Open Access
ARTICLE
The Potential Efficacy of Glycyrrhizic Acid and Its Nanostructure Against Brown Rot of Peach fruits
1
Environmental Biotechnology Department, College of Biotechnology, Misr University for Science and Technology, 6th of October
City, Egypt
2
The Regional Center for Mycology and Biotechnology (RCMB), Al-Azhar University, Cairo, 11435, Egypt
3
Plant Pathology Research Institute, Agricultural Research Center, Giza, 12619, Egypt
* Corresponding Author: Khamis Youssef. Email:
(This article belongs to the Special Issue: Modern Strategies for Controlling Plant Diseases)
Phyton-International Journal of Experimental Botany 2023, 92(4), 1139-1152. https://doi.org/10.32604/phyton.2023.026515
Received 09 September 2022; Accepted 20 October 2022; Issue published 06 January 2023
Abstract
Production of peaches (Prunus persica (L.) Batsch) for both local market and export is increasing each year in Egypt. Brown rot disease, caused by Monilinia laxa and Monilinia fructigena, is considered one of the most important postharvest rots affecting peaches in Egypt and economic losses are increasing. Antifungal activity of glycyrrhizic acid nanoparticles (GA-NPs) and glycyrrhizic acid (GA) at 0.2 and 0.4 mmol/L was investigated as a control for both these brown rot pathogens on peach fruits in both in vitro and in vivo studies. In the in vitro studies, GA-NPs were the most effective as shown by the ability to decrease linear growth of both brown rot pathogens in potato dextrose agar (PDA) amended with 0.4 mmol/L GA-NPs. Micrographs of M. fructigena exposed to 0.4 mmol/LGA showed mycelial deformations, nodule formation, detachment of the cell wall, shrinkage and inhomogeneous cytoplasmic materials with large vacuoles. Mycelium of M. laxa exposed to 0.4 mmol/ LGA-NPs resulted in thinner and distorted hyphae, nodule formation, cell wall thinning, and swellings. The GANPs and GA treatments improved fruit quality by maintaining firmness and total soluble solids (TSS). GA-NPs were more effective in decreasing decay incidence than their bulk material. The 0.4 mmol/L GA-NPs completely inhibited the disease on naturally infected peach fruits for both seasons of 2018 and 2019. Furthermore, 0.4 mmol/L GA-NPs reduced the disease incidence in inoculated fruits by 95 (M. laxa) and 88% (M. fructigena) in 2018 season and 96 (M. laxa) and 85% (M. fructigena) in 2019 season. In conclusion, GA-NPs could enhance the resistance of peaches against brown rot caused by M. laxa and M. fructigena.Keywords
Production of peaches (Prunus persica L. Batsch) for both the local market and export is increasing each year in Egypt. In the last decade, peach production has spread widely in the recently reclaimed regions of Egypt [1]. In Egypt, the peach area under production is estimated to be 16,226 ha with a harvest of 337,910 tonnes [2]. Peach fruits are enriched with phenolic compounds, ascorbic acid, and carotenoids (pro-vitamin A), and are considered a major source of antioxidants [3,4]. Recently, the production and marketing of peach fruits have increased rapidly in Egypt due to their enriched nutrients and pleasant flavor.
Peach fruits are infected by several fungi during the postharvest and storage stages. Brown rot disease, caused by pathogenic fungi Monilinia laxa and M. fructigena, is thought to be the most serious postharvest rot affecting peaches and economic losses are increasing. The disease begins in the fields, but symptoms commonly appear at the fruit maturity [5]. The pathogenic fungi penetrate fruit mainly through wounds, incited by insects in the field or during harvest and postharvest handling [6]. M. laxa and M. fructigena conidia can germinate at low temperatures as low as −4°C, while mycelia can grow at 0°C [7,8].
In traditional and integrated production systems, brown rot disease is managed by protective applications of fungicides during blooming and preharvest phases. Postharvest fungicide applications are only allowed in a few countries and restrictions are increasing [6]. This reality has catalysed research in Egypt on alternative means of brown rot control on peach fruits during the storage as well as research on improved fruit quality. Several alternative control methods for various postharvest diseases of fruits and vegetables have been proposed [9–11]. Postharvest rots of peach fruits have been effectively controlled using different treatments such as hot water dipping [12], hexanal [13], biocontrol agents, modified atmosphere [14], sodium bicarbonate, calcium chloride, potassium sorbate, boric acid [15] and citric acid [16]. Such products are associated with plant defense mechanisms and could be extracted and applied to other harvested perishables such as fruit and vegetables.
Recently, nanomaterial treatments have shown favourable results in the field of plant disease management [17]. Natural nanomaterials have emerged as a key solution for limiting the use of synthetic fungicides for postharvest rots in fruits and vegetables [18]. Postharvest treatment with nano-chitosan at 0.4% has been shown to decrease the percentage of peach fruit decay, total soluble solids (TTS)/acid ratio and maintained fruit pulp firmness [19]. In storage, chitosan–rice starch nanocomposite films have been shown to maintain peach quality [20]. Antimicrobial activities of formulated nanocomposite showed bacteriostatic properties against peach microorganisms [20].
Glycyrrhizinic acid (GA) was designated as a Generally Recognised as Safe (GRAS) substance in the United States in 1985 [21]. GA is extracted from the root of the licorice plant (Glycyrrhiza glabra). It is a triterpene glycoside with glycyrrhetinic acid that possesses a wide range of pharmacological and biological activities [22]. When extracted from the plant, it can be obtained as both ammonium glycyrrhizin and mono-ammonium glycyrrhizin forms [23]. GA has been used in Japan and China as a hepatoprotective drug in cases of chronic hepatitis [24].
The present study aimed to (i) investigate the effect of bulk materials and the nano-form of GA against M. laxa and M. fructigena in vitro and in vivo on peach fruit during storage; and (ii) to evaluate their effect on peach fruit quality parameters as firmness and total soluble solids content.
The strains of M. laxa (NO.154) and M. fructigena (NO.256) were previously isolated from naturally infected peach fruits showing brown rot symptoms [25] and tested for their pathogenicity at the Postharvest Diseases Dept., Plant Pathol. Res. Inst., ARC, Giza, Egypt. The isolates were maintained on potato dextrose agar (PDA) slants at 5 ± 1°C, with annual inoculation and re-isolation from fruits to maintain their virulence. For the present investigation, the strains were grown on PDA medium at 25 ± 2°C for 7 days for in vitro studies and for artificial inoculation of peach fruits in disease control experiments.
GA was purchased from Sigma-Aldrich (CAS Number: 1405-86-3), 0.2 mg of GA was dissolved in 1 mL of absolute ethanol and sonicated (XUBA3Analogue Ultrasonic Bath Range, Grant Instruments, Cambridge, UK) with an ultrasonic power and frequency of 44 KHz for 1 h at room temperature (25°C) [26].
2.3 Characterization of Nano-GA
Distribution and size of nanoparticles of GA (GA-NPs) were performed by a dynamic light scattering (DLS) method using Zetasizer Nano ZS (Malvern Instruments, UK) at room temperature. Before measurement, 30 μL of GA-NPs were diluted with 3 mL of water at 25°C. Particle size data was expressed as the mean of the Z-average of three independent batches of the nanoparticles [27]. The dispersibility of GA-NPs was determined using a transmission electron microscope (TEM) (FEI Tecnai G2, FEI Company, Hillsboro, OR, USA). Different forms of imaging were engaged: bright field at an electron accelerating voltage of 200 kV using a lanthanum hexaboride (LaB6) electron source gun, and diffraction example imaging. An Eagle CCD camera with (4 k/4 k) picture resolution was utilized for transmitted electron pictures [28].
2.4 The Antifungal Activity of GA-NPs and Their Bulk Materials on Linear Growth of the Two Tested Fungi in Vitro
The antifungal activity of synthesised GA-NPs and their bulk material (GA) was tested at two concentrations (0.2 and 0.4 mmol/L) on linear growth on PDA of the two Monilinia species. Individually tested concentrations of GA-NPs and GA were added to the melted PDA and gently blended to evenly mix all components PDA with no added compounds was used as a control. The treated medium was poured into sterile Petri dishes (9 cm in diameter) with approximately 10 mL of medium per dish (three dishes for each fungus). Once solid, all dishes were centrally inoculated with a 5 mm mycelial disc of each tested fungus. Each treatment had three replicates, and the whole experiment was repeated twice. Inoculated dishes were incubated at 20 ± 2°C for 7 days. The percentage of growth inhibition was calculated according to the following [29]:
where R is the radial growth of the fungal mycelia on the control plate and r is the radial growth of the fungal mycelia on the plate containing various concentrations of GA-NPs or GA.
2.5 Scanning Electron Microscopy (SEM)
The effect of 0.4 mmol/L GA-NPs and GA on hyphal morphological features and reproductive structures (conidia) of M. laxa and M. fructigena was investigated using Scanning electron microscopy (SEM, Model: JSM-5500 LV; JEOL, Ltd., Japan) following a reference methodology [30]. Tissue samples of the 4 day-old colonies on PDA two pathogens were cutted, fixed by immersion in 2.5% (v/v) glutaraldehyde in 0.1 mol/L sodium cacodylate buffer pH 7.0 for 1 h, washed three times in 0.1 mol/L sodium cacodylate buffer pH 7.0, post-fixed with 1% (m/v) osmium tetroxide in the same buffer for 1 h and washed three times in 0.1 mol/L sodium cacodylate buffer pH 7.0. The material was then dehydrated in a crescent acetone series (10%, 25%, 40%, 60%, 75%, 85%, 95% and 100%, v/v) with 15 min per change. The specimens were transferred to a critical point dryer to complete the drying process with carbon dioxide as a transition fluid. Then, the specimens obtained were mounted on aluminums tubs, with double-stick carbon tape and sputter-coated with gold and observed using a field emission scanning electron microscope in high vacuum mode at the Regional Center of Mycology and Biotechnology, Cairo, Egypt.
2.6 Transmission Electron Microscopy (TEM)
For TEM preparation, the tissue samples of the colonies of the two Monilinia species on PDA medium containing GA-NPs and GA at 0.4 mmol/L were fixed in 3% glutaraldehyde, rinsed in phosphate buffer, and post-fixed in potassium permanganate solution for 5 min at room temperature. The samples were dehydrated in an ethanol series ranging from 10% to 90% for 15 min in each alcohol dilution and finally with absolute ethanol for 30 min. Samples were infiltrated with epoxy resin and acetone through a graded series of tests until in pure resin. Ultrathin sections were cut and collected on copper grids. Sections were then double stained in uranyl acetate, followed by lead citrate. Stained sections were observed with a JEOL-JEM 1010 (Tokyo, Japan) transmission electron microscope at 70 kV at The Regional Center for Mycology and Biotechnology (RCMB), Al Azhar University [31,32].
2.7 Impact of Postharvest Treatments of GA-NPs and Their Bulk Materials on Fruit Rot Incidence and Quality of Peach Fruits during Storage
GA-NPs and their bulk materials each at 0.2 and 0.4 mmol/L were tested for controlling brown rot caused by the two Monilinia species in natural and artificially infected fruit. Peach fruits cv. Desert Red were harvested at maturity stage from an orchard in Ismailia Governorate, Egypt in the last week of April 2018 and 2019. Fruits were selected for uniform size, color, and absence of mechanical damage. Peach fruits were divided into three groups. The first group classified as natural infection was used without surface disinfection. The other two groups of peach fruits were washed thoroughly with tap water, surface-sterilized by dipping in 2% sodium hypochlorite solution (v/v) for 2 min, washed with distilled water then left to dry at sterilized room temperature. Fruits were punctured using a stainless-steel rod (diameter 1 mm × 2 mm) at the opposite sides of the fruit. One group of the punctured fruit was inoculated with M. laxa (isolate NO.154), and the last group was inoculated with M. fructigena (isolate NO.256). Inoculation was by immersion of the fruits in a conidial suspension of 106 conidia/ml for 15 s. The conidial suspension was prepared following the methods described previously [33]. After 24 h of incubation, the naturally infected and artificially inoculated fruits were dipped separately in 0.2 and 0.4 mmol/L of the proposed compound solutions for 2 min, while the control treatment was dipped in sterile distilled water and left to dry at room temperature. The treated fruits were packed in polypropylene baskets (1 kg). Three replicates were used for each treatment. Each replicate consisted of three baskets (containing 13 peach fruits each). Naturally infected and artificially inoculated peach fruits were stored at 0–1°C with 90% RH for 35 days. After the cold storage period, brown rot incidence (%), fruit firmness, and TSS were determined.
2.8 Disease Incidence Assessment
The percent disease incidence of infected fruits was calculated using the following formula [16] as follows:
2.9 Assay of Fruit Quality Parameters
The fruit firmness was measured on the two opposite sides of peach fruit samples by using a hand Magness Taylor pressure tester (lb/in2) (Model FT 327, Italy). Total soluble solid (TSS) content was determined using a hand refractometer (PAL 1, Atago Co., Ltd., Tokyo, Japan) and the results were expressed as Brix° [34].
The experiments were designed as completely randomised designs and were repeated twice to confirm the observations. Statistica Software Ver. 6.0 (Stat Soft, Inc., Tulsa, OK, USA) was used to perform a one-way ANOVA and differences between means were compared by Duncan’s Multiple Range Test at P < 0.05.
3.1 Characterization of GA-NPs
The size distribution of the GA-NPs primarily ranged between 18–50 nm as shown in Fig. 1. The zeta potential is a key indicator of the stability of colloidal dispersions. Hence, colloids with high zeta potential (positive) were electrically stabilised (Fig. 2). TEM analysis showed that the GA-NPs were widely dispersed and composed of particles with an average size of <100 nm. The TEM micrograph showed that GA-NPs had circular morphology with an average size between 22.6 and 48.3 nm (Fig. 3).
Figure 1: Z average size and size distribution of glycyrrhizic acid nanoparticles using dynamic light scattering
Figure 2: Zeta potential distribution of glycyrrhizic acid nanoparticles using dynamic light scattering
Figure 3: Transmission electron microscope micrograph of glycyrrhizic acid nanoparticles with circular morphology
3.2 The Antifungal Activity of GA-NPs and Their Bulk Materials on Linear Growth of the Two Tested Fungi in Vitro
GA-NPs and their bulk materials each at 0.2 and 0.4 mmol/L reduced the linear growth of M. laxa and M. fructigena compared to the untreated control (Table 1). GA-NPs were the most effective treatment providing the largest decrease (P < 0.05) in linear growth of both Monlinia species when tested at 0.4 mmol/L. GA-NPs at 0.2 mmol/L did reduce (P < 0.05) linear growth but was less effective than the 0.4 mmol/L. Overall, the mycelial growth of M. laxa was slightly more sensitive to the tested compounds than M. fructigena.
3.3 Scanning Electron Microscopy
Results for M. fructigena after 4 days of growth at 20°C demonstrated that 0.4 mmol/L GA-NPs were more effective in altering the morphology of mycelia than they were in their bulk state, which induced deformation, dehydration, and collapsing hyphae (Fig. 4C). As a result of exposure to 0.4 mmol/LGA, mycelial deformations, nodule development, and cell wall detachment were visible in micrographs of M. fructigena-mycelium (Fig. 4B). The mycelia from the control treatment (Fig. 4A) were undamaged.
Figure 4: Scanning electron micrographs of M. fructigena mycelia after 4 days incubation period at 20 ± 2°C. (A) Control (untreated), (B) medium amended with GA (0.4 mmol/L) and (C) medium amended with GA-NPs (0.4 mmol/L)
In the control treatment (Fig. 5A), mycelium appeared normal and without deformations. M. laxa exposed to 0.4 mmol/L GA resulted in abnormal hyphal shapes and swellings (Fig. 5B). In addition, mycelium of M. laxa exposed to 0.4 mmol/L GA-NPs resulted in thinner and distorted hyphae, nodule formation, cell wall thinning (Fig. 5C). No damage to morphology was observed to the conidia of M. laxa in the control treatment (Fig. 5A). Conidia of M. laxa exposed to 0.4 mmol/L GA (Fig. 5B) and GA-NPs (Fig. 5C) showed deformations, dehydration and collapsed cell walls.
Figure 5: Scanning electron micrographs of M. laxa mycelia after 4 days incubation period at 20 ± 2°C. (A) control (untreated), (B) medium amended with GA (0.4 mmol/L) and (C) medium amended with GA-NPs (0.4 mmol/L)
3.4 Transmission Electron Microscopy
Typical fungal ultrastructure was visible in ultrathin slices of untreated M. fructigena, including normal cell wall thickness (CW), regular and intact cell membrane (CM), regularly shaped mitochondria (M), nuclei (N) and nucleoli (Nu), and homogenous cellular cytoplasm in the mycelia (Fig. 6A). An ultrathin segment of M. fructigena treated with 0.4 mmol/L GA when examined after 4 days showed shrinkage and inhomogeneous cytoplasmic materials with large vacuoles (Fig. 6B). Finally, M. fructigena cultivated treated with 0.4 mmol/L GA-NPs displayed considerable, dramatic alterations of cell wall/membrane, mitochondria, and nucleus (Fig. 6C).
Figure 6: TEM micrograph of M. fructigena (A) control, (B) treated with glycyrrhizic acid bulk at 0.4 mmol/L, and (C) treated with glycyrrhizic acid nanoparticles at 0.4 mmol/L. CW: Cell Wall, CM: Cell membrane, M: Mitochondria, N: Nucleus, and Nu: Nucleolus. Scale bar = 500 nm
The TEM findings revealed small changes in the ultrastructure of M. laxa mycelial cells exposed to 0.4 mmol/L GA for 4 days, such as partial cell wall removal and irregular distribution of cytoplasmic organelles (Fig. 7B). The M. laxa mycelial ultrastructure treated with 0.4 mmol/L GA-NPs demonstrated changes to the hyphal cell structures, including decreased thickness in both the cell wall and cell membrane and cytoplasmic component deformations (Fig. 7C). Untreated M. laxa-mycelial ultrastructure (Fig. 7A) showed no change to normal ultrastructure.
Figure 7: TEM micrographof M. laxa (A) control, (B) treated with glycyrrhizic acid bulk at 0.4 mmol/L, and (C) treated with glycyrrhizic acid nanoparticles at 0.4 mmol/L. CW: Cell Wall, CM: Cell membrane, M: Mitochondria, V: Vacuole N: Nucleus and Nu: Nucleolus. Scale bar = 500 nm
3.5 Effect of Treatments on Postharvest Decay Development of Peach Fruits in Vivo
GA-NPs and their bulk materials were applied to control brown rot caused by M. laxa and M. fructigena in peach fruits stored at 0°C–1°C and 90% RH for 35 days. The effect of different treatments on the reduction of disease incidence was shown in Tables 2 and 3. When compared to controls, all treatments demonstrated that peach fruit were better protected from M. laxa and M. fructigena infections. Data obtained indicated that over the course of two seasons, GA-NPs were more effective than their bulk material at reducing fruit decay brown rot infection.
Under artificial infection, 0.4 mmol/LGA-NPs reduced the disease incidence by 95 (M. laxa) and 88% (M. fructigena) in 2018 season, and 96 and 85% in 2019 season. Whereas, 0.2 mmol/L GA-NPs reduced the disease incidence by 77 (M. laxa) and 70% (M. fructigena) in 2018 season, and 78 and 68% in 2019 season. Results also showed that 0.4 mmol/L GA reduced the infection by 76 (M. laxa) and 67% (M. fructigena) in 2018 season and 72 and 65% in 2019 season. In addition, 0.2 mmol/LGA reduced the infection by 61 (M. laxa) and 52% (M. fructigena) in 2018 season and 59 and 50% in 2019 season.
Under natural infection, 0.4 mmol/L GA-NPs completely inhibited the disease for both 2018 and 2019 seasons. In the second rank, 0.2 mmol/L GA-NPs reduced the infection by 75 and 63.5% during the seasons 2018 and 2019, respectively. Results also showed that 0.4 mmol/L GA reduced the infection by 76 and 73% during the seasons 2018 and 2019, respectively. In addition, 0.2 mmol/L GA reduced the disease incidence by 50 and 44% during the seasons 2018 and 2019, respectively.
3.6 Effect of Postharvest Treatment with GA-NPs and Their Bulk Materials on Firmness and Total Soluble Solids of Peach Fruits during Cold Storage
The efficacy of postharvest treatments of GA-NPs and their natural forms on firmness (lb/in2) of peach fruit cv. Desert Red was shown in Table 4. In most cases, the firmness of peach fruits treated with GA-NPs and their natural forms was reduced after cold storage compared to at harvest time with some exceptions. During the cold storage, under natural infection, the tested treatments significantly increased the firmness as compared to the untreated control. The firmness ranged between 5.2–6.9 and 4.8–6.8 for 2018 and 2019 seasons, respectively. Firmness was high in the natural infection when peach fruits were treated with 0.2 mmol/L GA-NPs. Under natural infected fruits, the firmness was 2.2 and 3.2 for 2018 and 2019 seasons, respectively.
Under artificial inoculation, the bulk material of GA at 0.4 mmol/L was the most effective treatment to maintain the highest firmness of peach fruits during the cold storage in both seasons of 2018 and 2019. The firmness was 7.2 and 12.2 for fruits treated with GA and inoculated with M. laxa and M. fructigena during 2018, respectively. It was also 6.7 and 12 for fruits treated with GA and inoculated with M. laxa and M. fructigena during 2019, respectively.
The efficacy of postharvest treatments of GA-NPs and their bulk materials on total soluble solids (%) of peach fruit cv. Desert Red was shown in Table 5. Under natural infection, the total soluble solids (TSS) content was higher in the fruits after storage as compared to the initial TSS. In most cases, no significant differences were noted among all treatments. 0.2 mmol/LGA-NPs showed the highest TSS of naturally infected peach fruits compared to other treatments. On the other hand, TSS was enhanced after the cold storage for all treatments, including the control treatment.
The main objectives of the present study were to investigate the effects of bulk and nano form of glycyrrhizic acid treatments against M. laxa and M. fructigena in vitro and in vivo on peach fruit decay incidence during storage and to evaluate their effects on fruit firmness and percent total soluble solids. The in vitro studies showed that GA-NPs were more effective than bulk forms: they greatly decreased the linear growth of the two brown rot pathogens at 0.4 mmol/L. Several researchers have used nanomaterials in vitro against several pathogenic fungi. Some nanoparticles reduced the fungal growth of Botrytis cinerea compared with control [35]. In particular, in vitro tests showed that chitosan nanoparticles, silica nanoparticles and chitosan-silica nanocomposites reduced fungal growth by 72%, 76% and 100%, respectively at 1% as compared to control.
Also, nanosized silica silver at different concentrations (0.3–100 ppm) was evaluated in vitro against several plant pathogenic fungi including Pythium ultimum, Magnaporthe grisea, Colletotrichum gloeosporioides, Botrytis cinerea, and Rhizoctonia solani. The compound showed antifungal activity against the tested phytopathogenic fungi at 3.0 ppm with varied levels. In the field and greenhouse experiments, the compound effectively controlled powdery mildews of pumpkin at 0.3 ppm [36]. Recently, studies showed that chitosan nanoparticles significantly reduced the linear growth of many pathogenic fungi, including Rhizopus sp., Colletotrichum capsici, Colletotrichum gloeosporioides, Aspergillus niger, and Fusarium solani [37,38].
Our results of SEM analysis agree with other researchers. Similar observations have been reported [39] when testing the efficacy in inhibiting the growth of Penicillium verrucosum with small-sized nanoparticles. This strongly supports our hypothesis that the small particles penetrate the fungal cell and disturb important cellular mechanisms. Also, scanning electron microscopy (SEM) showed severe damage in the hyphae of Alternaria alternata and Penicillium digitatum by causing deformities in the conidia due to the effect of glycyrrhizic acid nanoparticles [26].
Transmission Electron Microscope observations [40] found that the cell wall and cell membrane structure of Trichosporon asahii was severely damaged by silver nanoparticles. Treatment with silver nanoparticles resulted in dead cells, as suggested by the disappearance of cell walls and cell membranes. There was degeneration and damage of organelles in silver-treated cells, including margination and condensation of chromatin. Also, Alternaria alternata treated with salicylic acid nanoparticles and their natural forms greatly decreased the cell wall thickness as well as causing shrinkage of the cytoplasm [41].
In vivo results obtained herein showed that GA-NPs were more effective in decreasing decay infection than their bulk material during two seasons. The approach of disease control using nanoparticles was also adopted [20] to demonstrate that chitosan–rice starch nanocomposite films showed high efficacy against peach microorganisms. Treatment with zeolite nanoparticles coated with potassium permanganate reduced the contamination of peach fruits during storage [42]. Also, chitosan NPs at 0.4% gave the lowest percentage of fruit decay [19]. Meanwhile, glycyrrhizic acid nanoparticles greatly reduced the disease severity of infected tomato fruits with Alternaria alternata and Penicillium digitatum [26].
Concerning fruit quality, chitosan–rice starch nanocomposite films were able to maintain peach quality properties [20]. During storage, the firmness was reduced, and shelf life of peach fruits treated with zeolite nanoparticles coated with potassium permanganate was increased [42]. Also, when peach fruits were treated with chitosan nanoparticles at 0.8%, fruit pulp firmness and TSSs were maintained [19]. Treatments of tomato fruits with nano-glycyrrhizic acid significantly reduced postharvest losses of fruit since they delayed decay and maintained fruit quality characteristics such as fruit firmness, titratable acidity, and TTSs during cold storage [26]. While it was found that the highest amounts of TSS, fruit set, vitamin C, and calcium content of peach fruit flesh was observed in presence of 20 mg/L f calcium nanoparticles treatment [43].
Current scientific research in Egypt is aimed at limiting or reducing the use of pesticides in the agricultural sector, in particular against postharvest diseases of fruit and vegetables. Natural nanomaterials have emerged as a key solution for integrated pest management programs and considered to be promising tools to manage post-harvest fruit diseases. This may help to produce a safer product leading to increased export of Egyptian peaches.
The present study showed that the exogenous application of GA-NPs was efficient against M. laxa and M. fructigena. On the other hand, GA-bulk showed an acceptable effect against those fungi, but its performance was not as effective as GA-NPs. As a result, GA-NPs could increase the defensive response of peaches against M. laxa and M. fructigena. Employing GA-NPs in agricultural practices may minimize the possibility of chemical control and that discovery is expected to pave the way for a better and more sustainable method of disease management and decrease yield losses. To learn more about how GA-NPs protect peach fruits from M. laxa and M. fructigena infections that are latent, more research is required.
Authorship: The authors confirm contribution to the paper as follows: FA conceived the research idea. TS, BHA and IASR helped to collect the data. MEK, KY and FAA analyzed the data and wrote the paper. All authors reviewed the results and approved the final version of the manuscript.
Funding Statement: The authors received no specific funding for this study.
Conflicts of Interest: The authors declare that they have no conflicts of interest to report regarding the present study.
References
1. El-Badawy, H. (2012). Effect of chitosan and calcium chloride spraying on fruits quality of florida prince peach under cold storage. Research Journal of Agriculture and Biological Sciences, 8(2), 272–281.
2. FAOSTAT (2020). http://www.fao.org/faostat/en/#data/QCL.
3. Byrne, D. (2001). Peach breeding trends: A worldwide perspective. Acta Hortic, 592, 49–59.
4. Tomás-Barberán, F. A., Gil, M. I., Cremin, P., Waterhouse, A. L., Hess-Pierce, B. et al. (2001). HPLC-DAD-ESIMS analysis of phenolic compounds in nectarines, peaches, and plums. Journal of Agricultural and Food Chemistry, 49(10), 4748–4760.
5. Ogawa, J. M., Zehr, E. I., Biggs, A. R. (1995). Brown rot. In: Ogawa, J. M., Zehr, E. I., Bird, G. W., Ritchie, D. F., Uriu, K. et al. (Eds.Compendium of stone fruit diseases, pp. 7–10. USA, Saint Paul: American Phytopathological Society Press.
6. Neri, F., Mari, M., Brigati, S., Bertolini, P. (2007). Fungicidal activity of plant volatile compounds for controlling Monilinia laxa in stone fruit. Plant Disease, 91(1), 30–35.
7. Tamm, L., Flückinger, W. (1993). Influence of temperature and moisture on growth, spore production, and conidial germination of Monilinia laxa. Phytopathology, 83(12), 1321–1326.
8. Tian, S. P., Bertolini, P. (1999). Effect of temperature during conidial formation of Monilinia laxa on conidial size, germination and infection of stored nectarines. Journal of Phytopathology, 147(11–12), 635–641.
9. Lachhab, N., Sanzani, S. M., Fallanaj, F., Youssef, K., Nigro, F. et al. (2015). Protein hydrolysates as resistance inducers for controlling green mould of citrus fruit. Acta Horticulturae, 1065, 1593–1598.
10. Salem, E. A., Youssef, K., Sanzani, S. M. (2016). Evaluation of alternative means to control postharvest Rhizopus rot of peaches. Scientia Horticulturae, 198, 86–90.
11. Youssef, K., Roberto, S. R., Tiepo, A. N., Constantino, L. V., de Resende, J. T. V. et al. (2020). Salt solution treatments trigger antioxidant defense response against gray mold disease in table grapes. Journal of Fungi, 6(3), 179.
12. Jemric, T., Ivic, D., Fruk, G., Matijas, H. S., Cvjetkovic, B. et al. (2011). Reduction of postharvest decay of peach and nectarine caused by Monilinia laxa using hot water dipping. Food Bioprocess and Technology, 4, 149–154.
13. Baggio, J. S., Lourenço, S. A., Amorim, L. (2014). Eradicant and curative treatments of hexanal against peach brown rot. Scientia Agricola, 71(1), 72–76.
14. Abd El wahab, S. M., Rashid, I. A. S. (2015). Effect of a biocontrol agent and modified atmosphere on postharvest control decay and quality retention of peach during storage and marketing life. Middle East Journal of Agriculture Research, 4(4), 660–672.
15. Abo-El Wafa, T. M. M., Youssef, S. E., Ali, M. K. (2019). Control of brown rot on some stone fruits during storage using some salts and inducing resistance. Arab Universities Journal of Agricultural Sciences, 26(2D), 2541–2555.
16. Yang, C., Chen, T., Shen, B., Sun, S., Song, H. et al. (2019). Citric acid treatment reduces decay and maintains the postharvest quality of peach (Prunus persica L.) fruit. Food Science & Nutrition, 7(11), 3635–3643.
17. Alghuthaymi, M. A., Ali, A. A., Hashim, A. F., Abd-Elsalam, K. A. (2016). A rapid method for the detection of Ralstonia solanacearum by isolation dna from infested potato tubers based on magnetic nanotools. Philippine Agricultural Scientist, 99(1), 113–118.
18. Shoala, T. (2018). Positive impacts of nanoparticles in plant resistance against different stimuli. In: Abd-Elsalam, K., Prasad, R. (Eds.Nanobiotechnology applications in plant protection, pp. 267–279. Germany, Cham: Springer.
19. Gad, M. M., Zagzog, O. A., Hemeda, O. M. (2016). Development of nano-chitosan edible coating for peach fruits Cv. Desert red. International Journal of Environment, 5(4), 43–55.
20. Kaur, M., Kalia, A., Thakur, A. (2017). Effect of biodegradable chitosan-rice-starch nanocomposite films on post-harvest quality of stored peach fruit. Starch, 69(1–2), 1600208. DOI 10.1002/star.201600208.
21. Anonymous (1985). 21 CFR Parts 182 and 184 GRAS status of licorice, (GlycyrrhizaAmmoniated glycyrrhizin and monoammonium glycyrrhizinate. Federal Register, 50, 21043–21044.
22. Ming, L. J., Yin, A. C. Y. (2013). Therapeutic effects of glycyrrhizic acid. Natural Product Communications, 8(3), 415–418. DOI 10.1177/1934578X1300800335.
23. Gloria, M. (2003). Encyclopedia of food sciences and nutrition. 2nd editionOxford, UK: Academic Press.
24. Li, J. Y., Cao, H. Y., Liu, P., Cheng, G. H., Sun, M. Y. (2014). Glycyrrhizic acid in the treatment of liver diseases: Literature review. BioMed Research International, 2014, 1–15. DOI 10.1155/2014/872139.
25. Abo-El Wafa, T. M. M. (2019). Biology and control of brown rot fungi on some stone fruits (Ph.D. Thesis). Faculty of Agriculture, Ain Shams University, Egypt.
26. Abdel-Rahman, F. A., Rashid, I. A., Shoala, T. (2020). Nanoactivities of natural nanomaterials rosmarinic acid, glycyrrhizic acid and glycyrrhizic acid ammonium salt against tomato phytopathogenic fungi Alternaria alternata and Penicillium digitatum. Journal of Plant Protection Research, 60(2), 150–160.
27. Youssef, K., Hashim, A. F., Margarita, R., Alghuthaymi, M. A., Abd-Elsalam, K. A. (2017). Fungicidal efficacy ofchemically-produced copper nanoparticles against Penincillium digitatum and Fusarium solani on citrus fruit. Philippine Agricultural Scientist, 100(1), 69–78.
28. Hashim, A. F., Youssef, K., Abd-Elsalam, K. A. (2019). Ecofriendly nanomaterials for controlling gray mold of table grapes and maintaining postharvest quality. European Journal of Plant Pathology, 154, 377–388.
29. Kim, S. W., Jung, J. H., Lamsal, K., Kim, Y. S., Min, J. S. et al. (2012). Antifungal effects of silver nanoparticles (AgNPs) against various plant pathogenic fungi. Mycobiology, 40(1), 53–58.
30. Melo, I. S., Faull, J. L. (2004). Scanning electron microscopy of conidia of Thichoderma stromaticum, a biocontrol agent of witches broom disease of cocoa. Brazilian Journal of Microbiology, 35(4), 330–332.
31. Basma, H. A. (2013). Studies on antifungal agents extracted from some desert and cultivated plants (Ph.D. Thesis). Botany and Microbiology Department, Al–Azhar Univerity, Egypt.
32. Basma, H. A. (2016). Isolation and characterization of antiprotozoal and antimicrobial metabolite from Penicillium roqueforti. African Journal of Biotechnology, 21(3), 13–26.
33. Youssef, K., Ahmed, Y., Ligorio, A., D’Onghia, A. M., Nigro, F. et al. (2010). First report of Penicillium ulaiense as a postharvest pathogen of orange fruit in Egypt. Plant Pathology, 59(6), 1174.
34. Hazali, N., Tajudin, A. R. A., Nor, N. M., Ibrahim, M., Yahya, M. N. A. (2013). Physicochemical characteristics of Belimbing Dayak (Baccaurea angulata) juice beverages. European International Journal of Science and Technology, 2(2), 203–210.
35. Youssef, K., de Oliveira, A. G., Tischer, C. A., Hussain, I., Roberto, S. R. (2019). Synergistic effect of a novel chitosan/silica nanocomposites based formulation against gray mold of table grapes and its possible mode of action. International Journal of Biological Macromolecules, 141, 247–258.
36. Park, H. J., Kim, S. H., Kim, H. J., Choi, S. H. (2006). A new composition of nanosized silica-silver for control of various plant diseases. Plant Pathology Journal, 22(3), 295–302.
37. Chookhongkha, N., Sopondilok, T., Photchanachai, S. (2013). Effect of chitosan and chitosan nanoparticles on fungal growth and chilli seed quality. Acta Hortic, 973, 231–237.
38. Cota-Arriola, O., Cortez-Rocha, M. O., Ezquerra-Brauer, J. M., Lizardi Mendoza, J., Burgos-Hernández, A. et al. (2013). Ultrastructural, morphological, and antifungal properties of micro and nanoparticles of chitosan crosslinked with sodium tripolyphosphate. Journal of Polymers and the Environment, 21, 971–980.
39. Kotzybik, K., Grk, V., Kugler, L., Stoll, D. A., Greiner, R. et al. (2016). Influence of different nanomaterials on growth and mycotoxin production of Penicillium verrucosum. PLoS One, 11(3), e0150855.
40. Xia, Z. K., Ma, Q. H., Li, S. Y., Zhang, D. Q., Cong, L. et al. (2016). The antifungal effect of silver nanoparticles on Trichosporon asahii. Journal of Microbiology, Immunology and Infection, 49(2), 182–188.
41. Abdel-Rahman, F. A., Khafagi, E. Y., Soliman, M. S., Shoala, T., Ahmed, Y. (2021). Preharvest application of salicylic acid induces some resistant genes of sweet pepper against black mold disease. European Journal of Plant Pathology, 159, 755–768.
42. Emadpour, M., Ghareyazie, B., Kalaj, Y. R., Entesari, M., Bouzari, N. (2015). Effect of the potassium permanganate coated zeolite nanoparticles on the quality characteristic and shelf life of peach and nectarine. International Journal of Agricultural Technology, 11(6), 1411–1421.
43. Kiafar, H., Mousavi, M., Ebadi, A., Moallemi, N., Moghadam, M. R. F. (2020). Effect of Ca nanoparticles on peach cultivars (Valad Abadi and Alberta). Journal of Agricultural Engineering, 43(1), 1–14.
Cite This Article
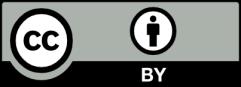
This work is licensed under a Creative Commons Attribution 4.0 International License , which permits unrestricted use, distribution, and reproduction in any medium, provided the original work is properly cited.