Open Access
ARTICLE
β-Cyclodextrin-Based Nitrosoglutathione Improves the Storage Quality of Peach by Regulating the Metabolism of Endogenous Nitric Oxide, Hydrogen Sulfide, and Phenylpropane
College of Chemistry and Material Science, Shandong Agricultural University, Taian, 271000, China
* Corresponding Author: Shuhua Zhu. Email:
(This article belongs to the Special Issue: Effect of Abiotic Stress Treatments on the Antioxidants Activities, Secondary Metabolites and Postharvest Physico-Chemical Properties of Fruits and Vegetables)
Phyton-International Journal of Experimental Botany 2023, 92(4), 1091-1107. https://doi.org/10.32604/phyton.2023.026331
Received 30 August 2022; Accepted 08 October 2022; Issue published 06 January 2023
Abstract
Nitrosoglutathione (GSNO) and β-cyclodextrin (β-CD) exhibit positive roles in regulating fruit quality. However, there are few reports about the effects of GSNO and β-CD on enhancing storability and boosting nitric oxide (NO), hydrogen sulfide (H2S), and phenylpropane metabolism in fruits during storage. “Xintaihong” peach were treated with 0.5, 1.0, 1.5 mmol L−1 GSNO in 0.5% (w/v) β-CD solution (GSNO/β-CD). The effects of GSNO/β-CD on endogenous NO, H2S, and phenylpropane metabolism were investigated. Treatment with GSNO/β-CD increased the color difference of peach and inhibited the increase of respiratory intensity, weight loss, and relative conductivity. Treatment with 1.0 mmol L−1 GSNO/β-CD increased the nitric oxide synthase (NOS-like) activity and L-arginine content, thereby promoting the accumulation of endogenous NO. By improving the activities of L-cysteine desulfhydrylase (L-CD), O-acetylserine sulfur lyase (OAS-TL), serine acetyltransferase (SAT), GSNO/β-CD increased the content of endogenous H2S in peach. Treatment with GSNO/β-CD increased the activities of phenylalanine ammonia-lyase (PAL), 4-coumarate-CoA ligase (4CL), and cinnamic acid-4-hydroxylase (C4H), promoted the increase of total phenols, flavonoids, and lignin in peach. These results indicated that GSNO/β-CD treatment better maintained the quality of peach by improving the metabolism of endogenous NO, H2S, and phenylpropane during storage.Keywords
Peach (Prunus persica) fruit is vulnerable to damage during postharvest transportation and storage, becomes brown and decays, and is easily attacked by fungi and bacteria [1–3]. Low-temperature storage is commercially used to prolong the life and maintain the quality of peach. Nitric oxide (NO) and hydrogen sulfide (H2S) are small bioactive molecules in plants’ biophysical, biochemical, and signaling processes. NO is involved in seed germination [4], pollen growth [5,6], leaf senescence [7], and stomatal closure [8] in plants. The maintenance of NO homeostasis is crucial for plant physiological processes. Exogenous NO alleviates the oxidative damage of tomato seed germination and seedling development under chromium stress [9]. NO also regulates physiological processes such as disease resistance, chilling injury, ripening, and senescence of fruits and plays a vital role in prolonging the fruit storage period and maintaining fruit postharvest quality [10,11]. Moreover, NO has been shown to reduce mitochondrial oxidative damage during peach cold storage and improve antioxidant capacity [12]. The role of endogenous NO in the stress and disease resistance of higher plants has been gradually reported [13]. Several quality-enhancing exogenous treatments were also associated with changes in endogenous NO levels [14,15]. GABA maintains high levels of endogenous NO content to enhance the resistance of tomatoes to Botrytis cinerea [16]. UV-B increases the level of endogenous NO in mango fruit and alleviates chilling injury [17]. Therefore, stimulating the accumulation of endogenous NO is a critical link in activating the positive roles of NO in plants. H2S has signal transduction characteristics and participates in the maintenance of reactive oxygen species (ROS) homeostasis to regulate the ripening, senescence, and disease resistance of fruits and vegetables [18,19]. In addition, the effect of H2S on prolonging the shelf life of broccoli [20], lotus root [21], and kiwifruits [22] have also been confirmed. The endogenous H2S accumulation has beneficial effects in ameliorating chilling injury and delaying aging [23,24]. Notably, H2S released from endogenous sulfides confer stress resistance in plants, resulting in substances that inhibit bacterial growth [25]. Delayed postharvest senescence of spinach leaves by NaHS treatment is also attributed to higher endogenous H2S accumulation [26]. It has been confirmed that H2S and NO have crosstalk in plants, and this crosstalk can be achieved by H2S and NO regulating each other’s metabolic enzymes. Exogenous application of GSNO significantly enhanced the L-CD activity in wheat, leading to the additional synthesis of H2S [27]. Wang et al. [28] found that exogenous H2S can also promote the accumulation of endogenous NO, thereby enhancing the salt tolerance of alfalfa. These studies demonstrated that the crosstalk between H2S and NO may be closely related to plant responses to stress.
The synthesis and transformation of secondary metabolites in plants are the biochemical basis of plant responses to abiotic stress [29]. The phenylpropane metabolism is a crucial pathway for producing these secondary metabolites [30]. However, during fruit ripening and senescence, the reduction of phenylpropane metabolites leads to a progressive decrease in defense capacity. The production of secondary metabolites can be accelerated by regulating the activities of key enzymes in the phenylpropane pathway, thereby improving fruit quality and disease resistance [31]. For example, methyl jasmonate and salicylic acid treatment maintain the quality of sweet cherries by promoting phenylpropane metabolism [32]. Treatment with acibenzolar-S-methyl increases the content of phenylpropanoid compounds and the activity of the main enzymes of the phenylpropane pathway in melon, thereby preventing the invasion of fungi [33]. β-Aminobutyric acid can promote the accumulation of polyphenols and lignin, accelerate potato tuber wound healing and increase disease resistance [34]. In addition, NO, as an exogenous inducer, activates the phenylpropanoid pathway in peach and induces disease resistance to Monilinia fructicola [35].
Nitrosoglutathione (GSNO) is an S-nitrosylated glutathione derivative, which stores and releases NO in cells as a NO donor [36]. GSNO modulates ROS balance and improves banana plants’ tolerance to Fusarium oxysporum [37]. However, GSNO as a NO donor has low molecular weight and poor thermal and photochemical stability [38]. To increase this stability, GSNO can be incorporated into the polymer matrix. For example, GSNO-chitosan nanoparticles enhance the antioxidant capacity of fresh-cut apples, and the effect is better than that of GSNO treatment [39]. The addition of GSNO in nanoparticles effectively improves the drought tolerance of sugarcane compared to the single application of GSNO [40]. β-Cyclodextrin (β-CD) is a cyclic glucose oligomer that can form complexes with many organic and inorganic molecules as the host [41]. Due to the lipophilic inner cavity and hydrophilic outer surface structure of β-CD, the hydrophilicity and stability of its binding partner are increased [42]. Previous studies have confirmed that S-nitrosothiol-modified cyclodextrin (CD) systems are capable of NO release [43]. Therefore, in this paper, β-CD was used as the polymer matrix, on which GSNO was added with different concentrations. Then, peach were used as the test material to study the effects of β-CD synergistic treatment with different concentrations of GSNO on postharvest quality, nitric oxide, hydrogen sulfide, and phenylpropane metabolism.
2.1 Plant Materials and Treatments
“Xintaihong” peach were harvested at the physiologically mature stage (about 70%–80% mature, with an average firmness of about 48 ± 1.1 N and soluble sugar content of about 9.8 ± 0.5 °Brix) from the orchard of Xintai City, Shandong Province. Peach with no diseases, no insect pests and no mechanical damage were selected and precooled at 0°C for 24 h, then were soaked in 0.5% (w/v) β-CD (the optimal concentration of pre-experiment treatment) as the control, 0.5 mmol L−1 GSNO + 0.5% (w/v) β-CD, 1.0 mmol L−1 GSNO + 0.5% (w/v) β-CD and 1.5 mmol L−1 GSNO + 0.5% (w/v) β-CD for 30 min, respectively (the temperature was about 20°C). After drying with cool air, the peach were stored at 0°C. The initial samples before treatment were represented as a sample on day 0.
2.2 Measurements of Firmness and the Soluble Solids Content
The firmness of peach fruit was measured by a GY-4 fruit firmness tester (Shanghai, China) with a probe diameter of 11 mm. The firmness value was expressed as the average value of the maximum force of each fruit measured at three points at the equator, and the unit was N.
The solid soluble content (SSC) of peach fruit was determined by a WY015R refractometer (Shanghai Cany Precision Instrument Co., Ltd., China) and was expressed in °Brix.
The exterior color of peach was measured using a CR-10 Colorimeter (Konica Minolta Holdings, Inc., Japan). Nine peach in each group were used for measurement. The colorimeter measured three values of L, a, b, where “L” represents lightness or darkness. “a” represents the red and green degree, and “b” represents the yellow and blue degree. “L0”, “a0”, “b0” represent the data of peach on day 0. ΔE represented the color difference value of peach.
ΔE=√(L−L0)2+(b−b0)2+(a−a0)2 (1)
About 1 mm thick peel was peeled off from the peach fruit. The round slices with a diameter of 15 mm and a thickness of about 1 mm were randomly taken from different parts of the mesocarps. Four peach were randomly selected from each treatment, and 3 peach slices were randomly selected from each peach. Twelve pieces of peach slides were dipped in 40 mL of double distilled water. The conductivities were measured after the peach slides were immediately dipped (P0), after incubated for 10 min (P1), and after the boiling bath for 10 min and cooled (P2), respectively. The relative conductivity was calculated with the following formula:
P=P1−P0P2−P0×100% (2)
2.5 Measurement of Weight Loss Rate and Respiratory Intensity
The fresh weight of peach was measured using the balance:
Weightloss=W1−W2W1×100% (3)
where W1 is the weight at day 0, W2 is the weight on the sampled day. The respiratory intensity of peach was measured with the SY-1022 gas analyzer (Shiya Technology Co. China). Respiration intensity represents the milligram value of carbon dioxide exhaled per kilogram of sample per hour and expressed as mg CO2 kg−1 h−1.
2.6 Determination of Related Indexes of Endogenous NO Metabolism
The NO Assay Kit (Nanjing Jiancheng Biological Engineering Co., Ltd., China.) was used to determine NO content. The NO content was calculated according to the method in the manual. NO content was expressed as µmol g−1 protein.
According to Zhang et al. [44], the content of L-arginine and nitrite, the activities of nitrite reductase (NR) and nitric oxide synthase (NOS-like) were determined. The absorbance at 530 nm was used to determine the L-arginine content, and standard arginine solution was used as the standard curve, expressed as nmol g−1 FW. The absorbance at 540 nm was measured. The content of nitrite was expressed as µmol g−1 FW. NR activity was expressed as nmol NO g−1 protein, and NOS-like activity was expressed as µmol NO g−1 protein.
2.7 Determination of the Content of Endogenous H2S
The content of endogenous H2S was determined according to Chen et al. [45]. The absorbance at 670 nm was measured. The content of endogenous H2S was calculated with a calibration curve with Na2S and expressed as μmol g−1 FW.
2.8 Determination of Activities of Enzymes Related to H2S Metabolism
According to Huang et al. [46], the activities of L-cysteine demethylase (L-CD), O-acetylserine sulfur lyase (OAS-TL), and serine acetyltransferase (SAT) were determined by the absorbance at 670, 562, and 560 nm. The change in absorbance value per unit time was 0.01 as one enzyme activity unit (U), expressed as U g−1 protein.
2.9 Determination of Enzyme Activities Related to the Phenol Metabolism
The phenylalanine ammonia-lyase (PAL) activity was determined by the method of Aghdam et al. [47]. The absorbance at 290 nm was recorded as A0. After terminating the reaction, the absorbance at 290 nm was measured again and recorded as A1. One unit (U) of enzyme activity was defined as the difference between A1 and A0. The unit of PAL activity was U g−1 protein.
The activities of 4-Coumaric acid-CoA ligase (4CL) and cinnamic acid 4-hydroxylase (C4H) were determined by the method of Gao et al. [48]. After the reaction was completed, the absorbance value at 333 nm was measured. One unit (U) of enzyme activity was defined as an absorbance change of 0.01 per minute, and the 4CL activity was expressed as U g−1 protein. For the cinnamic acid 4-hydroxylase (C4H) activity, the absorbance at 340 nm was determined, with distilled water as the blank reference. The results were expressed as U·g−1 protein, where U was defined as an absorbance change of 0.01 per hour.
2.10 Determination of Total Phenols, Flavonoids, and Lignin Content
The total phenol content was determined by the method of Zargoosh et al. [49]. The absorbance at 760 nm was measured, and a standard curve was drawn using various concentration gradients of water and gallic acid. The total phenol content was expressed as mg g−1 FW.
The flavonoid content was determined by the method of Angmo et al. [50]. Sample extraction refers to the sample extraction of total phenol. Finally, the absorbance at 510 nm was measured, and the standard curve was determined with rutin. The content of flavonoids was expressed as mg g−1 FW.
The lignin content was determined by the method of Zhang et al. [51]. After mixing the extract with glacial acetic acid, the absorbance of the sample at 280 nm was measured. The lignin content was expressed as OD280 g−1 FW. The lignin content was calculated as follows:
lignin=Abs×litersWsample×Astandard×100% (4)
where Abs is the absorbance of the sample solution at 280 nm; liters is the volume of the sample solution at constant volume (L); W sample is the weight of the sample (g); A standard is the standard absorbance of Arabidopsis lignin 17.2.
Data were analyzed by a least significant difference (Duncan’s test) analysis with differences significant at p < 0.05.
3.1 Peach Fruit from Different Treatments after 25 Days of Storage
Fig. 1 indicates the appearance of peach fruit from the different treatments after 25 days of storage. The fruit treated with 1.0 mmol L−1 GSNO/β-CD maintained a good morphological appearance of the peach fruit throughout storage. The control and other treated peach showed weight loss and rotted to different degrees.
Figure 1: Appearance of the peach in different treatments after 25 days of storage
3.2 Changes in the Characteristics of Peach
The overall firmness of peach showed a downward trend (Fig. 2A). Treatment with 1.0 mmol L−1 GSNO/β-CD delayed the decrease of peach fruit firmness and was significantly different from the other treatments between day 10 and day 20. GSNO/β-CD treatment had an apparent inhibitory effect on the increase of solid soluble content (SSC) in peach (Fig. 2B). The inhibitory effect of 1.0 mmol L−1 GSNO/β-CD was the most significant, and the solid soluble content was always lower than other treatments. The ΔE value of all treatments showed a continuous upward trend (Fig. 2C). However, peach treated with GSNO/β-CD had a lower ΔE value at the storage end. The results showed that the GSNO/β-CD treatments alleviated the changes in ΔE value and contributed to color maintenance in the peach. The inhibitory effect of 1.0 mmol L−1 GSNO/β-CD treatment on the increase in relative conductivity during peach storage was observed only on days 5, 10, and 25 (Fig. 2D). Other than that, there was no significant difference between the other treatments and the control.
Figure 2: Firmness (A), SSC (B), ΔE (C), relative conductivity (D), the weight loss rate (E), and the respiration rate (F) of peach during storage
The weight loss rate increased gradually with storage time in both the control and the GSNO/β-CD treatments (Fig. 2E). On day 25, the water loss rate of peach in control was 32%, which was 1.10 times and 1.23 times that of the 0.5 mmol L−1 GSNO/β-CD and 1.0 mmol L−1 GSNO/β-CD treatments. The GSNO/β-CD treated samples had consistently less weight loss than the control because the GSNO/β-CD reduced respiration rates. The respiration rate of peach showed an increasing trend at first, then exhibited a decreasing trend and reached a peak on day 20. The respiration rate of the peach treated with 1.0 mmol L−1 GSNO/β-CD was reduced by around 35% at the end of storage (Fig. 2F). Thus, it suggested that more effect of 1.0 mmol L−1 GSNO/β-CD on respiration and fruit quality could be expected.
The NR activity increased in the first 20 days during storage (Fig. 3A), and treatments with GSNO/β-CD inhibited the increase in NR activity during storage. Only on days 5 and 20, NR activity of the 1.5 mmol L–1 GSNO/β-CD was higher than the control. The nitrite content in the peach showed an overall upward trend (Fig. 3C). In the first 15 days, different concentrations of GSNO/β-CD treatment increased the nitrite contents of peach. Thereafter, there was no significant change in the nitrite content between treatments with GSNO/β-CD and the control.
Figure 3: Activities of NR (A) and NOS (B) and contents of nitrate (C), L-arginine (D), and endogenous NO (E) in peach during storage
The NOS-like activity and L-arginine content showed an upward trend followed by a downward trend (Figs. 3B, 3D). The NOS-like activity of 1.0 mmol L−1 GSNO/β-CD treated peach maintained a high level, 1.66 times higher than the control on day 20. Also, on day 20 of storage, the L-arginine content in peach treated with 1.0 mmol L−1 GSNO/β-CD was 1.3 times that of the control and higher than other treatments.
The content of the endogenous NO in peach of each treatment showed a trend of slow increase first and then rapid decrease, and reached the peak on the 15th day (Fig. 3E). The content of endogenous NO in peach treated with 0.5, 1.0 mmol L−1 GSNO/β-CD always maintained a high level. The NO content in peach treated with 1.5 mmol L−1 GSNO/β-CD was higher than in control except for day 15. The content of endogenous NO in peach treated with 1.0 mmol L−1 GSNO/β-CD was 1.14 and 1.46 times that of the control on days 15 and 25, respectively. It showed that 1.0 mmol L−1 GSNO/β-CD treatment effectively increased the endogenous NO content in peach.
The L-CD activity peaked on day 20 (Fig. 4A). The L-CD activity of the control was the lowest among all treatments with GSNO/β-CD. The activities of both OAS-TL and SAT increased during storage (Figs. 4B and 4C). The activities of SAT and OAS-TL in peach treated with GSNO/β-CD were maintained at a high level, and 1.0 mmol L−1 GSNO/β-CD treatment had the best effect. Compared with the control, the activities of SAT and OAS-TL in peach treated with 1.0 mmol/L GSNO/β-CD increased by 184% and 52% at the storage end. As shown in Fig. 4D, the content of endogenous H2S in peach continued to accumulate with time in the first 20 days of storage. The peach treated with 1.0 mmol L−1 GSNO/β-CD had the highest endogenous H2S content. It showed that GSNO/β-CD treatment could promote the synthesis of endogenous H2S in peach by enhancing the activities of enzymes related to H2S metabolism.
Figure 4: Activities of L-CD (A), SAT (B), OAS-TL (C), and H2S content (D) in peach during storage
As shown in Fig. 5A, GSNO/β-CD treatment increased PAL activity in peach. On day 20, the PAL activities in peach treated with 0.5, 1.0, and 1.5 mmol L−1 GSNO/β-CD were 1.17, 1.36, and 1.06 times that of the control. Among them, 1.0 mmol L−1 GSNO/β-CD treatment had the best effect.
Figure 5: Activities of PAL (A), 4CL (B), and C4H (C) and contents of total phenol (D), flavonoids (E), and lignin (F) in peach during storage
The activities of C4H and 4CL in each treatment showed a trend of increasing and decreasing slowly, reaching the highest enzyme activity value on day 20 (Figs. 5B, 5C). treatment significantly promoted the activities of C4H and 4CL in peach. On the last day of storage, the C4H and 4CL activities of peach treated with 1.0 mmol L−1 GSNO/β-CD were 34% and 25% higher than those of the control.
The total phenolic content showed a unimodal trend and increased sharply from the first day of 1.0 mmol L−1 GSNO/β-CD treatment, which was much higher than other treatments (Fig. 5D). Flavonoid content was also 1.21-fold higher than controls on day 20 of 1.0 mmol L−1 GSNO/β-CD treatment (Fig. 5E). As shown in Fig. 5F, GSNO/β-CD treatment increased the lignin content of peach during storage. At the end of storage, the lignin contents in peach treated with 0.5, 1.0, and 1.5 mmol L−1 GSNO/β-CD were 1.14, 1.23, and 1.05 times that of the control. These results suggested that, compared to other treatments, treatment with 1.0 mmol L−1 GSNO/β-CD significantly promoted the phenylpropane pathway of peach during storage.
3.6 Correlation Analysis of Indicators Related to Quality during Peach Storage
Fig. 6 showed the correlation between the enzyme activities and substance contents of NO, H2S, and phenylpropane metabolism during the storage of peach fruit. Red indicated a positive correlation, and the darker the color, the more significant the correlation. It could be seen that NOS-like, the key enzyme of NO metabolism, significantly correlated with the contents of endogenous NO and L-arginine. The increase of enzyme activities such as L-CD and SAT also had a significant positive correlation with the increase of H2S content. Similarly, the increase of phenylpropane metabolites was also associated with the increase of PAL, 4CL, and C4H enzymatic activities. The changes in these indicators were the reasons for the quality changes of peach during storage.
Figure 6: Correlation analysis of indicators related to quality during peach storage. SAT, serine acetyltransferase; OAS-TL, O-acetylserine thiolyase; L-CD, L-cysteine desulfhydrase; NR, nitrate reductase; NOS, nitric oxide synthase-like; PAL, phenylalanine ammonia-lyase; 4CL, 4-coumaric acid coenzyme A ligase; C4H, cinnamic acid-4-hydroxylase
The quality indicators during fruit storage include firmness, soluble solids content, color difference value, relative conductivity, respiration rate, and weight loss rate. Generally, in drupe fruits, especially peach and nectarines, SSC rises due to the loss of water in the pulp during storage. And in the storage process, along with the decomposition of intracellular carbohydrates and the decrease of intracellular metabolite concentration, the fruit firmness gradually decreases, and the fruit becomes soft [52]. In studies on plums [53], mangoes [54], and peach [55], NO inhibits the decrease in firmness, inhibits fruit softening, and maintains storage quality. As in this study, the use of GSNO/β-CD effectively inhibited the increase in SSC and suppressed the decrease in peach firmness. The color difference value reflects the brightness and browning of the pulp in the peach [56]. When the plant is damaged, the cell membrane ruptures, resulting in changes in the liquid environment inside and outside the cytoplasm, thereby increasing the relative conductivity. Therefore, relative conductivity reflects the degree of lipid peroxidation and cell membrane permeability. The upward trend in relative conductivity implies low membrane integrity. However, treatment with 1.0 mmol L−1 GSNO/β-CD reduced the degree of membrane damage to a certain extent. The intensity of respiration is one of the indicators to measure the shelf life of fruits and vegetables [57]. A high respiration rate leads to the high activity of softening enzymes and affects fruit quality [58]. The respiration rate of peach increases after harvest, and inhibiting the respiration rate delays the ripening process and prolongs the storage period of the fruit [59]. This study found that GSNO/β-CD treatment inhibited the increase of color difference value and weight loss rate while reducing the electrical conductivity and respiration rate of peach to prolong the storage time of peach. Among them, the treatment with 1.0 mmol L−1 GSNO/β-CD could achieve the best effect.
Changes in the content of endogenous NO are related to various physiological processes in plant growth and stress response [60]. NR catalyzes the reduction of nitrate and nitrite, which produces endogenous NO. At the same time, NOS-like also generates NO by converting L-arginine to citrulline [61]. When plants are under stress, the activities of NR and NOS increase so that the content of endogenous NO increases to avoid plant damage. Exogenous NO triggers the accumulation of endogenous NO by promoting NOS enzyme activity, thereby maintaining the cold tolerance of bananas [62]. In this experiment, the peach treated with GSNO/β-CD had increased content of endogenous NO. That may be caused by NOS activity and L-arginine content. Studies on melatonin-treated lotus seeds show that increased NOS activity can induce the accumulation of cellular and mitochondrial NO and achieve the effect of inhibiting aging [63]. L-arginine has been shown to inhibit browning in apples, lettuce, and potatoes [64,65]. Therefore, increasing NOS activity, L-arginine, and endogenous NO content also conferred better storage quality of peach. GSNO/β-CD treatment did not significantly increase NR activity, although it increased nitrite content for the first 15 days. It was suggested that the NR pathway in this experiment might not play a significant role in the accumulation of endogenous NO content. Similar findings were also noted by Liao et al. [66] and Huang et al. [46]. Overall, GSNO/β-CD treatment activated the NOS pathway, increased NOS activity and L-arginine content in peach, induced endogenous NO accumulation, and maintained the storage quality of peach.
In plants, serine synthesizes cysteine through the action of SAT and OAS-TL. Then L-CD in the cytoplasm and mitochondria utilizes cysteine to synthesize endogenous H2S [67]. Crosstalk between H2S and NO in fruit ripening has been reported [28]. The combination of H2S and NO inhibits ethylene synthesis and cell wall-modifying enzyme activity better than each treatment alone [68]. The combined application of NO and H2S significantly delays fruit ripening [69]. Promoting the endogenous accumulation of H2S provides cells with sufficient ATP and NADPH to enhance reactive oxygen species (ROS) scavenging activity and maintain the quality of crops during cold storage [70]. This study showed that GSNO/β-CD treatment activated L-CD, OAS-TL, and SAT in peach, thereby promoting endogenous H2S accumulation, which might enhance the activity of the oxidative system and delay the postharvest senescence of peach. Sodium nitroprusside (SNP) treatment induces the accumulation of endogenous H2S in maize seedlings by enhancing the activity of L-CD [71]. NO induces endogenous H2S production in soybean by regulating key enzymatic activities of H2S metabolism [72]. The similarity of these findings further supports the role of GSNO/β-CD treatment in H2S metabolism in peach.
The phenylpropane pathway is vital in plant and fruit disease resistance [73]. PAL, C4H, and 4CL are the first three critical enzymes in the phenylpropane metabolic pathway, catalyzing the conversion of phenylalanine for synthesizing flavonoids, lignin, and other phenolic compounds [74]. The activities of PAL, C4H, and 4CL in peach after GSNO/β-CD treatment were significantly increased during storage, indicating that GSNO/β-CD treatment could trigger the critical enzymes of the phenylpropane metabolic pathway in peach. Phenolics and flavonoids have antibacterial, antiviral, and free radical scavenging activities and play essential roles in plant defenses [75]. Increased enzymatic activity in phenylpropane metabolism promotes the accumulation of total phenolics and flavonoids and enhances resistance to pathogenic bacteria in pears and mangoes [76]. Sodium nitroprusside (SNP), a donor of NO, activates the activity of PAL, C4H, and 4CL and the accumulation of metabolites in apples, which are closely related to the postharvest quality and disease resistance of fruit [74]. The same effect is obtained in SNP-treated blueberries [77]. Similarly, GSNO/β-CD treatment enhanced the activities of key enzymes in the phenylpropane metabolic pathway, such as PAL, 4CL, and C4H, induced the accumulation of antifungal compounds such as phenols, flavonoids, and lignin in the phenylpropane pathway. That might improve the disease resistance of peach and play a role in the interaction between peach and pathogenic bacteria.
The overall results showed that peach treatment with GSNO/β-CD could activate the phenylpropane pathway, increase the accumulation of endogenous NO and regulate the content of endogenous H2S. Fig. 7 showed the possible pathways of GSNO/β-CD regulating NO, H2S, and phenylpropanoid metabolism in peach fruit. It was suggested that 1.0 mmol L−1 GSNO/β-CD has positive effects on improving the disease resistance of postharvest peach and maintaining the postharvest quality of the fruit via regulating the metabolism of endogenous NO, H2S, and phenylpropane. However, the molecular mechanisms by that GSNO/β-CD regulate the metabolism of NO and H2S are still unclear, and further research is also needed to explore the crosstalk among NO, H2S, and phenylpropane, and their roles in maintaining the quality of peach.
Figure 7: Regulation of NO, H2S, and phenylpropanoid metabolism by GSNO/β-CD in peach. SAT, serine acetyltransferase; OAS-TL, O-acetylserine thiolyase; L-CD, L-cysteine desulfhydrase; NR, nitrate reductase; NOS-like, nitric oxide synthase-like; PAL, phenylalanine ammonia-lyase; 4CL, 4-coumaric acid coenzyme A ligase; C4H, cinnamic acid-4-hydroxylase
Authorship: Chen Chen: Investigation, Writing-original draft, Formal analysis. Methodology, Project administration, Validation, Visualization, Writing-review & editing, Software, Data curation. Shuhua Zhu: Funding acquisition, Conceptualization, Supervision, Resources, Writing-review & editing.
Acknowledgement: This work was financially supported by the National Natural Science Foundation of China (32071808).
Funding Statement: This work was supported by the National Natural Science Foundation of China (32071808).
Conflicts of Interest: The authors declare that they have no conflicts of interest to report regarding the present study.
References
1. Liu, S., Jing, G., Zhu, S. (2022). Nitric oxide (NO) involved in antioxidant enzyme gene regulation to delay mitochondrial damage in peach fruit. Postharvest Biology and Technology, 192, 111993. DOI 10.1016/j.postharvbio.2022.111993. [Google Scholar] [CrossRef]
2. Sun, J., You, X., Li, L., Peng, H., Su, W. et al. (2011). Effects of a phospholipase D inhibitor on postharvest enzymatic browning and oxidative stress of litchi fruit. Postharvest Biology and Technology, 62(3), 288–294. DOI 10.1016/j.postharvbio.2011.07.001. [Google Scholar] [CrossRef]
3. Zheng, H., Liu, W., Liu, S., Liu, C., Zheng, L. (2019). Effects of melatonin treatment on the enzymatic browning and nutritional quality of fresh-cut pear fruit. Food Chemistry, 299, 125116. DOI 10.1016/j.foodchem.2019.125116. [Google Scholar] [CrossRef]
4. Piterkova, J., Luhova, L., Hofman, J., Tureckova, V., Novak, O. et al. (2012). Nitric oxide is involved in light-specific responses of tomato during germination under normal and osmotic stress conditions. Annals of Botany, 110(4), 767–776. DOI 10.1093/aob/mcs141. [Google Scholar] [CrossRef]
5. Sami, F., Faizan, M., Faraz, A., Siddiqui, H., Yusuf, M. et al. (2018). Nitric oxide-mediated integrative alterations in plant metabolism to confer abiotic stress tolerance, NO crosstalk with phytohormones and NO-mediated post translational modifications in modulating diverse plant stress. Nitric Oxide, 73(4), 22–38. DOI 10.1016/j.niox.2017.12.005. [Google Scholar] [CrossRef]
6. Sirova, J., Sedlarova, M., Piterkova, J., Luhova, L., Petrivalsky, M. (2011). The role of nitric oxide in the germination of plant seeds and pollen. Plant Science, 181(5), 560–572. DOI 10.1016/j.plantsci.2011.03.014. [Google Scholar] [CrossRef]
7. Deng, Y., Wang, C., Huo, J., Hu, W., Liao, W. (2019). The involvement of NO in ABA-delayed the senescence of cut roses by maintaining water content and antioxidant enzymes activity. Scientia Horticulturae, 247, 35–41. DOI 10.1016/j.scienta.2018.12.006. [Google Scholar] [CrossRef]
8. Adamipour, N., Khosh-Khui, M., Salehi, H., Razi, H., Karami, A. et al. (2020). Role of genes and metabolites involved in polyamines synthesis pathways and nitric oxide synthase in stomatal closure on Rosa damascena Mill. under drought stress. Plant Physiology and Biochemistry, 148(9), 53–61. DOI 10.1016/j.plaphy.2019.12.033. [Google Scholar] [CrossRef]
9. Khan, M. N., Alamri, S., Al-Amri, A. A., Alsubaie, Q. D., Al-Munqedi, B. et al. (2021). Effect of nitric oxide on seed germination and seedling development of tomato under chromium toxicity. Journal of Plant Growth Regulation, 40(6), 2358–2370. DOI 10.1007/s00344-020-10212-2. [Google Scholar] [CrossRef]
10. Zhu, L., Yang, R., Sun, Y., Zhang, F., Du, H. et al. (2021). Nitric oxide maintains postharvest quality of navel orange fruit by reducing postharvest rotting during cold storage and enhancing antioxidant activity. Physiological and Molecular Plant Pathology, 113(4), 101589. DOI 10.1016/j.pmpp.2020.101589. [Google Scholar] [CrossRef]
11. Zhang, Z., Xu, J., Chen, Y., Wei, J., Wu, B. (2019). Nitric oxide treatment maintains postharvest quality of table grapes by mitigation of oxidative damage. Postharvest Biology and Technology, 152, 9–18. DOI 10.1016/j.postharvbio.2019.01.015. [Google Scholar] [CrossRef]
12. Wang, C., Huang, D., Tian, W., Zhu, S. (2021). Nitric oxide alleviates mitochondrial oxidative damage and maintains mitochondrial functions in peach fruit during cold storage. Scientia Horticulturae, 287, 110249. DOI 10.1016/j.scienta.2021.110249. [Google Scholar] [CrossRef]
13. Xu, M., Dong, J., Zhang, M., Xu, X., Sun, L. (2012). Cold-induced endogenous nitric oxide generation plays a role in chilling tolerance of loquat fruit during postharvest storage. Postharvest Biology and Technology, 65, 5–12. DOI 10.1016/j.postharvbio.2011.10.008. [Google Scholar] [CrossRef]
14. Shu, P., Li, Y., Wang, X., Yao, L., Sheng, J. et al. (2021). Exogenous ferulic acid treatment increases resistance against Botrytis cinerea in tomato fruit by regulating nitric oxide signaling pathway. Postharvest Biology and Technology, 182, 111678. DOI 10.1016/j.postharvbio.2021.111678. [Google Scholar] [CrossRef]
15. Zheng, Y., Shen, L., Yu, M., Fan, B., Zhao, D. et al. (2011). Nitric oxide synthase as a postharvest response in pathogen resistance of tomato fruit. Postharvest Biology and Technology, 60(1), 38–46. DOI 10.1016/j.postharvbio.2010.12.003. [Google Scholar] [CrossRef]
16. Wang, X., Cao, J., Qiao, J., Pan, J., Zhang, S. et al. (2022). GABA keeps nitric oxide in balance by regulating GSNOR to enhance disease resistance of harvested tomato against Botrytis cinerea. Food Chemistry, 392(14), 133299. DOI 10.1016/j.foodchem.2022.133299. [Google Scholar] [CrossRef]
17. Ruan, J., Li, M., Jin, H., Sun, L., Zhu, Y. et al. (2015). UV-B irradiation alleviates the deterioration of cold-stored mangoes by enhancing endogenous nitric oxide levels. Food Chemistry, 169, 417–423. DOI 10.1016/j.foodchem.2014.08.014. [Google Scholar] [CrossRef]
18. Luo, Z., Li, D., Du, R., Mou, W. (2015). Hydrogen sulfide alleviates chilling injury of banana fruit by enhanced antioxidant system and proline content. Scientia Horticulturae, 183, 144–151. DOI 10.1016/j.scienta.2014.12.021. [Google Scholar] [CrossRef]
19. Siddiqui, M. W., Deshi, V., Homa, F., Aftab, M. A., Aftab, T. (2021). Inhibitory effects of hydrogen sulfide on oxidative damage and pericarp browning in harvested litchi. Journal of Plant Growth Regulation, 40(6), 2560–2569. DOI 10.1007/s00344-021-10300-x. [Google Scholar] [CrossRef]
20. Li, S. P., Hu, K. D., Hu, L. Y., Li, Y. H., Jiang, A. M. et al. (2014). Hydrogen sulfide alleviates postharvest senescence of broccoli by modulating antioxidant defense and senescence-related gene expression. Journal of Agricultural and Food Chemistry, 62(5), 1119–1129. DOI 10.1021/jf4047122. [Google Scholar] [CrossRef]
21. Sun, Y., Zhang, W., Zeng, T., Nie, Q., Zhang, F. et al. (2015). Hydrogen sulfide inhibits enzymatic browning of fresh-cut lotus root slices by regulating phenolic metabolism. Food Chemistry, 177, 376–381. DOI 10.1016/j.foodchem.2015.01.065. [Google Scholar] [CrossRef]
22. Zhu, L., Wang, W., Shi, J., Zhang, W., Shen, Y. et al. (2014). Hydrogen sulfide extends the postharvest life and enhances antioxidant activity of kiwifruit during storage. Journal of the Science of Food and Agriculture, 94(13), 2699–2704. DOI 10.1002/jsfa.6613. [Google Scholar] [CrossRef]
23. Li, D., Limwachiranon, J., Li, L., Du, R., Luo, Z. (2016). Involvement of energy metabolism to chilling tolerance induced by hydrogen sulfide in cold-stored banana fruit. Food Chemistry, 208, 272–278. DOI 10.1016/j.foodchem.2016.03.113. [Google Scholar] [CrossRef]
24. Liu, D., Xu, S., Hu, H., Pan, J., Li, P. et al. (2017). Endogenous hydrogen sulfide homeostasis is responsible for the alleviation of senescence of postharvest daylily flower via increasing antioxidant capacity and maintained energy status. Journal of Agricultural and Food Chemistry, 65(4), 718–726. DOI 10.1021/acs.jafc.6b04389. [Google Scholar] [CrossRef]
25. Huang, T., Zhang, W., Wang, J., Cai, Z., Shen, Y. et al. (2022). H2S: a new gas with potential biotechnological applications in postharvest fruit and vegetable storage: an overview. Scientia Horticulturae, 300, 111071. DOI 10.1016/j.scienta.2022.111071. [Google Scholar] [CrossRef]
26. Hu, H., Liu, D., Li, P., Shen, W. (2015). Hydrogen sulfide delays leaf yellowing of stored water spinach (Ipomoea aquatica) during dark-induced senescence by delaying chlorophyll breakdown, maintaining energy status and increasing antioxidative capacity. Postharvest Biology and Technology, 108, 8–20. DOI 10.1016/j.postharvbio.2015.05.003. [Google Scholar] [CrossRef]
27. Khan, M. N., Mobin, M., Abbas, Z. K., Siddiqui, M. H. (2017). Nitric oxide-induced synthesis of hydrogen sulfide alleviates osmotic stress in wheat seedlings through sustaining antioxidant enzymes, osmolyte accumulation and cysteine homeostasis. Nitric Oxide, 68, 91–102. DOI 10.1016/j.niox.2017.01.001. [Google Scholar] [CrossRef]
28. Wang, Y., Li, L., Cui, W., Xu, S., Shen, W. et al. (2012). Hydrogen sulfide enhances alfalfa (Medicago sativa) tolerance against salinity during seed germination by nitric oxide pathway. Plant and Soil, 351(1), 107–119. DOI 10.1007/s11104-011-0936-2. [Google Scholar] [CrossRef]
29. Cheng, Y., Lin, Y., Cao, H., Li, Z. (2020). Citrus postharvest green mold: recent advances in fungal pathogenicity and fruit resistance. Microorganisms, 8, 449. DOI 10.3390/microorganisms8030449. [Google Scholar] [CrossRef]
30. Xin, J., Zhao, C., Li, Y., Ma, S., Tian, R. (2022). Transcriptional, secondary metabolic, and antioxidative investigations elucidate the rapid response mechanism of Pontederia cordata to cadmium. Ecotoxicology and Environmental Safety, 232, 113236. DOI 10.1016/j.ecoenv.2022.113236. [Google Scholar] [CrossRef]
31. Zhang, H., Liu, F., Wang, J., Yang, Q., Wang, P. et al. (2021). Salicylic acid inhibits the postharvest decay of goji berry (Lycium barbarum L.) by modulating the antioxidant system and phenylpropanoid metabolites. Postharvest Biology and Technology, 178, 111558. DOI 10.1016/j.postharvbio.2021.111558. [Google Scholar] [CrossRef]
32. Gu, S., Xu, D., Zhou, F., Feng, K., Chen, C. et al. (2022). Repairing ability and mechanism of methyl jasmonate and salicylic acid on mechanically damaged sweet cherries. Scientia Horticulturae, 292, 110567. DOI 10.1016/j.scienta.2021.110567. [Google Scholar] [CrossRef]
33. Liu, Y., Ge, Y., Bi, Y., Li, C., Deng, H. et al. (2014). Effect of postharvest acibenzolar-S-methyl dipping on phenylpropanoid pathway metabolism in muskmelon (Cucumis melo L.) fruits. Scientia Horticulturae, 168, 113–119. DOI 10.1016/j.scienta.2014.01.030. [Google Scholar] [CrossRef]
34. Zhu, Y., Zong, Y., Liang, W., Sabina, A., Chai, X. et al. (2021). β-Aminobutyric acid treatment accelerates the deposition of suberin polyphenolic and lignin at wound sites of potato tubers during healing. Postharvest Biology and Technology, 179, 111566. DOI 10.1016/j.postharvbio.2021.111566. [Google Scholar] [CrossRef]
35. Li, G., Zhu, S., Wu, W., Zhang, C., Peng, Y. et al. (2017). Exogenous nitric oxide induces disease resistance against Monilinia fructicola through activating the phenylpropanoid pathway in peach fruit. Journal of the Science of Food and Agriculture, 97(9), 3030–3038. DOI 10.1002/jsfa.8146. [Google Scholar] [CrossRef]
36. Matamoros, M. A., Cutrona, M. C., Wienkoop, S., Begara-Morales, J. C., Sandal, N. et al. (2020). Altered plant and nodule development and protein S-nitrosylation in Lotus japonicus mutants deficient in S-nitrosoglutathione reductases. Plant and Cell Physiology, 61(1), 105–117. DOI 10.1093/pcp/pcz182. [Google Scholar] [CrossRef]
37. Nasir, N. N. M., Ho, C. L., Lamasudin, D. U., Saidi, N. B. (2020). Nitric oxide improves tolerance to Fusarium oxysporum f. sp. cubense tropical race 4 in banana. Physiological and Molecular Plant Pathology, 111, 101503. DOI 10.1016/j.pmpp.2020.101503. [Google Scholar] [CrossRef]
38. Shishido, S. l. M., Seabra, A. B., Loh, W., Ganzarolli de Oliveira, M. (2003). Thermal and photochemical nitric oxide release from S-nitrosothiols incorporated in Pluronic F127 gel: potential uses for local and controlled nitric oxide release. Biomaterials, 24(20), 3543–3553. DOI 10.1016/S0142-9612(03)00153-4. [Google Scholar] [CrossRef]
39. Zhao, H., Fan, Z., Wu, J., Zhu, S. (2021). Effects of pre-treatment with S-nitrosoglutathione-chitosan nanoparticles on quality and antioxidant systems of fresh-cut apple slices. LWT, 139, 110565. DOI 10.1016/j.lwt.2020.110565. [Google Scholar] [CrossRef]
40. Silveira, N. M., Seabra, A. B., Marcos, F. C. C., Pelegrino, M. T., Machado, E. C. et al. (2019). Encapsulation of S-nitrosoglutathione into chitosan nanoparticles improves drought tolerance of sugarcane plants. Nitric Oxide, 84, 38–44. DOI 10.1016/j.niox.2019.01.004. [Google Scholar] [CrossRef]
41. Jiang, S., Penner, M. H. (2019). The nature of β-cyclodextrin inhibition of potato polyphenol oxidase-catalyzed reactions. Food Chemistry, 298, 125004. DOI 10.1016/j.foodchem.2019.125004. [Google Scholar] [CrossRef]
42. Jia, Z., Luo, Y., Barba, F. J., Wu, Y., Ding, W. et al. (2022). Effect of β-cyclodextrins on the physical properties and anti-staling mechanisms of corn starch gels during storage. Carbohydrate Polymers, 284(9), 119187. DOI 10.1016/j.carbpol.2022.119187. [Google Scholar] [CrossRef]
43. Jin, H., Yang, L., Ahonen, M. J. R., Schoenfisch, M. H. (2018). Nitric oxide-releasing cyclodextrins. Journal of the American Chemical Society, 140(43), 14178–14184. DOI 10.1021/jacs.8b07661. [Google Scholar] [CrossRef]
44. Zhang, L., Zhu, S., Chen, C., Zhou, J. (2011). Metabolism of endogenous nitric oxide during growth and development of apple fruit. Scientia Horticulturae, 127(4), 500–506. DOI 10.1016/j.scienta.2010.11.016. [Google Scholar] [CrossRef]
45. Chen, J., Wu, F., Wang, W., Zheng, C., Lin, G. et al. (2011). Hydrogen sulphide enhances photosynthesis through promoting chloroplast biogenesis, photosynthetic enzyme expression, and thiol redox modification in Spinacia oleracea seedlings. Journal of Experimental Botany, 62(13), 4481–4493. DOI 10.1093/jxb/err145. [Google Scholar] [CrossRef]
46. Huang, D., Wang, Y., Zhang, D., Dong, Y., Meng, Q. et al. (2021). Strigolactone maintains strawberry quality by regulating phenylpropanoid, NO, and H2S metabolism during storage. Postharvest Biology and Technology, 178, 111546. DOI 10.1016/j.postharvbio.2021.111546. [Google Scholar] [CrossRef]
47. Aghdam, M. S., Fard, J. R. (2017). Melatonin treatment attenuates postharvest decay and maintains nutritional quality of strawberry fruits (Fragaria × anannasa cv. Selva) by enhancing GABA shunt activity. Food Chemistry, 221, 1650–1657. DOI 10.1016/j.foodchem.2016.10.123. [Google Scholar] [CrossRef]
48. Gao, H., Zhang, Z. K., Chai, H. K., Cheng, N., Yang, Y. et al. (2016). Melatonin treatment delays postharvest senescence and regulates reactive oxygen species metabolism in peach fruit. Postharvest Biology and Technology, 118, 103–110. DOI 10.1016/j.postharvbio.2016.03.006. [Google Scholar] [CrossRef]
49. Zargoosh, Z., Ghavam, M., Bacchetta, G., Tavili, A. (2019). Effects of ecological factors on the antioxidant potential and total phenol content of Scrophularia striata Boiss. Scientific Reports, 9(1), 16021. DOI 10.1038/s41598-019-52605-8. [Google Scholar] [CrossRef]
50. Angmo, P., Chorol, S., Namgail, D., Chaurasia, O. P., Stobdan, T. (2019). Effect of maturation on phenolics and flavonoids content of greenhouse-grown beet leaf. Pharmacognosy Journal, 11(5), 1010–1013. DOI 10.5530/pj.2019.11.159. [Google Scholar] [CrossRef]
51. Zhang, L., Kamitakahara, H., Sasaki, R., Oikawa, A., Saito, K. et al. (2020). Effect of exogenous GA4+7 and BA+CPPU treatments on fruit lignin and primary metabolites in Japanese pear “Gold Nijisseiki”. Scientia Horticulturae, 272, 109593. DOI 10.1016/j.scienta.2020.109593. [Google Scholar] [CrossRef]
52. Zhang, C., Xiong, Z., Yang, H., Wu, W. (2019). Changes in pericarp morphology, physiology and cell wall composition account for flesh firmness during the ripening of blackberry (Rubus spp.) fruit. Scientia Horticulturae, 250, 59–68. DOI 10.1016/j.scienta.2019.02.015. [Google Scholar] [CrossRef]
53. Singh, S. P., Singh, Z., Swinny, E. E. (2009). Postharvest nitric oxide fumigation delays fruit ripening and alleviates chilling injury during cold storage of Japanese plums (Prunus salicina Lindell). Postharvest Biology and Technology, 53(3), 101–108. DOI 10.1016/j.postharvbio.2009.04.007. [Google Scholar] [CrossRef]
54. Ren, Y., He, J., Liu, H., Liu, G., Ren, X. (2017). Nitric oxide alleviates deterioration and preserves antioxidant properties in ‘Tainong’ mango fruit during ripening. Horticulture, Environment, and Biotechnology, 58(1), 27–37. DOI 10.1007/s13580-017-0001-z. [Google Scholar] [CrossRef]
55. Huang, D., Hu, S., Zhu, S., Feng, J. (2019). Regulation by nitric oxide on mitochondrial permeability transition of peaches during storage. Plant Physiology and Biochemistry, 138, 17–25. DOI 10.1016/j.plaphy.2019.02.020. [Google Scholar] [CrossRef]
56. Wantat, A., Seraypheap, K., Rojsitthisak, P. (2022). Effect of chitosan coatings supplemented with chitosan-montmorillonite nanocomposites on postharvest quality of ‘Hom Thong’ banana fruit. Food Chemistry, 374, 131731. DOI 10.1016/j.foodchem.2021.131731. [Google Scholar] [CrossRef]
57. Ma, Y., Zhang, W., Cheng, S., Liu, Y., Yang, W. et al. (2022). Postharvest storage at near-freezing temperature maintained the quality and antioxidant properties of Prunus domestica L. cv. Ximei fruit. Scientia Horticulturae, 293, 110720. DOI 10.1016/j.scienta.2021.110720. [Google Scholar] [CrossRef]
58. Chen, Y., Ge, Y., Zhao, J., Wei, M., Li, C. et al. (2019). Postharvest sodium nitroprusside treatment maintains storage quality of apple fruit by regulating sucrose metabolism. Postharvest Biology and Technology, 154, 115–120. DOI 10.1016/j.postharvbio.2019.04.024. [Google Scholar] [CrossRef]
59. Pan, Y., Li, X., Jia, X., Zhao, Y., Li, H. et al. (2019). Storage temperature without fluctuation enhances shelf-life and improves postharvest quality of peach. Journal of Food Processing and Preservation, 43(3), e13881. DOI 10.1111/jfpp.13881. [Google Scholar] [CrossRef]
60. Adhikary, T., Gill, P. P. S., Jawandha, S. K., Bhardwaj, R. D., Anurag, R. K. (2021). Efficacy of postharvest sodium nitroprusside application to extend storability by regulating physico-chemical quality of pear fruit. Food Chemistry, 346, 128934. DOI 10.1016/j.foodchem.2020.128934. [Google Scholar] [CrossRef]
61. Gupta, P., Seth, C. S. (2020). Interactive role of exogenous 24 epibrassinolide and endogenous NO in Brassica junceaL. under salinity stress: Evidence for NR-dependent NO biosynthesis. Nitric Oxide, 97, 33–47. DOI 10.1016/j.niox.2020.01.014. [Google Scholar] [CrossRef]
62. Wang, Y., Luo, Z., Mao, L., Ying, T. (2016). Contribution of polyamines metabolism and GABA shunt to chilling tolerance induced by nitric oxide in cold-stored banana fruit. Food Chemistry, 197, 333–339. DOI 10.1016/j.foodchem.2015.10.118. [Google Scholar] [CrossRef]
63. Sun, L., Luo, S., Huali, H., Zhou, H., Zhang, Y. et al. (2022). Melatonin promotes the normal cellular mitochondrial function of lotus seeds through stimulating nitric oxide production. Postharvest Biology and Technology, 185, 111814. DOI 10.1016/j.postharvbio.2021.111814. [Google Scholar] [CrossRef]
64. Wills, R. B. H., Li, Y. (2016). Use of arginine to inhibit browning on fresh cut apple and lettuce. Postharvest Biology and Technology, 113, 66–68. DOI 10.1016/j.postharvbio.2015.11.006. [Google Scholar] [CrossRef]
65. Ali, H. M., El-Gizawy, A. M., El-Bassiouny, R. E. I., Saleh, M. A. (2016). The role of various amino acids in enzymatic browning process in potato tubers, and identifying the browning products. Food Chemistry, 192, 879–885. DOI 10.1016/j.foodchem.2015.07.100. [Google Scholar] [CrossRef]
66. Liao, W., Zhang, M., Yu, J. (2013). Role of nitric oxide in delaying senescence of cut rose flowers and its interaction with ethylene. Scientia Horticulturae, 155, 30–38. DOI 10.1016/j.scienta.2013.03.005. [Google Scholar] [CrossRef]
67. Li, Z. G. (2015). Analysis of some enzymes activities of hydrogen sulfide metabolism in plants. Methods Enzymol, 555, 253–269. DOI 10.1016/bs.mie.2014.11.035. [Google Scholar] [CrossRef]
68. Zhu, L., Du, H., Wang, W., Zhang, W., Shen, Y. et al. (2019). Synergistic effect of nitric oxide with hydrogen sulfide on inhibition of ripening and softening of peach fruits during storage. Scientia Horticulturae, 256(2), 108591. DOI 10.1016/j.scienta.2019.108591. [Google Scholar] [CrossRef]
69. Gong, T., Li, C., Bian, B., Wu, Y., Dawuda, M. M. et al. (2018). Advances in application of small molecule compounds for extending the shelf life of perishable horticultural products: A review. Scientia Horticulturae, 230, 25–34. DOI 10.1016/j.scienta.2017.11.013. [Google Scholar] [CrossRef]
70. Niazi, Z., Razavi, F., Khademi, O., Aghdam, M. S. (2021). Exogenous application of hydrogen sulfide and γ-aminobutyric acid alleviates chilling injury and preserves quality of persimmon fruit (Diospyros kaki,cv. Karaj) during cold storage. Scientia Horticulturae, 285, 110198. DOI 10.1016/j.scienta.2021.110198. [Google Scholar] [CrossRef]
71. Li, Z., Yang, S., Long, W., Yang, G., Shen, Z. (2013). Hydrogen sulphide may be a novel downstream signal molecule in nitric oxide-induced heat tolerance of maize (Zea maysL.) seedlings. Plant, Cell & Environment, 36(8), 1564–1572. DOI 10.1111/pce.12092. [Google Scholar] [CrossRef]
72. Wang, H., Ji, F., Zhang, Y., Hou, J., Liu, W. et al. (2019). Interactions between hydrogen sulphide and nitric oxide regulate two soybean citrate transporters during the alleviation of aluminium toxicity. Plant, Cell & Environment, 42(8), 2340–2356. DOI 10.1111/pce.13555. [Google Scholar] [CrossRef]
73. Zhang, W., Jiang, H., Cao, J., Jiang, W. (2021). UV-C treatment controls brown rot in postharvest nectarine by regulating ROS metabolism and anthocyanin synthesis. Postharvest Biology and Technology, 180, 111613. DOI 10.1016/j.postharvbio.2021.111613. [Google Scholar] [CrossRef]
74. Ge, Y., Chen, Y., Li, C., Zhao, J., Wei, M. et al. (2019). Effect of sodium nitroprusside treatment on shikimate and phenylpropanoid pathways of apple fruit. Food Chemistry, 290, 263–269. DOI 10.1016/j.foodchem.2019.04.010. [Google Scholar] [CrossRef]
75. Cetin-Karaca, H., Newman, M. C. (2015). Antimicrobial efficacy of plant phenolic compounds against Salmonella and Escherichia coli. Food Bioscience, 11, 8–16. DOI 10.1016/j.fbio.2015.03.002. [Google Scholar] [CrossRef]
76. Yin, Y., Bi, Y., Li, Y., Wang, Y., Wang, D. (2012). Use of thiamine for controlling Alternaria alternata postharvest rot in Asian pear (Pyrus bretschneideriRehd. cv. Zaosu). International Journal of Food Science & Technology, 47(10), 2190–2197. DOI 10.1111/j.1365-2621.2012.03088.x. [Google Scholar]
77. Ge, Y., Li, X., Li, C., Tang, Q., Duan, B. et al. (2019). Effect of sodium nitroprusside on antioxidative enzymes and the phenylpropanoid pathway in blueberry fruit. Food Chemistry, 295, 607–612. DOI 10.1016/j.foodchem.2019.05.160. [Google Scholar] [CrossRef]
Cite This Article
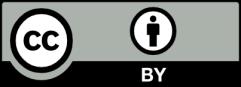
This work is licensed under a Creative Commons Attribution 4.0 International License , which permits unrestricted use, distribution, and reproduction in any medium, provided the original work is properly cited.