang Agricultural Sciences, 2018(11), 112–115. [Google Scholar]
19. Zhang, S., Chai, X., Hou, G., Zhao, F., Meng, Q. (2022). Platycodon grandiflorum (Jacq.) A.DC.: A review of phytochemistry, pharmacology, toxicology and traditional use. Phytomedicine, 106, 154422. https://doi.org/10.1016/j.phymed.2022.154422 [Google Scholar] [PubMed] [CrossRef]
20. Lu, H. Y., Peng, H. S., Gui, S. Y., Peng, D. Y. (2017). Evolution and transition of quality evaluation of Platycodon grandiflorum. Zhongguo Zhong Yao Za Zhi, 42(9), 1637–1640. https://doi.org/10.19540/j.cnki.cjcmm.20170121.033 [Google Scholar] [PubMed] [CrossRef]
21. Li, X. Y. (2018). Study on the quality of platycodonis radix in Sichuan province (Master Thesis). Chengdu University of Chinese Medicine, China. [Google Scholar]
22. Zhang, E. X. (2019). Study on the correlation between the contents of lead cadmium and total arsenic in wild vegetables in Yanbian (Master Thesis). Yanbian University, China. [Google Scholar]
23. Zeng, J. K. (2018). Study on the basic state data of Platycodon grandiflorum quality (Master Thesis). Nanjing University of Chinese Medicine, China. [Google Scholar]
24. Meng, C. Y., Gao, Z. J., Liu, Y. L., Qiang, L. I., Li, J. W. et al. (2019). Enrichment ability and health risk assessment of 9 elements in soil and Platycodon grandiflorum. Modern Preventive Medicine, 46(4), 605–608. [Google Scholar]
25. Shang, H. Q., Gao, C. Y. (2018). Effects of cadmium and mercury stress on seed germination, seedling physiological and biochemical characteristics and cadmium and mercury levels in Eryngium spp. Journal of Nuclear Agricultural Sciences, 32(6), 1211–1219. https://doi.org/10.11869/j.issn.100-8551.2018.06.1211 [Google Scholar] [CrossRef]
26. Wei, C., Liu, X., Song, Z., Chen, G. (2017). Comparison of heavy metal contents in Chinese herbal medicine and their cultivated soils from different habitats. Journal of Northeast Agricultural Sciences, 42(4), 39–43. https://doi.org/10.16423/j.cnki.1003-8701.2017.04.011 [Google Scholar] [CrossRef]
27. Xin, J., Zhao, X., Tan, Q., Sun, X., Hu, C. (2017). Comparison of cadmium absorption, translocation, subcellular distribution and chemical forms between two radish cultivars (Raphanus sativus L.). Ecotoxicology and Environmental Safety, 145, 258–265. https://doi.org/10.1016/j.ecoenv.2017.07.042 [Google Scholar] [PubMed] [CrossRef]
28. Li, G., Li, Q., Wang, L., Chen, G., Zhang, D. (2019). Subcellular distribution, chemical forms, and physiological response to cadmium stress in Hydrilla verticillata. International Journal of Phytoremediation, 21(3), 230–239. https://doi.org/10.1080/15226514.2018.1524830 [Google Scholar] [PubMed] [CrossRef]
29. Zhao, Y., Wu, J., Shang, D., Ning, J., Zhai, Y. et al. (2015). Subcellular distribution and chemical forms of cadmium in the edible seaweed, Porphyra yezoensis. Food Chemistry, 168(2), 48–54. https://doi.org/10.1016/j.foodchem.2014.07.054 [Google Scholar] [PubMed] [CrossRef]
30. Xu, J., Yu, M. G., Chen, Y. X., Fu, X. P., Duan, D. C. (2011). Characteristics of distribution and chemical forms of Pb in tea plant varieties. Ying Yong Sheng Tai Xue Bao, 22(4), 891–896. [Google Scholar] [PubMed]
31. Zhao, Y., Shang, D., Ning, J., Zhai, Y., Sheng, X. et al. (2019). Subcellular distribution and chemical forms of lead in the red algae, Porphyra yezoensis. Chemosphere, 227, 172–178. https://doi.org/10.1016/j.chemosphere.2019.04.049 [Google Scholar] [PubMed] [CrossRef]
32. Su, Y., Liu, J., Lu, Z., Wang, X., Zhang, Z. et al. (2014). Effects of iron deficiency on subcellular distribution and chemical forms of cadmium in peanut roots in relation to its translocation. Environmental and Experimental Botany, 97, 40–48. https://doi.org/10.1016/j.envexpbot.2013.10.001 [Google Scholar] [CrossRef]
33. Yang, L. P., Zhu, J., Wang, P., Zeng, J., Tan, R. et al. (2018). Effect of Cd on growth, physiological response, Cd subcellular distribution and chemical forms of Koelreuteria paniculata. Ecotoxicology and Environmental Safety, 160(14078), 10–18. https://doi.org/10.1016/j.ecoenv.2018.05.026 [Google Scholar] [PubMed] [CrossRef]
34. Xu, X., Zhang, S., Xian, J., Yang, Z., Cheng, Z. et al. (2018). Subcellular distribution, chemical forms and thiol synthesis involved in cadmium tolerance and detoxification in Siegesbeckia orientalis L. International Journal of Phytoremediation, 20(10), 973–980. https://doi.org/10.1080/15226514.2017.1365351 [Google Scholar] [PubMed] [CrossRef]
35. Xin, J., Huang, B. (2014). Subcellular distribution and chemical forms of cadmium in two hot pepper cultivars differing in cadmium accumulation. Journal of Agricultural and Food Chemistry, 62(2), 508–515. https://doi.org/10.1021/jf4044524 [Google Scholar] [PubMed] [CrossRef]
36. Wang, J., Chen, X., Chu, S., Hayat, K., Chi, Y. et al. (2021). Influence of Cd toxicity on subcellular distribution, chemical forms, and physiological responses of cell wall components towards short-term Cd stress in Solanum nigrum. Environmental Science and Pollution Research, 28(11), 13955–13969. https://doi.org/10.1007/s11356-020-11505-5 [Google Scholar] [PubMed] [CrossRef]
37. Lai, H. Y. (2015). Subcellular distribution and chemical forms of cadmium in Impatiens walleriana in relation to its phytoextraction potential. Chemosphere, 138, 370–376. https://doi.org/10.1016/j.chemosphere.2015.06.047 [Google Scholar] [PubMed] [CrossRef]
38. Lux, A., Martinka, M., Vaculik, M., White, P. J. (2011). Root responses to cadmium in the rhizosphere: A review. Journal of Experimental Botany, 62(1), 21–37. https://doi.org/10.1093/jxb/erq281 [Google Scholar] [PubMed] [CrossRef]
39. Borowiak, K., Kanclerz, J., Mleczek, M., Lisiak, M., Drzewiecka, K. et al. (2016). Accumulation of Cd and Pb in water, sediment and two littoral plants (Phragmites australis, Typha angustifolia) of freshwater ecosystem. Archives of Environmental Protection, 42(3), 47–57. https://doi.org/10.1515/aep-2016-0032 [Google Scholar] [CrossRef]
40. Xin, J. P., Zhang, Y., Tian, R. N. (2018). Tolerance mechanism of Triarrhena sacchariflora (Maxim.) Nakai. seedlings to lead and cadmium: Translocation, subcellular distribution, chemical forms and variations in leaf ultrastructure. Ecotoxicology and Environmental Safety, 165, 611–621. https://doi.org/10.1016/j.ecoenv.2018.09.022 [Google Scholar] [PubMed] [CrossRef]
41. Dong, X., Yang, F., Yan, S., Yan, C. (2019). Subcellular distribution and tolerance of cadmium in Canna indica L. Ecotoxicology and Environmental Safety, 185(2), 109692. https://doi.org/10.1016/j.ecoenv.2019.109692 [Google Scholar] [PubMed] [CrossRef]
42. Zhang, S. J., Li, T. X., Huang, H. G., Zhang, X. Z., Yu, H. Y. et al. (2014). Phytoremediation of cadmium using plant species of Athyrium wardii (Hook.). International Journal of Environmental Science and Technology, 11(3), 757–764. https://doi.org/10.1007/s13762-013-0384-z [Google Scholar] [CrossRef]
43. He, C., Zhao, Y., Wang, F., Oh, K., Zhao, Z. et al. (2020). Phytoremediation of soil heavy metals (Cd and Zn) by castor seedlings: Tolerance, accumulation and subcellular distribution. Chemosphere, 252, 126471. https://doi.org/10.1016/j.chemosphere.2020.126471 [Google Scholar] [PubMed] [CrossRef]
44. Qiao, L., Tanveer, M., Wang, L., Tian, C. (2018). Subcellular distribution and chemical forms of lithium in Li-accumulator Apocynum venetum. Plant Physiology and Biochemistry, 132, 341–344. https://doi.org/10.1016/j.plaphy.2018.09.022 [Google Scholar] [PubMed] [CrossRef]
45. Fu, X., Dou, C., Chen, Y., Chen, X., Shi, J. et al. (2011). Subcellular distribution and chemical forms of cadmium in Phytolacca americana L. Journal of Hazardous Materials, 186(1), 103–107. https://doi.org/10.1016/j.jhazmat.2010.10.122 [Google Scholar] [PubMed] [CrossRef]
46. Weng, B., Xie, X., Weiss, D. J., Liu, J., Lu, H. et al. (2012). Kandelia obovata (S., L.) Yong tolerance mechanisms to cadmium: Subcellular distribution, chemical forms and thiol pools. Marine Pollution Bulletin, 64(11), 2453–2460. https://doi.org/10.1016/j.marpolbul.2012.07.047 [Google Scholar] [PubMed] [CrossRef]
47. Mwamba, T. M., Li, L., Gill, R. A., Islam, F., Nawaz, A. et al. (2016). Differential subcellular distribution and chemical forms of cadmium and copper in Brassica napus. Ecotoxicology and Environmental Safety, 134, 239–249. https://doi.org/10.1016/j.ecoenv.2016.08.021 [Google Scholar] [PubMed] [CrossRef]
48. Lu, H., Li, Z., Wu, J., Shen, Y., Li, Y. et al. (2017). Influences of calcium silicate on chemical forms and subcellular distribution of cadmium in Amaranthus hypochondriacus L. Scientific Reports, 7(1), 40583. https://doi.org/10.1038/srep40583 [Google Scholar] [PubMed] [CrossRef]
49. Xiao, Y. T., Du, Z. J., Busso, C. A., Qi, X. B., Wu, H. Q. et al. (2020). Differences in root surface adsorption, root uptake, subcellular distribution, and chemical forms of Cd between low- and high-Cd-accumulating wheat cultivars. Environmental Science and Pollution Research, 27(2), 1417–1427. https://doi.org/10.1007/s11356-019-06708-4 [Google Scholar] [PubMed] [CrossRef]
50. Hu, X., Li, T., Xu, W., Chai, Y. (2021). Distribution of cadmium in subcellular fraction and expression difference of its transport genes among three cultivars of pepper. Ecotoxicology and Environmental Safety, 216, 112182. DOI https://doi.org/10.1016/j.ecoenv.2021.112182 [Google Scholar] [PubMed] [CrossRef]
51. Vaahtera, L., Schulz, J., Hamann, T. (2019). Cell wall integrity maintenance during plant development and interaction with the environment. Nature Plants, 5(9), 924–932. https://doi.org/10.1038/s41477-019-0502-0 [Google Scholar] [PubMed] [CrossRef]
52. Li, G., Li, Q., Wang, L., Zhang, D. (2020). Cadmium tolerance and detoxification in Myriophyllum aquaticum: Physiological responses, chemical forms, and subcellular distribution. Environmental Science and Pollution Research, 27(30), 37733–37744. https://doi.org/10.1007/s11356-020-09872-0 [Google Scholar] [PubMed] [CrossRef]
53. Qiu, Q., Wang, Y., Yang, Z., Yuan, J. (2011). Effects of phosphorus supplied in soil on subcellular distribution and chemical forms of cadmium in two Chinese flowering cabbage (Brassica parachinensis L.) cultivars differing in cadmium accumulation. Food and Chemical Toxicology, 49(9), 2260–2267. https://doi.org/10.1016/j.fct.2011.06.024 [Google Scholar] [PubMed] [CrossRef]
54. Li, H., Luo, N., Zhang, L. J., Zhao, H. M., Li, Y. W. et al. (2016). Do arbuscular mycorrhizal fungi affect cadmium uptake kinetics, subcellular distribution and chemical forms in rice? Science of the Total Environment, 571, 1183–1190. https://doi.org/10.1016/j.scitotenv.2016.07.124 [Google Scholar] [PubMed] [CrossRef]
55. Wang, J., Yuan, J., Yang, Z., Huang, B., Zhou, Y. et al. (2009). Variation in cadmium accumulation among 30 cultivars and cadmium subcellular distribution in 2 selected cultivars of water spinach (Ipomoea aquatica Forsk.). Journal of Agricultural and Food Chemistry, 57(19), 8942–8949. https://doi.org/10.1021/jf900812s [Google Scholar] [PubMed] [CrossRef]
56. Dai, M., Liu, W., Hong, H., Lu, H., Liu, J. et al. (2018). Exogenous phosphorus enhances cadmium tolerance by affecting cell wall polysaccharides in two mangrove seedlings Avicennia marina (Forsk.) Vierh and Kandelia obovata (S., L.) Yong differing in cadmium accumulation. Marine Pollution Bulletin, 126, 86–92. DOI https://doi.org/10.1016/j.marpolbul.2017.10.083 [Google Scholar] [PubMed] [CrossRef]
57. Wang, S., Dong, Q., Wang, Z. (2017). Differential effects of citric acid on cadmium uptake and accumulation between tall fescue and Kentucky bluegrass. Ecotoxicology and Environmental Safety, 145, 200–206. DOI https://doi.org/10.1016/j.ecoenv.2017.07.034 [Google Scholar] [PubMed] [CrossRef]
58. Huang, R., Xin, J. P., Tian, R. N. (2022). Growth changes and characteristics of cadmium accumulation and distribution in Pistia stratiotes under cadmium stress. Journal of Agro-Environment Science, 41(9), 2033–2042. https://doi.org/10.11654/jaes.2022-0008 [Google Scholar] [CrossRef]
59. Li, X., Ma, H., Li, L., Gao, Y., Li, Y. et al. (2019). Subcellular distribution, chemical forms and physiological responses involved in cadmium tolerance and detoxification in Agrocybe Aegerita. Ecotoxicology and Environmental Safety, 171, 66–74. https://doi.org/10.1016/j.ecoenv.2018.12.063 [Google Scholar] [PubMed] [CrossRef]
60. Kuang, X., Wang, W., Hu, J., Liu, W., Zeng, W. (2022). Subcellular distribution and chemical forms of manganese in Daucus carota in relation to its tolerance. Frontiers in Plant Science, 13, 947882. https://doi.org/10.3389/fpls.2022.947882 [Google Scholar] [PubMed] [CrossRef]
61. Tang, M., Zhang, X., Tan, X. R., Liu, Y., Wang, M. X. (2021). Accumulation, subcellular distribution, and chemical forms of zinc in three tree species. Ying Yong Sheng Tai Xue Bao, 32(12), 4298–4306. https://doi.org/10.13287/j.1001-9332.202112.033 [Google Scholar] [PubMed] [CrossRef]
62. Wu, F. B., Dong, J., Qian, Q. Q., Zhang, G. P. (2005). Subcellular distribution and chemical form of Cd and Cd-Zn interaction in different barley genotypes. Chemosphere, 60(10), 1437–1446. https://doi.org/10.1016/j.chemosphere.2005.01.071 [Google Scholar] [PubMed] [CrossRef]
63. Vega, A., Delgado, N., Handford, M. (2022). Increasing heavy metal tolerance by the exogenous application of organic acids. International Journal of Molecular Sciences, 23(10), 5438. https://doi.org/10.3390/ijms23105438 [Google Scholar] [PubMed] [CrossRef]
64. Kocaman, A. (2022). Combined interactions of amino acids and organic acids in heavy metal binding in plants. Plant Signaling & Behavior, 2064072. https://doi.org/10.1080/15592324.2022.2064072 [Google Scholar] [PubMed] [CrossRef]
65. Thakur, M., Praveen, S., Divte, P. R., Mitra, R., Kumar, M. et al. (2022). Metal tolerance in plants: Molecular and physicochemical interface determines the "not so heavy effect" of heavy metals. Chemosphere, 287, 131957. https://doi.org/10.1016/j.chemosphere.2021.131957 [Google Scholar] [PubMed] [CrossRef]
66. Nkoh, J. N., Yan, J., Hong, Z., Xu, R. (2020). Effects of citrate, oxalate, and phosphate on the sorption of Cr(VI) by extracellular polymeric substances. Journal of Water Process Engineering, 37, 101510. https://doi.org/10.1016/j.jwpe.2020.101510 [Google Scholar] [CrossRef]
67. Zhang, X., Liu, J., Wang, D., Zhu, Y., Hu, C. (2009). Bioaccumulation and chemical form of chromium in Leersia hexandra Swartz. Bulletin of Environmental Contamination and Toxicology, 82(3), 358–362. https://doi.org/10.1007/s00128-008-9587-2 [Google Scholar] [PubMed] [CrossRef]
68. Zhu, X. F., Zheng, C., Hu, Y. T., Jiang, T., Liu, Y. et al. (2011). Cadmium-induced oxalate secretion from root apex is associated with cadmium exclusion and resistance in Lycopersicon esulentum. Plant, Cell & Environment, 34(7), 1055–1064. https://doi.org/10.1111/j.1365-3040.2011.02304.x [Google Scholar] [PubMed] [CrossRef]
69. Lu, R. R., Hu, Z. H., Zhang, Q. L., Li, Y. Q., Lin, M. et al. (2020). The effect of Funneliformis mosseae on the plant growth, Cd translocation and accumulation in the new Cd-hyperaccumulator Sphagneticola calendulacea. Ecotoxicology and Environmental Safety, 203, 110988. https://doi.org/10.1016/j.ecoenv.2020.110988 [Google Scholar] [PubMed] [CrossRef]
70. Tian, S., Lu, L., Labavitch, J., Yang, X., He, Z. et al. (2011). Cellular sequestration of cadmium in the hyperaccumulator plant species Sedum alfredii. Plant Physiology, 157(4), 1914–1925. https://doi.org/10.1104/pp.111.183947 [Google Scholar] [PubMed] [CrossRef]
Appendix
Supplementary Materials (Supplementary Chapters, Table S1, and Table S2).
Cite This Article
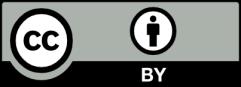
This work is licensed under a Creative Commons Attribution 4.0 International License , which permits unrestricted use, distribution, and reproduction in any medium, provided the original work is properly cited.