Open Access
ARTICLE
Salicylic Acid Application Mitigates Oxidative Damage and Improves the Growth Performance of Barley under Drought Stress
1
Institute of Biotechnology and Genetic Engineering, Bangabandhu Sheikh Mujibur Rahman Agricultural University, Gazipur, 1706,
Bangladesh
2
Institute of Genomics for Crop Abiotic Stress Tolerance, Department of Plant and Soil Science, Texas Tech University, Lubbock,
79409, USA
3
Plant Breeding Division, Bangladesh Agricultural Research Institute, Gazipur, 1701, Bangladesh
4
Department of Energy Plant Research Laboratory, Michigan State University, East Lansing, 48824, Michigan, USA
* Corresponding Author: Mohammad Golam Mostofa. Email:
Phyton-International Journal of Experimental Botany 2023, 92(5), 1513-1537. https://doi.org/10.32604/phyton.2023.025175
Received 26 June 2022; Accepted 01 December 2022; Issue published 09 March 2023
Abstract
Drought is a severe environmental constraint, causing a significant reduction in crop productivity across the world. Salicylic acid (SA) is an important plant growth regulator that helps plants cope with the adverse effects induced by various abiotic stresses. The current study investigated the potential effects of SA on drought tolerance efficacy in two barley (Hordeum vulgare) genotypes, namely BARI barley 5 and BARI barley 7. Ten-day-old barley seedlings were exposed to drought stress by maintaining 7.5% soil moisture content in the absence or presence of 0.5, 1.0 and 1.5 mM SA. Drought exposure led to severe damage to both genotypes, as indicated by phenotypic aberrations and reduction of dry biomass. On the other hand, the application of SA to drought-stressed plants protected both barley genotypes from the adverse effects of drought, which was reflected in the improvement of phenotypes and biomass production. SA supplementation improved relative water content and proline levels in drought-stressed barley genotypes, indicating the osmotic adjustment functions of SA under water-deficit conditions. Drought stress induced the accumulation of reactive oxygen species (ROS), such as hydrogen peroxide (H2O2) and superoxide (O2 •− ), and the lipid peroxidation product malondialdehyde (MDA) in the leaves of barley plants. Exogenous supply of SA reduced oxidative damage by restricting the accumulation of ROS through the stimulation of the activities of key antioxidant enzymes, including superoxide dismutase (SOD), peroxidase (POD), catalase (CAT), ascorbate peroxidase (APX) and glutathione peroxidase (GPX). Among the three-applied concentrations of SA, 0.5 mM SA exhibited better mitigating effects against drought stress considering the phenotypic performance and biochemical data. Furthermore, BARI barley 5 showed better performance under drought stress than BARI barley 7 in the presence of SA application. Collectively, our results suggest that SA played a crucial role in improving water status and antioxidant defense strategy to protect barley plants from the deleterious effects of water deficiency.Keywords
Drought is a major environmental threat that limits crop production, and its occurrence is anticipated to be increased further due to unprecedented climate change [1,2]. Crop production is predicted to drop by up to 30% by 2025 compared to 2018 due to water shortage [3]. Drought stress negatively affects various morphological, physiological and metabolic attributes in plants. Increased production of reactive oxygen species (ROS), such as singlet oxygen (1O2), superoxide (O2•−), hydrogen peroxide (H2O2) and hydroxyl radicals (OH.) is a key sign of drought stress at the cellular level [4,5]. ROS are extremely toxic and can oxidize biomolecules like DNA, proteins, lipids and pigments, leading to plant cell death and growth retardation [4]. Plants have evolved sophisticated antioxidant systems to protect cells from ROS-induced damage, which plays an important role in sustaining the normal metabolism of plants under stressful conditions [6,7]. The antioxidant defense of plants includes both enzymes, including superoxide dismutase (SOD), catalase (CAT), glutathione peroxidase (GPX), glutathione S-transferase (GST), ascorbate peroxidase (APX), monodehydroascorbate (MDHAR), dehydroascorbate reductase (DHAR) and glutathione reductase (GR), and non-enzymatic components, such as ascorbic acid (AsA) and glutathione (GSH) [8]. An integrated function of both enzymatic and non-enzymatic systems is critical to mounting a strong defense against ROS toxicity and assisting plants in surviving under drought adversities.
Barley (Hordeum vulgare) is one of the most significant cereal crops in the world. Despite its potential aspect in contribution to food and nutritional security, Bangladesh’s present barley production situation is highly concerning. Drought is believed to be one of the biggest hindrances to barley production in Bangladesh as a result of rising climate change [9]. Barley has four water-sensitive growth stages, including germination, booting, anthesis and milky [10]. Thus, it is crucial to understand the putative mechanisms underlying barley’s water resilience if drought-tolerant varieties are to be developed for sustaining barley production in arid and semi-arid regions. Moreover, effective measures should be undertaken to curtail the loss of barley productivity in drought-prone areas.
Salicylic acid (SA) is a naturally occurring phytohormone that affects a variety of physiological and biochemical activities in plants. SA can function as a signaling molecule and has a variety of roles in conferring abiotic stress tolerance in plants [11,12]. A wealth of studies revealed that SA treatment improves a plant’s ability to withstand a variety of abiotic stresses, including ozone, heat, salinity, heavy metal toxicity and osmotic stress [13–17]. When exogenously sprayed on the drought-stressed tomato (Solanum lycopersicum) plants, SA was found to stimulate the function of the antioxidant defense system by enhancing the activities of several enzymes, such as SOD, peroxidase (POD) and CAT [18]. Application of exogenous SA also enhanced the growth and photosynthetic rate in wheat (Triticum aestivum) plants under water-limited conditions [19]. Nevertheless, numerous studies demonstrated that the effectiveness of exogenous SA varied depending on various factors, including the type of species, plant developmental stages, the mode of application and the dose of SA [11,20–22]. The effect of SA on the growth performance of barley has not yet been thoroughly investigated. Moreover, the roles of SA in modulating oxidative stress tolerance in barley under water-shortage conditions were rarely examined.
Thus, the present study aimed to investigate the drought tolerance and responses of two commonly used barley genotypes, BARI Barley-5 (BB-5) and BARI Barley-7 (BB-7). We also evaluated the beneficial effects of SA in mediating the drought tolerance potential of both barley genotypes at physiological and biochemical levels. Finally, we looked at the effects of different doses of SA and their efficacy in two barley genotypes under restricted water availability in greenhouse settings.
2.1 Plant Materials and Treatments of Barley Genotypes with Drought and SA
The seeds of two high-yielding barley genotypes, namely BARI Barley-5 (BB-5) and BARI Barley-7 (BB-7), were generously provided by Plant Breeding Division, Bangladesh Agriculture Research Institute (BARI), Gazipur, Bangladesh. Barley seeds were surface sterilized by immersing them in 70% ethanol for 30 s, followed by treatment with 3% sodium hypochlorite solution for three minutes (min). The sterilized seeds were thoroughly washed five times with distilled water. The seeds were then sown in trays filled with a mixture of coarse sand and gravel for germination in the greenhouse at 22°C. After seven days of seed sowing, uniformly germinated 25 seedlings were selected and transferred to the plastic pots (pot size 2L; 1.80 Kg soil/pot) filled with soil and commercial compost (ACI Bumper Biofertilizer, ACI Limited, Dhaka, Bangladesh) at 4:1 ratio. Ten-day-old newly established seedlings of each genotype (17-day-old plants) were then subjected to drought stress by stopping irrigation. Soil moisture content (SMC) of the pots was regularly recorded using a soil moisture meter (MO750, Extech Instruments, Taiwan). Typical drought symptoms in barley seedlings were observed when SMC reached 7.5%. SMC at a 7.5% level was maintained for a period of seven days with the addition of the required amount of water. During this 7-day drought period, one set of drought-stressed seedlings of each genotype was foliar-sprayed with 500 mL of SA at three different concentrations, including 0.5 mM (SA1), 1.0 mM (SA2) and 1.5 mM (SA3). An equal volume of distilled water was sprayed in only drought-stressed and control plants.
The control seedlings were cultivated under 80% SMC alongside drought-stressed seedlings. Thus, each genotype comprised of 5 treatments as follows: (i) 80% SMC (control), (ii) 7.5% SMC (Drought), (iii) Drought + SA1, (iv) Drought + SA2 and (v) Drought + SA3. On day 7th of continuous maintenance of 7.5% SMC (hereafter referred to as drought treatment), shoot samples of drought-stressed and -unstressed plants (24-day-old plants) were collected for various physiological and biochemical analyses. The experiment had four independent replications (n =4) under the same experimental conditions.
2.2 Analysis of Phenotypes, Biomass and Relative Water Content
To record the drought effects on barely phenotypes, a digital camera (Nikon D700, Tokyo, Japan) was used to photograph the plants on day 7th of drought treatments. For the determination of plant biomass, five plants were randomly selected from each pot and carefully uprooted from the soil. The roots were cleaned and washed using tap water to remove soil particles, followed by soaking the roots in paper towels. Fresh weight (FW) of each plant was taken, and the plant samples were kept in labeled paper bags. The paper bags were then placed in an oven at 70°C for 24 h. The oven-dried samples were cooled in a desiccator jar, and dry weight (DW) was taken using an electronic balance.
Relative water content (RWC) of the detached aerial parts of the plants was determined following the method of Das et al. (2022) [23]. RWC was calculated using the formula; RWC (%) = (FW – DW)/ (TW – DW) × 100. FW, fresh weight; TW, turgid weight; DW, dry weight.
2.3 Determination of Proline Content
Proline content was colorimetrically determined following the procedure of Bates et al. [24]. Briefly, fresh leaf samples (0.5 g) were homogenized in 10 mL of 3% sulfosalicylic acid in ice. The homogenate was centrifuged at 11,500 × g for 15 min. Two mL of the filtrates were mixed with 2 mL of acid ninhydrin and 2 mL of glacial acetic acid solutions. The mixture was then boiled at 100°C for 1 h by placing the tubes in a hot water bath. The tubes were immediately cooled in an ice bath, followed by the addition of 4 mL of toluene to each tube. After 30 min of reaction, the developed chromophore was separated, and the absorbance was taken against toluene at 520 nm. The proline content was calculated using a standard graph developed with a series of proline concentrations.
2.4 Estimation of the Superoxide, Hydrogen Peroxide and Malondialdehyde Contents
The level of superoxide (O2•−) was determined according to the method of Elstner and Heupel [25] with some modifications. Barley leaf samples (0.3 g) were homogenized in 3 mL of 65 mM potassium-phosphate (K-P) buffer (pH 7.8) using mortars and pestles in an ice bath. After centrifugation at 5,000 × g for 10 min at 4°C, the supernatants (0.75 mL) were mixed with 0.67 mL of 65 mM K-P buffer and 0.07 mL of 10 mM hydroxylamine hydrochloride. The reaction was incubated at 25°C for 20 min. Following the incubation, 0.37 mL of 17 mM sulfanilamide and 0.37 mL of 7 mM α-naphthylamine were added, and the mixture was further incubated at 25°C for 20 min before it was mixed with 2.25 mL of diethyl ether. The absorbance was measured at 530 nm, and the O2•− concentration was calculated from a standard curve developed with sodium nitrite.
The H2O2 level was assayed according to the method described by Yu et al. [26]. H2O2 was extracted by homogenizing 0.5 g of leaf samples in 3 mL of 50 mM K-P buffer (pH 6.5) at 4°C. The homogenate was centrifuged at 11,500 × g for 15 min. Three mL of the supernatants were mixed with 1 mL of 0.1% TiCl4 in 20% H2SO4 (v/v) and kept at room temperature for 10 min. After that, the mixture was again centrifuged at 11,500 × g for 15 min. The absorbance of the collected supernatant was read at 410 nm for calculating H2O2 content using an extinction coefficient of 0.28 μM−1cm−1.
The level of lipid peroxidation was measured by estimating malondialdehyde (MDA) using thiobarbituric acid (TBA) as the reactive material following the method of Heath and Packer [27]. The leaf samples (0.5 g) were homogenized in 3 mL of 5% (w/v) trichloroacetic acid (TCA) solution, and the homogenate was centrifuged at 11,500 × g for 10 min. One mL supernatant was mixed with 4 mL of TBA reagent (0.5% of TBA in 20% TCA). The reaction mixture was heated in a water bath at 95°C for 30 min, followed by rapid cooling in an ice bath before being centrifuged at 11,500 × g for 15 min. The absorbance of the colored supernatant was measured at 532 nm and corrected for nonspecific absorbance at 600 nm. The content of MDA was calculated using the extinction coefficient of 155 mM−1 cm−1.
2.5 Enzyme Extraction and Determination of Enzymatic Activities
The leaf samples (0.5 g) were homogenized in 1 mL of 50 mM ice-cold K-P buffer (pH 7.0) containing 100 mM KCl, 1 mM ascorbate, 5 mM β-mercaptoethanol and 10% (w/v) glycerol using pre-cooled mortars and pestles. The homogenates were centrifuged at 11,500 × g for 10 min, and the supernatants were used to determine protein content and antioxidant enzyme activities. All procedures were carried out at 0°C–4°C. SOD activity was estimated according to Beyer and Fridovich [28], which was based on the xanthine-xanthine oxidase system. The reaction mixture contained K-P buffer (50 mM), nitroblue tetrazolium (NBT, 2.24 mM), CAT (0.1 units), xanthine oxidase (0.1 units), xanthine (2.36 mM) and enzyme extract. CAT was added to avoid the H2O2-mediated possible inactivation of CuZn-SOD. SOD activity was expressed as units (amount of enzyme required to inhibit NBT reduction by 50%) min−1 mg−1 protein.
The activity of POD was assayed, according to Hemeda and Klein [29]. The reaction mixture contained 25 mM K-P buffer (pH 7.0), 0.05% guaiacol, 10 mM H2O2 and the enzyme extract. POD activity was determined by the increase in absorbance at 470 nm due to guaiacol oxidation for 1 min using an extinction coefficient of 26.6 mM−1 cm−1. CAT activity was measured according to the method of Csiszár et al. [30] by monitoring the decrease of absorbance at 240 nm for 1 min due to the decomposition of H2O2. The reaction mixture contained 50 mM K-P buffer (pH 7.0), 15 mM H2O2, and enzyme solution in a total volume of 0.7 mL. The reaction was initiated with the addition of enzyme extract, and the activity was calculated using the extinction coefficient of 39.4 M−1 cm−1.
The activity of APX was assayed following the method of Nakano and Asada [31]. The reaction solution contained 50 mM K-P buffer (pH 7.0), 0.50 mM ascorbate, 0.10 mM H2O2, 0.1 mM EDTA and enzyme extract in a final volume of 0.7 mL. The reaction was started by the addition of H2O2, and the activity was measured by observing the decrease in absorbance at 290 nm for 1 min using an extinction coefficient of 2.8 mM−1 cm−1. GPX activity was measured as described by Elia et al. [32] using H2O2 as a substrate. The reaction mixture consisted of 100 mM Na-P buffer (pH 7.5), 1 mM EDTA, 1 mM NaN3, 0.12 mM NADPH, 2 mM GSH, 1 unit GR, 0.6 mM H2O2, and 5 μL of sample extract. The reaction was started by the addition of H2O2. The oxidation of NADPH was recorded at 340 nm for 1 min, and the activity was calculated using the extinction coefficient of 6.62 mM−1 cm−1. Protein content was estimated according to the procedure described by Bradford [33].
All data were analyzed using an one-way and two-way analysis of variance (ANOVA) (Data used in the ANOVA was presented in Supplementary File 1), and the mean values of different treatments were compared using a least significant difference (LSD) test. The significance level was chosen at p < 0.05. Statistix 10 package was employed for all statistical analyses. The ‘pheatmap’ and ‘devtools’ packages were used to perform hierarchical clustering and principal component analysis (PCA), respectively [34].
3.1 Exogenous SA Application Improved Growth Performance of Barley Genotypes under Drought Stress
Under drought conditions, both barley genotypes exhibited severe phenotypical aberrations, including rolling of younger leaves, dull appearance and wilting of older leaves (Fig. 1A). The drought-induced damage was more visible in the BB-7 genotype when compared with that of the BB-5 genotype. Application of different doses of exogenous SA to the drought-stressed plants reversed the adverse effects of drought and improved the growth performance of both genotypes concerning ‘Drought’ plants only. However, SA1 and SA2 showed better recovery of the barley genotypes from drought-induced adverse effects when compared with SA3 (Fig. 1A). The effects of SA on barley growth under drought stress conditions were measured by estimating the DW of the whole plant. Drought stress caused a drastic reduction in DW by 55.47% and 44.53% in BB-5 and BB-7, respectively, compared with their respective ‘Control’ plants (Fig. 1B). However, foliar application of SA inhibited growth retardation and showed improvement of plant biomass under drought conditions in both genotypes (Fig. 1B). In BB-5, SA application increased plant biomass by 53.88%, 37.20% and 35.38% in ‘Drought+SA1’, ‘Drought+SA2’ and ‘Drought+SA3’ plants, respectively, compared with ‘Drought’ plants. On the other hand, the external application of SA improved BB-7 DW by 67.12%, 40.11% and 26.82% in ‘Drought+SA1’, ‘Drought+SA2’ and ‘Drought+SA3’ plants, respectively, as compared with ‘Drought’ plants (Fig. 1B). Collectively, BB-5 displayed higher plant biomass and performed better under drought stress conditions than BB-7. Interestingly, a low dose of SA (i.e., SA1) was more effective in protecting biomass of barley genotypes from drought effects when compared with the doses of SA2 and SA3 (Fig. 1).
Figure 1: Effects of foliar application of SA on the (A) the phenotypic appearance and (B) dry biobaass of BB-5 and BB-7 barley cultivars after exposure to drought stress for 7 days. Control, Drought, Drought+SA1, Drought+SA2, and Drought+SA3 represent 80% SMC, 7.5% SMC, 7.5% SMC + 0.5 mM SA, 7.5% SMC + 1.0 mM SA and 7.5% SMC + 1.5 mM SA, respectively. Values represent the means ± SEs (n = 4). Different capital and small alphabetical letters on the bars indicate significant differences among the treatments within the cultivar and between the cultivars, respectively, at p < 0.05 following the least significant difference test
3.2 Exogenous SA Improved the Levels of Proline and Relative Water Content
We quantified the level of Pro and RWC to assess water status and osmoprotectant strategy in both barley genotypes under drought conditions. Compared with ‘Control’ plants, leaf Pro content was increased by 256.82% and 102.06% in BB-5 and BB-7, respectively, under drought conditions (Fig. 2A). On the other hand, ‘Drought+SA1’ plants displayed a noteworthy increase in Pro level by 15.03% and 15.09% in BB-5 and BB-7 genotypes, respectively, when compared with the corresponding ‘Drought’ plants. However, comparable data were found for Pro among ‘Drought’, ‘Drought+SA2’ and ‘Drought+SA3’ plants of both genotypes (Fig. 2A).
Figure 2: Effects of foliar application of SA on the levels of (A) proline and (B) RWC of BB-5 and BB-7 barley cultivars after exposure to drought stress for 7 days. Control, Drought, Drought+SA1, Drought+SA2, and Drought+SA3 represent 80% SMC, 7.5% SMC, 7.5% SMC + 0.5 mM SA, 7.5% SMC + 1.0 mM SA and 7.5% SMC + 1.5 mM SA, respectively. Values represent the means ± SEs (n = 4). Different capital and small alphabetical letters on the bars indicate significant differences among the treatments within the cultivar and between the cultivars, respectively, at p < 0.05 following the least significant difference test. FW, fresh weight
We also found that ‘Drought’ plants of BB-5 and BB-7 genotypes had considerably lower levels of RWC (by 48.64% and 40.59%, respectively) than corresponding ‘Control’ plants (Fig. 2B). Surprisingly, in comparison with the ‘Drought’ plants, foliar application of SA significantly enhanced the leaf RWC in both genotypes under drought conditions (Fig. 2B). More precisely, we observed that the levels of leaf RWC in the BB-5 genotype were remarkably improved in ‘Drought+SA1’, ‘Drought+SA2’ and ‘Drought+SA3’ plants by 87.06%, 56.47% and 42.94%, respectively, relative to corresponding ‘Drought’ plants. Similarly, in comparison with the respective ‘Drought’ plants, ‘Drought+SA1’, ‘Drought+SA2’ and ‘Drought+SA3’ plants of the BB-7 genotype exhibited a noteworthy elevation of the leaf RWC by 52.97%, 33.66% and 18.32%, respectively (Fig. 2B). Moreover, our results indicated that SA1 was more effective in drought-induced water loss replenishment than SA2 and SA3.
3.3 Exogenous SA Reduced Oxidative Damage in Barley Plants under Drought Stress
To explore the role of SA in alleviating drought stress-mediated oxidative stress, we quantified the levels of ROS products, including O2•− and H2O2, as well as MDA in the leaves of both barley genotypes. In comparison with the ‘Control’ plants, we observed that drought stress exposure resulted in a significant increase in O2•− levels by 400.00% and 564.89% in BB-5 and BB-7, respectively (Fig. 3A). Intriguingly, foliar application of SA reduced O2•− content in both genotypes under drought situations (Fig. 3A). In BB-5, SA application caused a reduction of O2•− content by 44.95%, 40.71% and 27.75% in ‘Drought+SA1’, ‘Drought+SA2’ and ‘Drought+SA3’ plants, respectively, when contrasted with ‘Drought’ plants. Similarly, SA application reduced O2•− content in BB-7 genotype by 33.60%, 27.44% and 17.40% in ‘Drought+SA1’, ‘Drought+SA2’ and ‘Drought+SA3’ plants, respectively, compared with ‘Drought’ plants (Fig. 3A).
Figure 3: Effects of foliar application of SA on the levels of (A) O2•− (B) H2O2 and (C) MDA in the leaves of BB-5 and BB-7 barley cultivars after exposure to drought stress for 7 days. Control, Drought, Drought+SA1, Drought+SA2, and Drought+SA3 represent 80% SMC, 7.5% SMC, 7.5% SMC + 0.5 mM SA, 7.5% SMC + 1.0 mM SA and 7.5% SMC + 1.5 mM SA, respectively. Values represent the means ± SEs (n = 4). Different capital and small alphabetical letters on the bars indicate significant differences among the treatments within the cultivar and between the cultivars, respectively, at p < 0.05 following the least significant difference test. FW, fresh weight
When compared with those in ‘Control’ plants, the levels of H2O2 in drought-exposed BB-5 and BB-7 genotypes were increased significantly by 115.72% and 152.94%, respectively (Fig. 2B). Conversely, the exogenous supply of SA reduced the contents of H2O2 in both genotypes under drought conditions (Fig. 3B). More precisely, SA application to the drought-stressed BB-5 genotype remarkably reduced the levels of H2O2 by 34.83%, 32.47% and 23.34% in ‘Drought+SA1’, ‘Drought+SA2’ and ‘Drought+SA3’ plants, respectively, compared with ‘Drought’ plants. Likewise, ‘Drought+SA1’, ‘Drought+SA2’ and ‘Drought+SA3’ plants of BB-7 genotype displayed a substantial reduction in H2O2 level by 35.48%, 24.86%, and 14.91%, respectively, in comparison with ‘Drought’ only plants (Fig. 3B).
Compared with the corresponding ‘Control’ plants, ‘Drought’ plants of BB-5 and BB-7 genotypes exhibited an upsurge of MDA content by 158.00% and 143.28%, respectively (Fig. 3C). On the other hand, SA supplementation to the drought-stressed barley genotypes impressively attenuated MDA levels in leaves (Fig. 3C). In relation to the ‘Drought’ plants, ‘Drought+SA1’, ‘Drought+SA2’ and ‘Drought+SA3’ plants of BB-5 genotype showed a reduction of leaf MDA levels by 43.67%, 30.23% and 21.96%, respectively (Fig. 3C). Similarly, the exogenous application of SA to drought-exposed BB-7 genotype reduced MDA content by 33.13%, 23.01%, and 13.50% in ‘Drought+SA1’, ‘Drought+SA2’ and ‘Drought+SA3’ plants, respectively, in comparison with the corresponding ‘Drought’ plants (Fig. 3C).
Collectively, in the presence of SA, drought-stressed BB-5 plants accumulated less O2•−, H2O2 and MDA in their leaves than drought-stressed BB-7 plants. Furthermore, SA1 showed higher effects in safeguarding both barley genotypes from drought-mediated ROS-induced oxidative damage when compared with SA2 and SA3.
3.4 Exogenous SA Enhanced Antioxidant Defense System
Next, we investigated the activities of some important antioxidant enzymes to evaluate the involvement of SA in alleviating oxidative stress under drought conditions. We observed that drought stress to BB-5 and BB-7 plants significantly heightened the SOD activity by 200.09% and 181.29%, respectively, in comparison with the corresponding ‘Control’ plants (Fig. 4A). Strikingly, the activity of SOD was further escalated by external application of SA in ‘Drought+SA1’ and ‘Drought+SA2’ plants of BB-5 (by 21.55% and 13.70%) and BB-7 (29.03% and 11.72%, respectively) genotypes, in relation to those observed in the corresponding ‘Drought’ plants (Fig. 4A). However, the activity of SOD did not differ significantly between the ‘Drought’ and ‘Drought+SA3’ plants of both genotypes (Fig. 4A). On the other hand, CAT activity was substantially increased by 21.37% and 73.66% in the leaves of drought-exposed BB-5 and BB-7 genotypes, respectively, relative to that of ‘Control’ plants (Fig. 4B). By comparison, ‘Drought+SA1’, ‘Drought+SA2’ and ‘Drought+SA3’ plant leaves displayed a further enhancement of CAT activity in BB-5 (by 29.39%, 14.80% and 7.60%) and BB-7 (56.45%, 31.14% and 6.31%, respectively) genotypes when contrasted with the corresponding ‘Drought’ plants (Fig. 4B).
Figure 4: Effects of foliar application of SA on the activities of (A) SOD, (B) CAT, (C) APX, (D) POD, and (E) GPX in the leaves of BB-5 and BB-7 barley cultivars after exposure to drought stress for 7 days. Control, Drought, Drought+SA1, Drought+SA2, and Drought+SA3 represent 80% SMC, 7.5% SMC, 7.5% SMC + 0.5 mM SA, 7.5% SMC + 1.0 mM SA and 7.5% SMC + 1.5 mM SA, respectively. Values represent the means ± SEs (n =4). Different capital and small alphabetical letters on the bars indicate significant differences among the treatments within the cultivar and between the cultivars, respectively, at p < 0.05 following the least significant difference test
In comparison with the respective ‘Control’ plants, BB-5 and BB-7 genotypes exposed to drought stress showed a significant increment of APX activity by 93.20% and 133.09%, respectively (Fig. 4C). In contrast, a further increment in APX activity in ‘Drought+SA1’ (53.34% and 43.23%), ‘Drought+SA2’ (32.21% and 25.17%) and ‘Drought+SA3’ (28.83% and 19.50%) of BB-5 and BB-7 genotypes, respectively, were found compared with the values observed in their corresponding ‘Drought’ plants (Fig. 4C). Drought stress without SA supplementation caused a considerable increase in POD activity (by 94.86% and 276.47%) in the BB-5 and BB-7 genotypes, respectively, when compared with the corresponding ‘Control’ plants (Fig. 4D). By comparison, SA application further heightened the POD activity in ‘Drought+SA1’, ‘Drought+SA2’ and ‘Drought+SA3’ plants of BB-5 (by 17.13%, 3.85% and 2.22%) and BB-7 (by 24.53%, 11.42% and 6.95%, respectively) genotypes, in comparison with the values obtained in the corresponding ‘Drought’ plants (Fig. 4D).
Exposure of BB-5 and BB-7 genotypes to drought stress without SA supplementation displayed a significant rise in GPX activity by 99.82% and 118.06%, respectively, concerning the corresponding ‘Control’ plants (Fig. 4E). However, GPX activity increased even more in ‘Drought+SA1’ plant leaves of BB-5 (by 21.13%) and BB-7 (18.73%) genotypes than in the respective ‘Drought’ plants (Fig. 4E). We did not observe any significant divergence in the activity of GPX between ‘Drought’, and ‘Drought+SA2’ and ‘Drought+SA3’ plant leaves of both genotypes (Fig. 4E). Overall, we can conclude that adding SA to drought-stressed plants increased the activities of SOD, CAT, APX, POD and GPX further than the ‘Drought’ plants, and the effects of SA1 were more prominent than those of SA2 and SA3.
3.5 Evaluation of the Treatment-Variable Interaction Using Clustering Heatmap and PCA
The clustering heatmaps with different color gradients were generated to depict the overall scenario of the studied morpho-physiological and biochemical parameters under both normal and drought stress (with or without SA supplementation) conditions (Figs. 5A and 5B). The morpho-physiological and biochemical attributes of both genotypes were grouped into three distinct clusters (Cluster–I, –II and –III) (Figs. 5A and 5B). Interestingly, for both the genotypes, cluster-I represented morpho-physiological features like biomass and RWC, whereas cluster-II represented ROS products, such as O2•− and H2O2, and MDA, and cluster-III represented all ROS quenchers such as SOD, CAT, APX, GPX, POD and Pro (Figs. 5A and 5B). Compared with the respective ‘Control’ plants, all the parameters of cluster-I showed a decreasing trend under drought stress without SA supplementation; however, an increasing trend was observed for the parameters of cluster-II and cluster-III (Figs. 5A and 5B). By contrast, ‘Drought+SA1’, ‘Drought+SA2’ and ‘Drought+SA3’ plants displayed an increasing trend for cluster-I and cluster-III parameters (except for Pro and SOD in ‘Drought+SA3’ plants of BB-5 and BB-7 genotype, respectively), while the decreasing trend was observed for cluster-II parameters, in relation to ‘Drought’ plants (Figs. 5A and 5B). PCA was then carried out to understand the association of treatment groups with different morpho-physiological and biochemical parameters (Figs. 5C and 5D). For the BB-5 and BB-7 barley genotypes, the first two principal components, PC1 and PC2, collectively explained 99.46% and 99.54% of the data variabilities, respectively (Figs. 5C and 5D). The PCA biplot revealed that the variables in clusters-I and cluster-III were intricately associated with the ‘Drought+SA1’, ‘Drought+SA2’ and ‘Drought+SA3’ treatments of both genotypes; however, cluster-II exhibited a negative association with those treatment variables (Figs. 5C and 5D). Opposite trends were observed for SA-devoid drought-stressed plants (Figs. 5C and 5D).
Figure 5: (A and B) Clustered heatmap for treatment and variable relationship. Color scale indicates the changing trend of different parameters with different treatments. Normalized mean values of different parameters were used to construct the heatmap. The parameters were grouped into three distinct clusters for both cultivars. (C and D) PCA was conducted to understand the relationships between treatments and variable levels by the first two principal components (PC1 and PC2). The variables included, biomass, RWC, O2•−, H2O2, MDA, SOD (superoxide dismutase), CAT, APX, POD, GPX and Pro (proline). Control, Drought, Drought+SA1, Drought+SA2, and Drought+SA3 represent 80% SMC, 7.5% SMC, 7.5% SMC t + 0.5 mM SA, 7.5% SMC + 1.0 mM SA and 7.5% SMC + 1.5 mM SA, respectively
Drought stress is one of the most critical environmental constraints for agricultural crop production worldwide, resulting in significant annual yield losses of vital cereal crops, such as rice, maize, wheat, and other grains [35–37]. Therefore, scientists pay considerable attention to improving the drought resistance of cereal crops, especially barley, to meet the growing population’s food demands. Among numerous drought mitigation strategies, treating plants with phytohormones is now considered a viable technique for relieving the negative consequences of drought on plants [38]. The phytohormone SA has been considered an ‘effective therapeutic agent’ for plants due to its role in regulating physiological and biochemical processes under multiple abiotic stresses, including cold, drought, heat, heavy metal toxicity and osmotic stress [39–41]. In the current study, we examined the beneficial effects of SA in alleviating the negative consequences of drought effects on barley plants.
Our findings revealed that the barley genotypes exposed to drought stress exhibited severe aberration of phenotypes, including rolling of younger leaves, yellowing and wilting of older leaves, as well as a reduction of plant biomass (Fig. 1A). In line with our findings, drought stress-induced phenotypic distortions and decreased biomass have also been observed in other cereal crops, including rice, maize and wheat [35–37]. In contrast, foliar application of SA, particularly SA1, alleviated the negative impacts of drought stress, as evident by reduced leaf rolling, yellowing and wilting, as well as improved biomass production (Figs. 1A and 1B). PCA results further supported our findings, revealing that drought-exposed barley genotypes sprayed with SA had a lower extent of the negative association with biomass production when compared with SA-devoid drought-stressed plants (Figs. 5C and 5D). The positive regulatory role of SA in improving the phenotypic appearance and biomass production has also been reported in other cereals as well [42–43].
Plants accumulate a wide array of low-molecular-weight osmotic compounds, including Pro, to support osmotic balance under drought circumstances [44,45]. Our results demonstrated that drought-stressed barley genotypes accumulated a substantially high amount of Pro while retaining significantly less RWC in their leaves as compared with the ‘Control’ plants (Figs. 2A and 2B). These findings indicated that Pro accumulation in drought-exposed barley plants was insufficient to retain water under severe water-paucity situations, which is consistent with the findings of others [46,47]. On the other hand, SA supplementation to the drought-stressed plants further increased the levels of Pro, which corroborated with the results of improved leaf RWC under water dearth conditions (Figs. 2A and 2B). Similar to our results, a positive correlation between improved drought tolerance and the levels of Pro and leaf RWC has also been reported in wheat and mungbean plants [48,49]. In Brassica napus, it was reported that SA-treated drought plants showed 1.4 times elevation of the expression of proline biosynthetic genes, including 1-pyrroline-5-carboxylate synthetase (P5CS1 and P5CS2) and pyrroline-5-carboxylate reductase (P5CR) when compared with drought-stressed only plants [50]. Accordingly, the PCA biplot also exhibited a positive relationship between the SA-added drought-stressed barley plants with the increased levels of Pro and leaf RWC (Figs. 5C and 5D).
A plethora of studies reported that drought-induced biomass reduction was interlinked with the induction of oxidative damage due to increased production of ROS, including O2•− and H2O2 [37,51–53]. In the present study, we observed that drought stress led to a greater accumulation of O2•− and H2O2, and subsequently, MDA levels in the leaves of both barley genotypes (Figs. 3A–3C), implying that barely plants suffered from severe oxidative stress and membrane damage as a result of drought stress. On the other hand, exogenous application of SA to drought-stressed barley plants helped to reduce the levels of O2•−, H2O2, and MDA in their leaves (Figs. 3A–3C), suggesting that SA played a decisive role in minimizing the ROS-induced oxidative stress burden and protecting the cell membrane integrity from drought-induced damage. In support of these findings, PCA results showed that SA-treated drought-exposed barley plants exhibited a decreased extent of positive correlation with these ROS products, as well as MDA levels when compared with drought-stressed barley plants that were not treated with SA (Figs. 5C and 5D).
Plants have developed a robust antioxidant defense system to combat ROS-mediated oxidative burden when exposed to drought [47,54,55]. In the current study, drought-stressed barley plants supplemented with SA displayed further enhancement of the activities of antioxidant enzymes, including SOD, CAT, APX, POD and GPX, when compared with the SA-devoid drought-stressed barley plants (Figs. 4A–4E). Fundamentally, increased SOD activity in the leaves of drought-exposed barley plants treated with SA corroborated with the reduction of O2•− levels (Fig. 3A), as SOD is known to catalyze the dismutation of O2•− to H2O2 [56,57]. It has also been reported that foliar application of SA improved the expression of SOD encoding gene Cu/ZnSOD in Impatiens walleriana plants under drought stress [58]. CAT, APX and POD are involved in the detoxification of H2O2 [59]. Accordingly, the reduced level of H2O2 in SA-treated drought-exposed barley plants also coincided with the elevated activities of CAT, APX and POD (Figs. 3B, and 4B–4D). Furthermore, under drought conditions, the increased activity of GPX in the leaves of barley genotypes supplemented with SA provided indirect evidence of the likely involvement of SA in boosting the GSH-dependent peroxide-detoxification system. Similar to our findings, numerous studies reported the potential functions of SA in activating ROS-detoxification mechanisms in plants under water shortage conditions [60–63]. It was observed that drought stress increased the expression of the SA-inducible genes like pathogenesis-related (PR)-1 and PR-2 [64], indicating that SA is required to mount drought tolerance potential in plants. Moreover, SA also interacted with other phytohormones like abscisic acid (ABA) and jasmonic acid (JA) in regulating water stress tolerance in tomato plants [65]. Our results were also supported by the PCA, which showed a significant positive association of the SA-supplemented drought-stressed plants with the activities of enzymatic antioxidants (Figs. 5C and 5D).
In summary, our results suggested that supplementation of SA improved the drought tolerance in barley genotypes by improving plant biomass, Pro level and relative water content, as well as by reducing the levels of O2•−, H2O2 and MDA through the activation of several key antioxidant enzymes, such as SOD, CAT, APX, POD and GPX. Among the two studied barley genotypes, BB-5 performed better in the presence of SA to resist drought-caused adverse effects. Overall, our results demonstrate the potential effects of SA in alleviating drought-induced damage to an economically important crop, barley. To ascertain the beneficial impact of SA in the effective management of the drought problem, more extensive field research using a variety of crop species under various drought regimes and modes of SA applications should be implemented. In addition, it would be intriguing to determine whether SA supplementation has any favorable impact on the biochemical components of barley seeds and their nutrient contents, which could help us better address the problem of malnutrition in developing nations.
Funding Statement: The authors received no specific funding for this study.
Author Contributions: S.M.N.I. and M.A.H. designed and supervised the experiment. N.P. performed the experiment, harvested plant materials and carried out the analysis. M.M.R. and M.G.M. analyzed the data and prepared the figures. M.G.M., S.M.N.I. and M.M.R. drafted the manuscript. N.P. and M.M. performed biochemical analysis and analyzed data. All authors revised and finalized the manuscript.
Conflicts of Interest: The authors declare that they have no conflicts of interest to report regarding the present study.
References
1. Pennisi, E. (2008). Getting to the root of drought responses. Science, 320(5873), 173. [Google Scholar]
2. Ceccarelli, S., Grando, S., Maatougui, M., Michael, M., Slash, M. et al. (2010). Plant breeding and climate changes. The Journal of Agricultural Science, 148(6), 627–637. [Google Scholar]
3. Alghabari, F., Ihsan, M. Z. (2018). Effects of drought stress on growth, grain filling duration, yield and quality attributes of barley (Hordeum vulgare L.). Bangladesh Journal of Botany, 47(3), 421–428. [Google Scholar]
4. Gill, S. S., Tuteja, N. (2010). Reactive oxygen species and antioxidant machinery in abiotic stress tolerance in crop plants. Plant Physiology and Biochemistry, 48(12), 909–930. [Google Scholar] [PubMed]
5. Rahman, A., Mostofa, M. G., Nahar, K., Hasanuzzaman, M., Fujita, M. (2016). Exogenous calcium alleviates cadmium-induced oxidative stress in rice (Oryza sativa L.) seedlings by regulating the antioxidant defense and glyoxalase systems. Brazilian Journal of Botany, 39(2), 393–407. [Google Scholar]
6. Noctor, G., Foyer, C. H. (1998). Ascorbate and glutathione: Keeping active oxygen under control. Annual Review of Plant Biology, 49(1), 249–279. [Google Scholar]
7. Noctor, G., Veljovic-Jovanovic, S. O. N. J. A., Driscoll, S., Novitskaya, L., Foyer, C. H. (2002). Drought and oxidative load in the leaves of C3 plants: A predominant role for photorespiration? Annals of Botany, 89(7), 841–850. [Google Scholar] [PubMed]
8. Apel, K., Hirt, H. (2004). Reactive oxygen species: Metabolism, oxidative stress, and signal transduction. Annual Review of Plant Biology, 55, 373–399. [Google Scholar] [PubMed]
9. Karim, M. M., Islam, M. A., Rana, M. R., Hossain, M. A., Kader, M. A. (2018). Screening of barley genotypes for drought tolerance based on culm reserves contribution to grain yield. Journal of the Bangladesh Agricultural University, 16(1), 62–66. [Google Scholar]
10. Sallam, A., Alqudah, A. M., Dawood, M. F., Baenziger, P. S., Börner, A. (2019). Drought stress tolerance in wheat and barley: Advances in physiology, breeding and genetics research. International Journal of Molecular Sciences, 20(13), 3137. [Google Scholar] [PubMed]
11. Arfan, M., Athar, H. R., Ashraf, M. (2007). Does exogenous application of salicylic acid through the rooting medium modulate growth and photosynthetic capacity in two differently adapted spring wheat genotypes under salt stress? Journal of Plant Physiology, 164(6), 685–694. https://doi.org/10.1016/j.jplph.2006.05.010 [Google Scholar] [PubMed] [CrossRef]
12. El-Tayeb, M. A. (2005). Response of barley grains to the interactive effect of salinity and salicylic acid. Plant Growth Regulation, 45(3), 215–224. https://doi.org/10.1007/s10725-005-4928-1 [Google Scholar] [CrossRef]
13. Szepesi, Á. (2005). Role of salicylic acid pre-treatment on the acclimation of tomato plants to salt-and osmotic stress. Acta Biologica Szegediensis, 49(1–2), 123–125. [Google Scholar]
14. Liu, C., Guo, J., Cui, Y., Lü, T., Zhang, X. et al. (2011). Effects of cadmium and salicylic acid on growth, spectral reflectance and photosynthesis of castor bean seedlings. Plant and Soil, 344(1), 131–141. https://doi.org/10.1007/s11104-011-0733-y [Google Scholar] [CrossRef]
15. Kadioglu, A., Saruhan, N., Sağlam, A., Terzi, R., Acet, T. (2011). Exogenous salicylic acid alleviates effects of long term drought stress and delays leaf rolling by inducing antioxidant system. Plant Growth Regulation, 64(1), 27–37. https://doi.org/10.1007/s10725-010-9532-3 [Google Scholar] [CrossRef]
16. Pedranzani, H., Vigliocco, A. (2017). Regulation of jasmonic acid and salicylic acid levels in abiotic stress tolerance, past and present. In: Singh, V. P., Singh, S., Prasad, S. M. (Eds.Mechanisms behind phytohormonal signalling and crop abiotic stress tolerance, pp. 329–370. New York, NY: Nova Science Publishers. [Google Scholar]
17. Mostofa, M. G., Fujita, M. (2013). Salicylic acid alleviates copper toxicity in rice (Oryza sativa L.) seedlings by up-regulating antioxidative and glyoxalase systems. Ecotoxicology, 22(6), 959–973. [Google Scholar] [PubMed]
18. Yusuf, M., Hasan, S. A., Ali, B., Hayat, S., Fariduddin, Q. et al. (2008). Effect of salicylic acid on salinity-induced changes in Brassica juncea. Journal of Integrative Plant Biology, 50(9), 1096–1102. [Google Scholar] [PubMed]
19. Hussein, M. M., Balbaa, L. K., Gaballah, M. S. (2007). Salicylic acid and salinity effects on growth of maize plants. Research Journal of Agriculture and Biological Sciences, 3(4), 321–328. [Google Scholar]
20. Vanacker, H., Lu, H., Rate, D. N., Greenberg, J. T. (2001). A role for salicylic acid and NPR1 in regulating cell growth in Arabidopsis. The Plant Journal, 28(2), 209–216. [Google Scholar] [PubMed]
21. Horváth, E., Szalai, G., Janda, T. (2007). Induction of abiotic stress tolerance by salicylic acid signaling. Journal of Plant Growth Regulation, 26(3), 290–300. [Google Scholar]
22. Pasala, R. K., Khan, M. I. R., Minhas, P. S., Farooq, M. A., Sultana, R. et al. (2016). Can plant bio-regulators minimize crop productivity losses caused by drought, heat and salinity stress? An integrated review. Journal of Applied Botany and Food Quality, 89, 113–125. [Google Scholar]
23. Das, A. K., Anik, T. R., Rahman, M. M., Keya, S. S., Islam, M. R. et al. (2022). Ethanol treatment enhances physiological and biochemical responses to mitigate saline toxicity in soybean. Plants, 11(3), 272. https://doi.org/10.3390/plants11030272 [Google Scholar] [PubMed] [CrossRef]
24. Bates, L. S., Waldren, R. P., Teare, I. D. (1973). Rapid determination of free proline for water-stress studies. Plant and Soil, 39(1), 205–207. https://doi.org/10.1007/BF00018060 [Google Scholar] [CrossRef]
25. Elstner, E. F., Heupel, A. (1976). Inhibition of nitrite formation from hydroxylammonium chloride: A simple assay for superoxide dismutase. Analytical Biochemistry, 70(2), 616–620. https://doi.org/10.1016/0003-2697(76)90488-7 [Google Scholar] [PubMed] [CrossRef]
26. Yu, C. W., Murphy, T. M., Lin, C. H. (2003). Hydrogen peroxide-induced chilling tolerance in mung beans mediated through ABA-independent glutathione accumulation. Functional Plant Biology, 30(9), 955–963. https://doi.org/10.1071/FP03091 [Google Scholar] [PubMed] [CrossRef]
27. Heath, R. L., Packer, L. (1968). Photoperoxidation in isolated chloroplasts: I. Kinetics and stoichiometry of fatty acid peroxidation. Archives of Biochemistry and Biophysics, 125(1), 189–198. https://doi.org/10.1016/0003-9861(68)90654-1 [Google Scholar] [PubMed] [CrossRef]
28. Beyer Jr, W. F., Fridovich, I. (1987). Assaying for superoxide dismutase activity: Some large consequences of minor changes in conditions. Analytical Biochemistry, 161(2), 559–566. https://doi.org/10.1016/0003-2697(87)90489-1 [Google Scholar] [PubMed] [CrossRef]
29. Hemeda, H. M., Klein, B. P. (1990). Effects of naturally occurring antioxidants on peroxidase activity of vegetable extracts. Journal of Food Science, 55(1), 184–185. https://doi.org/10.1111/j.1365-2621.1990.tb06048.x [Google Scholar] [CrossRef]
30. Csiszár, J., Lantos, E., Tari, I., Madosa, E., Wodala, B. et al. (2007). Antioxidant enzyme activities in allium species and their genotypes under water stress. Plant Soil and Environment, 53(12), 517–523. https://doi.org/10.17221/2192-PSE [Google Scholar] [CrossRef]
31. Nakano, Y., Asada, K. (1981). Hydrogen peroxide is scavenged by ascorbate-specific peroxidase in spinach chloroplasts. Plant and Cell Physiology, 22(5), 867–880. [Google Scholar]
32. Elia, A. C., Galarini, R., Taticchi, M. I., Dörr, A. J. M., Mantilacci, L. (2003). Antioxidant responses and bioaccumulation in Ictalurus melas under mercury exposure. Ecotoxicology and Environmental Safety, 55(2), 162–167. [Google Scholar] [PubMed]
33. Bradford, M. M. (1976). A rapid and sensitive method for the quantitation of microgram quantities of protein utilizing the principle of protein-dye binding. Analytical Biochemistry, 72(1–2), 248–254. [Google Scholar] [PubMed]
34. R Core Team (2016). Vienna: R Foundation for Statistical Computing. [Google Scholar]
35. Panda, D., Mishra, S. S., Behera, P. K. (2021). Drought tolerance in rice: Focus on recent mechanisms and approaches. Rice Science, 28(2), 119–132. [Google Scholar]
36. Ahmad, S., Muhammad, I., Wang, G. Y., Zeeshan, M., Yang, L. et al. (2021). Ameliorative effect of melatonin improves drought tolerance by regulating growth, photosynthetic traits and leaf ultrastructure of maize seedlings. BMC Plant Biology, 21(1), 1–14. [Google Scholar]
37. Kirova, E., Pecheva, D., Simova-Stoilova, L. (2021). Drought response in winter wheat: Protection from oxidative stress and mutagenesis effect. Acta Physiologiae Plantarum, 43(1), 1–11. [Google Scholar]
38. Sabagh, A. E., Hossain, A., Islam, M. S., Barutcular, C., Hussain, S. et al. (2019). Drought and salinity stresses in barley: Consequences and mitigation strategies. Australian Journal of Crop Science, 13(6), 810–820. [Google Scholar]
39. Saleem, M., Fariduddin, Q., Janda, T. (2021). Multifaceted role of salicylic acid in combating cold stress in plants: A review. Journal of Plant Growth Regulation, 40(2), 464–485. https://doi.org/10.1007/s00344-020-10152-x [Google Scholar] [CrossRef]
40. Liu, J., Qiu, G., Liu, C., Li, H., Chen, X. et al. (2022). Salicylic acid, a multifaceted hormone, combats abiotic stresses in plants. Life, 12(6), 886. https://doi.org/10.3390/life12060886 [Google Scholar] [PubMed] [CrossRef]
41. Khan, M. I., Poor, P., Janda, T. (2022). Salicylic acid: A versatile signaling molecule in plants. Journal of Plant Growth Regulation, 41(5), 1–4. https://doi.org/10.1007/s00344-022-10692-4 [Google Scholar] [CrossRef]
42. Azmat, A., Yasmin, H., Hassan, M. N., Nosheen, A., Naz, R. et al. (2020). Co-application of bio-fertilizer and salicylic acid improves growth, photosynthetic pigments and stress tolerance in wheat under drought stress. PeerJ, 8(1), e9960. https://doi.org/10.7717/peerj.9960 [Google Scholar] [PubMed] [CrossRef]
43. Nawaz, M., Ishaq, S., Ishaq, H., Khan, N., Iqbal, N. et al. (2020). Salicylic acid improves boron toxicity tolerance by modulating the physio-biochemical characteristics of maize (Zea mays L.) at an early growth stage. Agronomy, 10(12), 2013. [Google Scholar]
44. Hayat, S., Hayat, Q., Alyemeni, M. N., Wani, A. S., Pichtel, J. et al. (2012). Role of proline under changing environments: A review. Plant Signaling & Behavior, 7(11), 1456–1466. [Google Scholar]
45. Zulfiqar, F., Akram, N. A., Ashraf, M. (2020). Osmoprotection in plants under abiotic stresses: New insights into a classical phenomenon. Planta, 251(1), 1–17. [Google Scholar]
46. Dien, D. C., Thu, T. T. P., Moe, K., Yamakawa, T. (2019). Proline and carbohydrate metabolism in rice varieties (Oryza sativa L.) under various drought and recovery conditions. Plant Physiology Reports, 24(3), 376–387. [Google Scholar]
47. Rahman, M., Mostofa, M. G., Keya, S. S., Rahman, A., Das, A. K. et al. (2021). Acetic acid improves drought acclimation in soybean: An integrative response of photosynthesis, osmoregulation, mineral uptake and antioxidant defense. Physiologia Plantarum, 172(2), 334–350. [Google Scholar] [PubMed]
48. Altaf, A., Gull, S., Zhu, X., Zhu, M., Rasool, G. et al. (2021). Study of the effect of peg-6000 imposed drought stress on wheat (Triticum aestivum L.) cultivars using relative water content (RWC) and proline content analysis. Pakistan Journal of Agricultural Sciences, 58(1), 356–357. [Google Scholar]
49. Bangar, P., Chaudhury, A., Tiwari, B., Kumar, S., Kumari, R. et al. (2019). Morphophysiological and biochemical response of mungbean [Vigna radiata (L.) Wilczek] varieties at different developmental stages under drought stress. Turkish Journal of Biology, 43(1), 58–69. [Google Scholar] [PubMed]
50. La, V. H., Lee, B. R., Islam, M. T., Park, S. H., Jung, H. I. et al. (2019). Characterization of salicylic acid-mediated modulation of the drought stress responses: Reactive oxygen species, proline, and redox state in Brassica napus. Environmental and Experimental Botany, 157, 1–10. [Google Scholar]
51. Noctor, G., Mhamdi, A., Foyer, C. H. (2014). The roles of reactive oxygen metabolism in drought: Not so cut and dried. Plant Physiology, 164(4), 1636–1648. [Google Scholar] [PubMed]
52. Mukarram, M., Choudhary, S., Kurjak, D., Petek, A., Khan, M. M. A. (2021). Drought: Sensing, signalling, effects and tolerance in higher plants. Physiologia Plantarum, 172(2), 1291–1300. [Google Scholar] [PubMed]
53. Kerchev, P. I., van Breusegem, F. (2022). Improving oxidative stress resilience in plants. The Plant Journal, 109(2), 359–372. https://doi.org/10.1111/tpj.15493 [Google Scholar] [PubMed] [CrossRef]
54. Laxa, M., Liebthal, M., Telman, W., Chibani, K., Dietz, K. J. (2019). The role of the plant antioxidant system in drought tolerance. Antioxidants, 8(4), 94. https://doi.org/10.3390/antiox8040094 [Google Scholar] [PubMed] [CrossRef]
55. Mostofa, M. G., Rahman, M. M., Siddiqui, M. N., Fujita, M., Tran, L. S. P. (2020). Salicylic acid antagonizes selenium phytotoxicity in rice: Selenium homeostasis, oxidative stress metabolism and methylglyoxal detoxification. Journal of Hazardous Materials, 394, 122572. https://doi.org/10.1016/j.jhazmat.2020.122572 [Google Scholar] [PubMed] [CrossRef]
56. Czarnocka, W., Karpiński, S. (2018). Friend or foe? Reactive oxygen species production, scavenging and signaling in plant response to environmental stresses. Free Radical Biology and Medicine, 122, 4–20. https://doi.org/10.1016/j.freeradbiomed.2018.01.011 [Google Scholar] [PubMed] [CrossRef]
57. Mostofa, M. G., Rahman, M. M., Ansary, M. M. U., Keya, S. S., Abdelrahman, M. et al. (2021). Silicon in mitigation of abiotic stress-induced oxidative damage in plants. Critical Reviews in Biotechnology, 41(6), 918–934. https://doi.org/10.1080/07388551.2021.1892582 [Google Scholar] [PubMed] [CrossRef]
58. Antonić, D. D., Subotić, A. R., Dragićević, M. B., Pantelić, D., Milošević, S. M. et al. (2020). Effects of exogenous salicylic acid on drought response and characterization of dehydrins in Impatiens walleriana. Plants, 9(11), 1589. [Google Scholar] [PubMed]
59. Dumanović, J., Nepovimova, E., Natić, M., Kuča, K., Jaćević, V. (2021). The significance of reactive oxygen species and antioxidant defense system in plants: A concise overview. Frontiers in Plant Science, 11, 552969. [Google Scholar] [PubMed]
60. Shemi, R., Wang, R., Gheith, E. S., Hussain, H. A., Hussain, S. et al. (2021). Effects of salicylic acid, zinc and glycine betaine on morpho-physiological growth and yield of maize under drought stress. Scientific Reports, 11(1), 1–14. [Google Scholar]
61. Yadav, T., Kumar, A., Yadav, R. K., Yadav, G., Kumar, R. et al. (2020). Salicylic acid and thiourea mitigate the salinity and drought stress on physiological traits governing yield in pearl millet-wheat. Saudi Journal of Biological Sciences, 27(8), 2010–2017. [Google Scholar] [PubMed]
62. Abdelaal, K. A., Attia, K. A., Alamery, S. F., El-Afry, M. M., Ghazy, A. I. et al. (2020). Exogenous application of proline and salicylic acid can mitigate the injurious impacts of drought stress on barley plants associated with physiological and histological characters. Sustainability, 12(5), 1736. [Google Scholar]
63. Saleem, M., Fariduddin, Q., Castroverde, C. D. M. (2021). Salicylic acid: A key regulator of redox signalling and plant immunity. Plant Physiology and Biochemistry, 168, 381–397. [Google Scholar] [PubMed]
64. Miura, K., Okamoto, H., Okuma, E., Shiba, H., Kamada, H. et al. (2013). SIZ1 deficiency causes reduced stomatal aperture and enhanced drought tolerance via controlling salicylic acid-induced accumulation of reactive oxygen species in Arabidopsis. The Plant Journal, 73, 91–104. [Google Scholar] [PubMed]
65. Muñoz-Espinoza, V. A., López-Climent, M. F., Casaretto, J. A., Gómez-Cadenas, A. (2015). Water stress responses of tomato mutants impaired in hormone biosynthesis reveal abscisic acid, jasmonic acid and salicylic acid interactions. Frontiers in Plant Science, 6, 997. [Google Scholar]
Supplementary File 1:Data used in the ANOVA analysis
For single variety
BARI Barley-5 (BB-5)
BARI Barley-7 (BB-7)
For interaction between treatments and varieties
Cite This Article
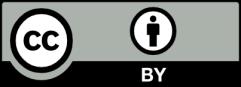
This work is licensed under a Creative Commons Attribution 4.0 International License , which permits unrestricted use, distribution, and reproduction in any medium, provided the original work is properly cited.