Open Access
ARTICLE
Silicon and Nitric Oxide-Mediated Regulation of Growth Attributes, Metabolites and Antioxidant Defense System of Radish (Raphanus sativus L.) under Arsenic Stress
1 Department of Botany, School of Bioengineering and Biosciences, Lovely Professional University, Phagwara, 144411, India
2 College of Agriculture, Fujian Agriculture and Forestry University (FAFU), Fuzhou, 350002, China
* Corresponding Authors: Ali Raza. Email: ; Dhriti Kapoor. Email:
(This article belongs to the Special Issue: Physiological and Molecular Interventions in Improving Abiotic Stress Tolerance in Plants)
Phyton-International Journal of Experimental Botany 2023, 92(3), 763-782. https://doi.org/10.32604/phyton.2023.025672
Received 25 July 2022; Accepted 15 September 2022; Issue published 29 November 2022
Abstract
Arsenic (As) contaminated food chains have emerged as a serious public concern for humans and animals and are known to affect the cultivation of edible crops throughout the world. Therefore, the present study was designed to investigate the individual as well as the combined effects of exogenous silicon (Si) and sodium nitroprusside (SNP), a nitric oxide (NO) donor, on plant growth, metabolites, and antioxidant defense systems of radish (Raphanus sativus L.) plants under three different concentrations of As stress, i.e., 0.3, 0.5, and 0.7 mM in a pot experiment. The results showed that As stress reduced the growth parameters of radish plants by increasing the level of oxidative stress markers, i.e., malondialdehyde and hydrogen peroxide. However, foliar application of Si (2 mM) and pretreatment with SNP (100 µM) alone as well as in combination with Si improved the plant growth parameters, i.e., root length, fresh and dry weight of plants under As stress. Furthermore, As stress also reduced protein, and metabolites contents (flavonoids, phenolic and anthocyanin). Activities of antioxidative enzymes such as catalase (CAT), ascorbate peroxidase (APX), guaiacol peroxidase (POD), and polyphenol oxidase (PPO), as well as the content of non-enzymatic antioxidants (glutathione and ascorbic acid) decreased under As stress. In most of the parameters in radish, As III concentration showed maximum reduction, as compared to As I and II concentrations. However, the individual and combined application of Si and NO significantly alleviated the As-mediated oxidative stress in radish plants by increasing the protein, and metabolites content. Enhancement in the activities of CAT, APX, POD and PPO enzymes were recorded. Contents of glutathione and ascorbic acid were also enhanced in response to co-application of Si and NO under As stress. Results obtained were more pronounced when Si and NO were applied in combination under As stress, as compared to their individual application. In short, the current study highlights that Si and NO synergistically regulate plant growth through lowering the As-mediated oxidative stress by upregulating the metabolites content, activity of antioxidative enzymes and non-enzymatic antioxidants in radish plants.
Keywords
Crop yield and productivity are hindered due to the presence of noxious elements in the soil, among which arsenic (As) is a non-essential metalloid present in the natural ecosystem. It is highly disadvantageous to plants and animals and is distributed throughout the world [1]. Arsenic enters the ecosystem through mining, industries, volcanic emissions, excessive use of fertilizers, insecticides, etc., and is mostly contained in domestic and industrial sewage sludge [2,3]. Various characteristics of soil and soil solution, and their associations with other noxious moieties, determine the bioavailability of As in the soil at any stage [4]. The accumulation of As in crop plants takes place either by the existence of As in soils or its occurrence in irrigation water. The presence of As in soil impedes food safety by adversely affecting plant growth and development and may become a part of the food chain [5]. Arsenic accumulation in crops is known to cause various morphological, physiological, biochemical, and molecular alterations [6,7]. Arsenic stress cause excessive release of reactive oxygen species (ROS) such as hydroxyl (OH.), superoxide (O2.−) radicals, and hydrogen peroxide (H2O2) in plants, which ultimately cause oxidative stress and damage the cell membranes. Plants possess antioxidant defense systems, i.e., enzymatic and non-enzymatic antioxidants, to minimize the noxious effects of excessive ROS, which helps to cope with the As-mediated oxidative stress [8].
Arsenic contamination has now been controlled by the use of eco-friendly substances, among which nitric oxide (NO) and silicon (Si) have received much attention recently. Nitric oxide is a small gaseous plant signaling molecule that plays a vital role in mediating plant growth and development under both normal physiological as well as biotic and abiotic conditions [9]. Depending on its rate of production, it acts both as an antioxidant and a pro-oxidant, as at a lower quantity, it functions as a beneficial material to protect against environmental stresses. At the same time, at larger amounts, it causes nitroso-oxidative stress and acts as a stress molecule [10]. Nitric oxide is also considered to reduce metal toxicity due to its signaling role and stimulates the plant’s antioxidant defense system to decline oxidative stress by decomposing H2O2 to molecular oxygen and triggering H2O2-suppressing enzymes [11–13]. An increase in the activities of catalase (CAT), superoxide dismutase (SOD), and ascorbate peroxidase (APX) enzymes showed a positive role of NO in the activation of stress-responsive enzymes [14]. Aluminium (Al) and As stress was significantly mitigated by the exogenous application of NO in wheat and rice plants [15,16]. Activities of antioxidant enzymes were improved by the exogenous application of NO to detoxify the overproduction of ROS [17,18]. Increased growth and reduced synthesis of H2O2 and MDA under As stress were observed in plants with exogenously applied NO [19]. Abbas et al. [20] reported reduced uptake of As and subsequent translocation from root to shoot in NO-treated plants.
Silicon is one of the essential mineral elements, which is 2nd most abundant mineral element on Earth’s crust after oxygen and is available to plants in the form of silicic acid [Si(OH)4] [21,22]. Silicon is highly considered as a beneficial nutrient as it increases various growth and metabolic activities such as growth parameters, photosynthetic activity, metabolism, antioxidant defense system etc., to improve plant tolerance to multiple environmental stresses [22–24]. Sustainable crop productivity can be enhanced by the accumulation of Si as it protects plants from various abiotic stresses such as metals, temperature, salinity, and drought [22,25,26]. Silicon also regulates various molecular aspects of the plants under both normal and stressed conditions [22,27,28]. Several researchers have found that different metal and metalloid stresses such as lead (Pb), As, cadmium (Cd), mercury (Hg), Al, manganese (Mn), zinc (Zn), copper (Cu), or antimony (Sb) can be mitigated by the exogenous application of Si in various plant cultivars [29–31]. Plants’ phenolics and flavonoids play important roles, notably in the antioxidant defence system, and aid the plants to perform metabolic flexibility to acclimatize under heavy metal stresses [32]. Phenolic compounds are considered to be associated with the mitigation of oxidative stress to stabilize the cellular membrane, due to their role in metal chelating and radical scavenging. Hence, the presence of phenolic compounds is thought to be an adaptive mechanism for dealing with metal toxicity [33].
Radish (Raphanus sativus L.) belongs to the Brassicaceae family and is an imperative annual root vegetable crop with diverse edible and therapeutic uses. Radish is rich in dietary fibres, carbohydrates, proteins, even some fats, water-soluble vitamins, mineral nutrients, etc. [34]. As a model crop, radish is a well-known crop as a hyperaccumulator of metals and is highly sensitive to various heavy metal stresses [35–37]. It is one of the most important horticulture and medicinal crop, which is a good source of ascorbic acid, folic acid, and potassium, and is rich in active constituents like glucosinolates and isothiocyanates [38,39].
Arsenic uptake in plants adversely affects morpho-anatomical, biochemical, physiological, and molecular aspects. Therefore, reduction in As uptake, translocation, and accumulation from soil to radish plants has recently become a global issue for improving its growth, and productivity and to fulfil the food demand of an ever-increasing world population. A variety of methods can be used to improve As tolerance in plants to limit or lessen As uptake by the roots, and to reduce its translocation to the upper plant parts [40]. However, As remediation through the physical method, thermal desorption, chemical, and electrokinetic remediation have not been successful so far due to their cost, effectiveness, and environmental friendly remediation issue. Therefore, a reduction in As toxicity is urgently needed through the application of safe and cost-effective substances. In this context, exogenous application of the micro-nutrient Si and the gaseous signaling molecule NO could be a cost-effective, efficient and eco-friendly strategy to improve plant tolerance to As contamination. Moreover, a few studies have been conducted on the combined effects of Si and NO under metal stresses [41,42], but no study has been conducted on the combined effects of Si and NO on radish under As stress. Therefore, the present study was intended to investigate the individual as well as combined effects of exogenously applied Si and NO on plant growth, metabolites, and antioxidant defense system in radish plants under As toxicity.
2.1 Plant Materials, Growth Conditions and Treatments
Seeds of radish (Punjab safed mooli 2) were procured from Punjab Agricultural University, Ludhiana (Punjab), India. The present experiment was carried out under natural environmental conditions at the agricultural farm of Lovely Professional University, Phagwara, Punjab. Surface sterilization of seeds was done with 1% sodium hypochlorite for 5 min and washed three times with distilled water.
Seeds of radish were then soaked in 100 µM sodium nitroprusside (SNP) solution, which is a NO donor, for 8 h, and for the same time duration, the remaining seeds were dipped in distilled water. Thirty seeds were then sown in each plastic pot (total 48 pots, 24 cm in diameter and 40 cm in height) which were filled with field soil and organic manure (vermicompost) in a ratio of 3:1. Arsenic was applied in the form of sodium arsenate to the soil. In the preliminary study, a 50% reduction in growth was observed at 0.5 mM As concentration, so the final concentrations chosen for the present study were 0.3, 0.5, and 0.7 mM. Effective concentrations of Si (2 mM) and NO (100 µM) were chosen based on the highest plant growth observed (these preliminary results are unpublished). Fifteen plants were retained in each pot after the seedling emergence. Plants were exogenously supplied with a foliar spray of Si in the form of silicic acid at 2 mM concentration after 5 days of seedling emergence. The selected pots for Si treatment were sprayed with 150 mL silicic acid. Plants were then harvested on the 30th day after sowing to evaluate various morphological and biochemical parameters. Plant leaves were used for further analysis. The experiment included 16 treatments: (1) control, (2) As I (0.3 mM), (3) As II (0.5 mM), (4) As III (0.7 mM), (5) Si, (6) Si + As I, (7) Si + As II, (8) Si + As III, (9) NO, (10) NO + As I, (11) NO + As II, (12) NO + As III, (13) Si + NO, (14) Si + NO + As I, (15) Si + NO + As II and (16) Si + NO + As III.
2.2 Measurement of Growth Parameters
In the growth parameters, root length (cm), fresh and dry weight (mg) of whole plants were measured in 30 days old plants of radish.
2.3.1 Malondialdehyde (MDA) Concentration
Heath et al. [43] protocol was followed to measure MDA concentration. Briefly, 0.1% trichloroacetic acid (TCA) was used to homogenize 1 g of leaf tissue, followed by centrifugation at 5,000 rpm. Then 20% TCA containing 0.5% thiobarbituric acid (TBA) was mixed with 1 mL of supernatant, which was then incubated at 95°C for half an hour followed by cooling on ice. Absorbance of the supernatant was read at 532 nm and correction of nonspecific absorbance was done by subtraction of absorbance taken at 600 nm. The extinction coefficient used was 155 mM−1 cm−1.
2.3.2 Hydrogen Peroxide (H2O2) Concentration
Hydrogen peroxide concentration was measured according to the method of Velikova et al. [44]. Briefly, 0.1% of TCA was used for the homogenization of 100 mg of leaf tissue and centrifugation was carried out at 12,000 rpm for 15 min. Then, 0.4 and 0.8 mL of potassium phosphate buffer (10 mM, pH 7.0) and potassium iodide (1 M, pH 7.4) respectively were mixed with the 0.5 mL of supernatant. The absorbance of the sample was measured at 390 nm. H2O2 was used as standard and plotted in the standard curve to calculate the H2O2 concentration.
To measure the flavonoid concentration, 500 mg of fresh leaf tissue was homogenized in 3 mL of absolute methanol. Centrifugation was carried out at 13,000 rpm for 20 min. Then, 4 mL of double distilled water (DDW), 0.3 mL of sodium nitrite (NaNO2) and 0.3 mL of aluminum chloride (AlCl3) were added to the 1 mL of supernatant and then incubated for 5 min in the dark. Pink color was appeared after the incorporation of 2 mL sodium hydroxide (NaOH) into it. Then 2.4 mL of distilled water was added. Rutin was taken as standard for the calculation of flavonoid concentration through the standard curve and 510 nm wavelength was set to take the optical density. Flavonoid concentration was measured by the method of Kim et al. [45].
Phenolic concentration was analyzed by following the method of Malick et al. [46]. Briefly, 0.5 g of leaves were crushed in 80% ethanol. Homogenate was then centrifuged for 20 min at 10,000 rpm. After this, 0.5 mL of folin-ciocalteu reagent and 20% sodium carbonate (Na2CO3) were mixed with the supernatant. Optical density was read at 650 nm and gallic acid was used as reference standard.
2.4.3 Anthocyanin Concentration
Mancinelli [47] protocol was followed to evaluate total anthocyanin concentration. Extraction mixture was prepared by mixing methanol:water:HCl in the ratio of 79:20:1. Then 3 mL of this extraction mixture was taken to crush 0.5 g of fresh leaf tissue. Centrifugation of the homogenate was performed at 13,000 rpm for 20 min. Optical density of the supernatant was read at 530 and 657 nm wavelength.
2.5 Protein Concentration and Activities of Antioxidative Enzymes
Lowry [48] protocol was followed to estimate protein concentration. Briefly, 3 mL phosphate buffer was used for the extraction of 500 mg leaf tissue followed by centrifugation at 10,000 rpm for 10 min. Further, 0.9 mL distilled water was added in 0.1 mL of the supernatant to make it 1 mL. 5 mL of reagent C [mixture of reagent A (sodium carbonate in sodium hydroxide) + reagent B (copper sulphate in potassium sodium tartarate)] was added to each tube. It was mixed well and was allowed to stand for 10 min. Then 0.5 mL of reagent D (folin-ciocalteu reagent) was added, mixed well and incubated at room temperature in the dark for 30 min. Blue color was developed. The readings were taken at 660 nm. Bovine serum albumin (BSA) was used as a reference standard.
2.5.2 Activities of Antioxidative Enzymes
CAT activity was evaluated by the standard protocol given by Aebi [49]. Briefly, 1 g of leaf tissue was used for extracting enzyme in 3 mL phosphate buffer (100 mM, pH 7.0) and centrifuged at 5,000 rpm for 20 min. Change in absorbance was measured at 240 nm by adding 50 µL of plant sample containing 2.650 mL of 100 mM phosphate buffer and 300 µL of H2O2 (150 mM). The extinction coefficient used was 43.6 mM−1 cm−1.
APX enzyme activity was estimated by the standard protocol of Nakano et al. [50] method. Briefly, 1 g of leaf tissue was used for extracting enzyme in 3 mL phosphate buffer (100 mM, pH 7.0). Centrifugation was carried out at 5,000 rpm for 20 min. After this, 50 µL of plant sample was added the in the cuvette containing 2.370 mL phosphate buffer (100 mM, pH 7.0), 0.3 mL ascorbate (5 mM) and 0.3 mL H2O2 (0.5 mM). The absorbance was recorded at 290 nm. The extinction coefficient used was 2.8 mM−1 cm−1.
POD enzyme activity was measured by following the standard protocol of Putter [51]. Briefly, 1 g of leaf tissue was used for extracting enzyme in 3 mL phosphate buffer (100 mM, pH 7.0). Centrifugation was carried out at 5,000 rpm for 20 min. Then, 50 µL of plant sample was added in the cuvette containing 2.370 mL phosphate buffer (0.1 M, pH 7.0), 0.3 mL guaiacol (20 mM) and 0.3 mL H2O2 (12.3 mM). The absorbance was recorded at 436 nm. The extinction coefficient used was 25.5 mM−1 cm−1.
Polyphenol oxidase (PPO) enzyme activity was measured by Kumar et al. [52] method. Briefly, 50 µL of leaf sample was added in the cuvette containing 1950 µL of phosphate buffer (0.1 M), 0.5 mL catechol (0.1 M), and 0.5 mL of 2.5 N sulfuric acid (H2SO4). The absorbance was taken at 495 nm. The extinction coefficient used was 2.9 mM−1 cm−1.
2.6 Non-enzymatic Antioxidants
Ascorbic acid concentration was measured by the method given by Roe et al. [53]. Briefly, 0.5 g of leaf tissue was homogenized in 50 mM tris buffer (pH 10.0) followed by centrifugation at 13,000 rpm for 20 min. To the 0.5 mL of leaf extract, 100 mg charcoal, 4 mL of DDW, and 0.5 mL of 50% TCA were added, followed by filtration with Whatman filter paper 1. Incubation was given at 37°C for 3 h after adding 0.4 mL of DNPH (2,4-dinitrophenyl hydrazine) to filtrate followed by addition of 1.6 mL cold H2SO4 (65%). The mixture was then kept at room temperature for 30 min. Ascorbic acid (1 mg 100 mL−1) was used as standard. The absorbance was measured at 520 nm.
2.6.2 Glutathione (GSH) Concentration
The concentration of glutathione was analyzed by the method given by Sedlak et al. [54]. Briefly, 1 g of leaf tissue was extracted in 3 mL tris buffer (50 mM, pH 10.0) followed by centrifugation at 13,000 rpm for 20 min. Then, 1 mL of tris buffer (0.2 M, pH 8.2), 50 µL of 0.01 M DTNB [(5,5′-dithiobis-(2-nitrobenzoic acid)] and 4 mL of absolute methanol, were added in the test tube containing 100 µL of supernatant. Incubation was done at room temperature for 15 min. The reaction mixture was again centrifuged for 15 min at 3,000 rpm. Furthermore, 1 mg 100 mL−1 glutathione was used as a standard for the determination of glutathione concentration. Optical density was measured at 412 nm.
Data were analyzed by using SPSS 16.0 software (SPSS Inc., Chicago, IL, USA). The statistical significance was performed with a one-way analysis of variance (ANOVA) and Tukey’s test at P < 0.05 level of significance between the treatments. Means with dissimilar letters in the bar graphs are statistically significant at a P value less than 0.05. Triplicates (three pots per replication) were used for each experiment and performed two times independently (total of 6 samples per treatment). Data is expressed as the means ± SEM in figures. Principal component analysis (PCA) was performed using fviz-pca function of the factoextra R package Ver. 1.0.7 in RStudio. Pearson’s correlation analysis was performed using the mcor function, and corrplots were prepared using corrplot package Ver. 0.89 in RStudio.
3.1 Si and NO-Mediated Plant Growth Parameters under As Stress
Effect of exogenous application of Si and NO under As stress on plant growth parameters were evaluated in terms of root length (Fig. 1A), fresh weight (Fig. 1B), and dry weight of whole plant (Fig. 1C). Root length was reduced by 32%, 42% and 56%; at 0.3, 0.5 and 0.7 mM As concentrations, respectively, as compared to control plants. Individual application of Si and NO significantly improved the root length. Only a 4% reduction in root length was observed in the case of Si + As I treatment when compared to control plants. Combined application of Si + NO + As I caused a 22% increase in the root length, as compared to control plants.
Figure 1: Effect of Si and NO on root length (A), fresh weight (B) and dry weight (C) in radish plants under As stress. Each value denotes the mean of three replicates for every treatment level along with standard error of mean (SEM). Letters above a column followed by dissimilar letters above other columns are significantly different at P < 0.05. CN-control; Si-silicon; NO-nitric oxide; As I-0.3 mM; As II-0.5 mM; As III-0.7 mM
Results revealed that As treatment also caused a decrease in the fresh and dry weights with 65%, 58% and 68%; 58%, 60% and 63% at As I, As II and As III concentrations, respectively. But the addition of Si and NO significantly alleviated the As-mediated toxicity in radish plants. Under unstressed conditions, fresh and dry weights were increased by 56%, and 151% in Si applied plants while 48%, and 163% were observed in NO pre-treated plants, respectively, as compared to control plants. In case of NO + As I treated plants, an increase of 9% in fresh weight and 114% in dry weight was observed, in comparison to control plants. Combined application of Si and NO under As stress caused a reduction in fresh and dry weights by 19%, 22% and 27%; 14%, 26% and 30% at As I, As II and As III concentrations, respectively, in comparison to their respective control plants, i.e., Si + NO.
3.2 Si and NO-Mediated Regulation of Oxidative Stress Markers in As Stressed Plants
Oxidative stress was observed to be high in the case of As-treated plants in terms of MDA and H2O2 levels. As I, As II and As III concentrations caused 28%, 27% and 36% elevation in the MDA concentration, respectively, as compared to control plants (Fig. 2A). However, individual application of Si and NO at As I, As II and As III concentration reduced the level of MDA with 18%, 22% and 28%; 20%, 15% and 25%, respectively in comparison to control plants. The level of H2O2 was also found to be increased under all three As concentrations among which the highest H2O2 level was at As III concentration. Individual as well as combined application of Si and NO caused a significant decrease in the level of H2O2 as compared to As alone treated plants. A 47% increase in the H2O2 level was found in Si + As III applied plants, as compared to As III alone treated plants (Fig. 2B). Combined application of Si + NO under As I and As III concentration caused a decline of 6% and 25%, respectively, in comparison to their respective control plants, i.e., Si + NO.
Figure 2: Effect of Si and NO on MDA (A) and H2O2 (B) level in radish plants under As stress. Each value denotes the mean of three replicates for every treatment level along with standard error of mean (SEM). Letters above a column followed by dissimilar letters above other columns are significantly different at P < 0.05. CN-control; Si-silicon; NO-nitric oxide; As I-0.3 mM; As II-0.5 mM; As III-0.7 mM
3.3 Influence of Si and NO on Plant Metabolites under As Stress
Flavonoid concentrations drastically declined in all the As stressed radish plants with a decrease of 26%, 27% and 29% at As I, As II and As III concentrations, respectively (Fig. 3A). Silicon application under stressed conditions caused alleviation of As stress by increasing the flavonoid concentration. Among these, Si + As II treated plants caused only a 6% reduction in flavonoid concentration when compared with the Si control plants. The combined application of Si and NO caused 19%, 24% and 17% increase in the flavonoid concentration at As I, As II and As III concentrations, respectively, as compared to control plants.
Figure 3: Effect of Si and NO on flavonoid (A), phenolic (B), anthocyanin (C) and protein (D) concentration in radish plants under As stress. Each value denotes the mean of three replicates for every treatment level along with standard error of mean (SEM). Letters above a column followed by dissimilar letters above other columns are significantly different at P < 0.05. CN-control; Si-silicon; NO-nitric oxide; As I-0.3 mM; As II-0.5 mM; As III-0.7 mM
As stress caused a decline in the phenolic concentration in radish plants with a decrease of 20%, 40% and 44% at As I, As II and As III concentrations, respectively when compared with control plants (Fig. 3B). Individual as well as combined application of Si and NO improved the As mediated decrease in the phenolic concentration. A maximum increase in the phenolic concentration was observed in Si + NO and NO control plants by 26% and 13%, respectively, as compared to control plants. Silicon and NO in combination under As II stress showed better concentration of phenolic as compared to Si + NO + As I and Si + NO + As III treated plants.
Arsenic stressed radish plants exhibited a decrease in anthocyanin concentration by 31%, 32% and 41% in contrast to the control plants. In the present study, application of Si and pretreatment with NO alone and in combination enhanced the anthocyanin concentration. Individual application of Si under As I, II and III concentrations caused only a decrease of 15%, 21% and 17% in anthocyanin concentration (Fig. 3C), when compared with control plants. Whereas individual application NO at As I caused a minor increase of 2%, and a decrease of 3% and 8% at As II and III concentrations, respectively, as compared to control plants. The maximum concentration of anthocyanin among Si + NO treatments under As stress was found at As I concentration.
3.4 Si and NO-Mediated Protein Concentration and Antioxidative Enzymes Activity
Total protein concentration was observed to be decreased due to exposure to As in radish plants. It was found to be decreased by 14%, 14% and 21% at As I, II, and III concentrations, respectively, as compared to control plants (Fig. 3D). On the other hand, application of Si, NO and Si + NO significantly mitigated the toxic effects of As in radish plants. In addition to this, Si, NO, and Si + NO control plants significantly influenced protein concentration with an increase of 22%, 24% and 29% over the control plants. In the Si and NO combined plants under As stress, maximum protein concentration was observed at As I concentration. Only a 13% decrease in the protein concentration was found in Si + NO + As I treated plants, when compared with As I stressed plants.
In the present study, the activities of antioxidative enzymes, i.e., CAT, APX, POD, and PPO were evaluated. In As-treated plants, the activity of CAT enzyme was observed to be reduced as compared to control plants. The activity of CAT was found to be increased by 21%, 13% and 34% in Si, NO and Si + NO control plants, respectively as compared to control plants (Fig. 4A). Exogenous application of Si under As stress augmented the CAT activity in comparison to As alone stressed plants. In the case of combined application of Si + NO under As stress, maximum increase in the CAT activity was at As III concentration (30%), over the control plants.
Figure 4: Effect of Si and NO on CAT (A), APX (B), POD (C) and PPO (D) in radish plants under As stress. Each value denotes the mean of three replicates for every treatment level along with standard error of mean (SEM). Letters above a column followed by dissimilar letters above other columns are significantly different at P < 0.05. CN-control; Si-silicon; NO-nitric oxide; As I-0.3 mM; As II-0.5 mM; As III-0.7 mM
The activity of APX enzyme was also found to be improved by the supplementation of Si and NO under As stress. Application of Si under As I stress showed only a 5% increase in the APX activity, in contrast to control plants. Pretreatment with NO caused elevation of 10% and 6% under As I and II concentrations, respectively, while observed only a decrease of 3% in case of As III concentration as compared to control plants (Fig. 4B). Co-application of Si and NO significantly alleviated the As-mediated adverse effects on APX activity by reduction of only by 16%, 8%, and 21% at As I, II and III concentrations as compared to their respective control plants, i.e., Si + NO.
Arsenic stress also caused a decline in the POD activity when compared with the control plants. However, the application of Si and NO resulted in a significant increase in the POD activity as compared to As-stressed plants. Maximum POD activity was observed at Si + NO treatment followed by NO control plants. Individual pretreatment with NO caused elevation in the POD activity by 8%, 3% and 3% at As I, II and III concentrations, respectively, when compared with control plants (Fig. 4C). In Si + NO plants under As II stress, 6% increase in the activity of POD was noticed, as compared to control plants.
Polyphenol oxidase enzyme activity was observed to be reduced under As stress with a maximum reduction of 41% at As III concentration, as compared to control plants. Individual as well as combined application of Si and NO improved the PPO activity of radish plants under As stress (Fig. 4D). An increase of 19% and 22% was noticed in Si and NO control plants, respectively as compared to control plants. Individual application of Si and NO resulted in a reduction of only 18%, 21% and 10%; 14%, 5% and 8% at As I, II and III concentrations when compared with their respective control plants. Combined application of Si and NO under As stress showed maximum PPO activity at As III concentration with a 33% increase in comparison to control plants.
3.5 Effect of Si and NO on Non-Enzymatic Antioxidants of Radish under As Stress
Glutathione concentration was found to be decreased with the increase in the As concentration. Minimum glutathione concentration was in As III stressed plants i.e., a decrease of 39% as compared to control plants (Fig. 5A). Silicon and NO control plants showed an increase of 5% and 1%, as compared to control plants. Individual application of Si mitigated the As toxicity by showing a decrease of only 13%, 10% and 6% at As I, II and III concentrations, as compared to their respective control plants. Combined supplementation of Si and NO increased the glutathione concentration by 10%, 8% and 5% as compared to control plants.
Figure 5: Effect of Si and NO on glutathione (A) and ascorbic acid (B) concentration in radish plants under As stress. Each value denotes the mean of three replicates for every treatment level along with standard error of mean (SEM). Letters above a column followed by dissimilar letters above other columns are significantly different at P < 0.05. CN-control; Si-silicon; NO-nitric oxide; As I-0.3 mM; As II-0.5 mM; As III-0.7 mM
The ascorbic acid amount was also declined in the case of As stressed plants. At As I, II and III concentrations, a decline of 26%, 36% and 41% was observed, when compared with control plants. However, individual as well as combined application of Si and NO significantly improved the ascorbic acid concentration under As stress (Fig. 5B). Maximum concentration was observed at Si + NO control plants, among all the treatments used in this study. In the case of combined treatment of Si and NO, only a decrease of 11%, 7% and 12% was observed at As I, II and III concentrations as compared to their respective control plants, i.e., Si + NO.
3.6 Principal Component and Correlation Analysis
To appraise the impact of Si and NO treatments on the explored traits of radish plants, the score and loading diagrams of PCA were implemented (Fig. 6). The first two components, i.e., Dim1 (PC1, 84.4%) and Dim2 (PC2, 6.1%) exhibited the highest participation and represented 90% of the total variance in the dataset. Pertaining to Si, NO, and As application, similar treatments at various levels/combinations were grouped nearby. On the other hand, the various treatments were divided well by the first two components (Fig. 6A). This division of the diverse treatments obviously suggested that the NO and Si treatments under As stress had a strong ameliorative effect on the examined traits of radish plants than CN. The first set of the variables of PCA (PC1), is found to be positively correlated with most of the variables such as root length, fresh weight, dry weight, flavonoid, protein, phenolic, anthocyanin, catalase, ascorbate peroxidase, guaiacol peroxidase, polyphenol oxidase, glutathione, and ascorbic acid (Fig. 6B). In contrast, a noteworthy negative correlation of PC1 variables (H2O2 and MDA) was found to be in line up with PC2 (Fig. 6B).
Figure 6: Principal component analysis (PCA) of (A) individual treatments by PCA and (B) diverse analyzed parameters of radish plants under As stress. (A) Score plot indicates the separation of treatments of NO, As, Si, Si + NO, NO + As, Si + As and Si + NO + As
Abbreviations: control (CN); silicon (Si); nitric oxide (NO); root length (RL); fresh weight (FW); dry weight (DW); malondialdehyde (MDA); hydrogen peroxide (H2O2); flavonoid (FL); protein (Prot); phenolic (Phen); anthocyanin (Ant); catalase (CAT); ascorbate peroxidase (APX); guaiacol peroxidase (POD); polyphenol oxidase (PPO); glutathione (GSH); ascorbic acid (AsA).
A Pearson’s correlation analysis was executed between diverse analyzed traits of radish plants (Fig. 7). The correlation analysis showed that H2O2 and MDA were negatively correlated with other traits such as root length, fresh weight, dry weight, flavonoid, protein, phenolic, anthocyanin, catalase, ascorbate peroxidase, guaiacol peroxidase, polyphenol oxidase, glutathione, and ascorbic acid. In contrast, all other traits showed a strong positive correlation with each other (Fig. 7). This correlation characterizes a close bond between growth traits, metabolites, antioxidant defense systems and NO and Si-mediated As tolerance in radish plants.
Figure 7: Pearson’s correlation analysis between diverse analyzed parameters of radish plants under As stress. Blue and brownish colors indicate positive and negative correlation, correspondingly
Abbreviations: control (CN); silicon (Si); nitric oxide (NO); root length (RL); fresh weight (FW); dry weight (DW); malondialdehyde (MDA); hydrogen peroxide (H2O2); flavonoid (FL); protein (Prot); phenolic (Phen); anthocyanin (Ant); catalase (CAT); ascorbate peroxidase (APX); guaiacol peroxidase (POD); polyphenol oxidase (PPO); glutathione (GSH); ascorbic acid (AsA).
Inhibitory effects of As on plant growth parameters were observed in various studies [55–57]. In the present study, As stress negatively affected the growth and biomass of radish plants. Chromium stress caused ultrastructural alterations in various parts of plants and degradation of chlorophyll pigments in Brassica napus [58]. Similar impairments could be involved in the As-mediated reduction in the growth of radish plants. However, our results showed that individual as well as combined application of Si and NO mitigated As-mediated toxicity and improved the growth of radish plants. Previous studies indicated that NO [59–61] and Si [62,63] increased the growth and biomass of plants due to their impacts on lowering heavy metal uptake or improving uptake of essential nutrients and elevating photosynthetic pigments. Saleem et al. [64] found that the application of Si to Zea mays plants increased the root length, and plant biomass under Cd stress. Si triggered an improvement in nutrient uptake and played a defensive role on root structure to increase the plant growth under metal stress (Cu in wheat and Ni in cotton seedlings, respectively) [65,66]. Biomass production can be improved through the application of Si in the form of soluble silicic acid Si(OH)4, which ultimately stimulates biochemical and molecular processes [67]. Shoot length and dry weight were observed to be increased by the addition of NO under Pb and Cd stress in Arundinaria pygmaea [12]. Similar findings were observed by Singh et al. [68] in terms of an increase in root length, shoot length, and biomass under As stress in Oryza sativa plants. The stimulated activity of antioxidant enzymes and osmolytes, decline in electrolyte leakage, and metal accretion in different plant parts are some of the major factors behind the positive role of NO on plant growth under stressed conditions [69].
Phenolic compounds, which are formed by shikimic acid or phenyl-propanoid pathways, act as imperative antioxidants under different environmental stresses [70]. During the present study, the concentration of metabolites, i.e., flavonoids, phenolics, and anthocyanin, were found to be increased by the application of Si and NO under As toxicity. Similar findings were observed by Pirooz et al. [71] in terms of Si and NO-mediated increase in phenolics and flavonoids in Salvia officinalis under Cu stress. The stimulation of phenylpropanoid pathway could be the reason for the Si-mediated increase in the metabolites concentration under As stress as described by Dar et al. [72] in Fagopyrum esculentum under Al stress. Flavonoid concentration was increased by the application of Si NPs to coriander plants under Pb stress to improve the plant’s tolerance against metal stress [73]. Silicon-mediated mitigation of metal stress in plants is due to the metal chelation by phenolic and flavonoid compounds [74,75]. Induction of phenolic concentration after Si application has been reported in Zea mays under Al stress by stimulating the metabolism of phenolic compounds [76].
In the present study, the levels of oxidative stress markers, i.e., MDA and H2O2, were found to be elevated in the case of As stress while application of Si and NO alone as well as in combination reduced the level of MDA and H2O2. Similarly, exogenous application of Si reduced the level of MDA and H2O2 in B. juncea [77] and also by the application of NO in Isatis cappadocica plants [19] under As stress. This Si and NO-mediated decline in the level of oxidative stress markers is due to ROS scavenging and reduced As accumulation [5]. Silicon is considered to maintain membrane integrity and permeability by lowering the end product of lipid peroxidation, i.e., MDA concentration [78].
Another study also revealed that application of Si reduced the Zn stress-induced lipid peroxidation in rice plants, indicating the role of Si in preventing structural and functional deterioration of cell membrane [79]. Electrolyte leakage, concentrations of methylglyoxal, MDA, and H2O2 were markedly lowered by the supplementation of Si in roots and leaves of mustard plants which might be attributed to the declined level of Ni and improved functioning of antioxidative enzymes, i.e., SOD, CAT, and APX [80]. Nitric oxide also plays an important role in lowering lipid peroxidation in plants as it shows a protective effect in decreasing As accumulation and also functions as a ROS scavenger to diminish oxidative stress [16]. Concentrations of H2O2 and MDA were elevated by As treatment in lettuce leaves; however, application of NO significantly attenuated oxidative stress by decreasing the concentration of these oxidative stress markers through suppression of As absorption and translocation [81].
Oxidative stress is caused by metals in plants through the overaccumulation of ROS [82,83]. Overaccumulation of ROS in plants causes damage to various biomolecules such as proteins, lipids, and nucleic acids [84]. Therefore, cellular ROS balance must be maintained by regulating their production and scavenging, which is maintained by the plant antioxidant defense system, i.e., antioxidative enzymes such as SOD, CAT, glutathione peroxidase (GPOX), POD, APX, GR, etc., and non-enzymatic antioxidants, i.e., ascorbate and glutathione [85,86]. CAT and APX are the two essential enzymes that play an important role in H2O2 detoxification. Moreover, the balance between the SOD and H2O2-scavenging enzymes is recognized as crucial in determining the accumulation of O2 and H2O2 [87]. The As-mediated decline in the CAT activity could be due to excessive formation of ROS, or alterations in the balance between enzymes, i.e., in APX and POD [88]. Our results showed that the application of Si and NO alone and in combination in As-treated radish plants increased the activities of all antioxidative enzymes. Silicon is known to reduce oxidative stress by reducing the production of ROS in plants by stimulating the enzymatic and non-enzymatic antioxidants. Stress caused by metals such as Pb, Mn, Zn, and Cu was alleviated by adding Si by triggering the activity of enzymatic antioxidants [89]. The addition of Si improved the flax genotypes’ tolerance to copper stress by stimulating the functioning of SOD, POD, APX, and CAT enzymes to scavenge overaccumulation of ROS in plant tissues [90]. Silicon-mediated mechanisms for metal detoxification involve immobilization of metals (at soil level), diminishing ROS levels by improving the antioxidant system, compartmentation of metals in plant tissues, chelation, regulation of gene expression, and structural modifications in various portions of plants (at plant level) [89]. Similar mechanisms could be involved in Si-mediated increase in the activities of various antioxidative enzymes in radish plants under As stress.
Exogenous as well as endogenous levels of NO play an imperative role in the mitigation of metal stress in plants [91]. The beneficial effects of NO on various antioxidative enzymes on various plant species under As stress and other metals have been well documented [92–94]. For example, As stress was significantly alleviated by the supplementation of NO in rice and mung beans by lowering the ROS level and MDA concentration [16,95]. Cadmium-mediated oxidative stress and lipid peroxidation were lowered by the exogenous application of SNP by performing ROS scavenging mechanisms and by stimulating the functioning of antioxidative enzymes [96]. The activity of antioxidative enzymes, i.e., SOD, APX, GR, and DHAR, were increased by the application of NO to inhibit the silver nanoparticles (Ag NPs) induced oxidative damage [97]. The antioxidant potential of Oryza sativa plants was increased by SNP application through elevation in the activity of peroxidase (POD) and CAT under Ni stress [98].
Heavy metal-mediated oxidative stress in plants can be reduced not only by antioxidative enzymes but also by non-enzymatic antioxidants. Non-toxic complexes are produced by glutathione to protect plant tissues from metal-mediated phytotoxicity [99]. Moreover, regulation of redox balance and mitigation of abiotic stresses is carried out by the presence of glutathione in plant organelles via functioning as a buffer [100]. The results of the current study showed that the levels of glutathione and ascorbic acid, were found to be enhanced by the individual as well as combined application of Si and NO against As toxicity. Similarly, application of NO increased the concentration of non-enzymatic antioxidants under Cd and Cu stress in Typha angustifolia [101] and in periwinkle roots, respectively [102]. Glutathione alleviates As toxicity in plants by regulating the As binding phytochelatins (PCs) [17]. Silicon in combination with As also showed enhanced accumulation of non-enzymatic antioxidants as presented by Geng et al. [103] in rice, Sil et al. [104] in wheat, Tripathi et al. [105] in rice, and González-Moscoso et al. [106] in tomato.
In the present study, As stress caused the reduction in plant growth and biomass by causing oxidative stress in plants. However, individual as well as combined application of Si and NO proved to be significant in alleviating As stress in radish plants by regulating plant growth and the plant antioxidant defense system via the ROS scavenging mechanism (Fig. 8). Therefore, foliar spraying with Si or pretreatment with NO, particularly in combination, can be suggested as an inexpensive, eco-friendly, and efficient technique to mitigate As-mediated toxicity in radish on a commercial scale. Further studies should be focused on Si- and NO-mediated mechanisms at molecular level in order to understand the expression of various defense-related genes under As stress.
Figure 8: Silicon and nitric oxide-mediated mechanism of As stress tolerance in radish plants
Author Contribution: SB and DK designed the study. SB performed the experiments, analyzed the data, and wrote the manuscript. SB, TV, AR and DK reviewed and edited the manuscript. All authors reviewed the results and approved the final version of the manuscript.
Acknowledgement: Authors are thankful to Lovely Professional University, Punjab for providing all the laboratory facilities to carry out the present study.
Funding Statement: The authors received no specific funding for this study.
Conflicts of Interest: The authors declare that they have no conflicts of interest to report regarding the present study.
References
1. Solórzano, E., Corpas, F. J., González-Gordo, S., Palma, J. M. (2020). Reactive oxygen species (ROS) metabolism and nitric oxide (NO) content in roots and shoots of rice (Oryza sativa L.) plants under arsenic-induced stress. Agronomy, 10(7), 1014. DOI 10.3390/agronomy10071014. [Google Scholar] [CrossRef]
2. Raza, A., Tabassum, J., Zahid, Z., Charagh, S., Bashir, S. et al. (2022). Advances in “Omics” approaches for improving toxic metals/metalloids tolerance in plants. Frontiers in Plant Science, 12, 794373. DOI 10.3389/fpls.2021.794373. [Google Scholar] [CrossRef]
3. Chikkanna, A., Mehan, L., Sarath, P. K., Ghosh, D. (2019). Arsenic exposures, poisoning, and threat to human health: Arsenic affecting human health. In: Papadopoulou, P., Marouli, C., Misseyanni, A. (Eds.Environmental exposures and human health challenges, pp. 86–105. USA: IGI Global. [Google Scholar]
4. Dai, Y., Nasir, M., Zhang, Y., Gao, J., Lv, Y. et al. (2018). Comparison of DGT with traditional extraction methods for assessing arsenic bioavailability to Brassica chinensis in different soils. Chemosphere, 191, 183–189. DOI 10.1016/j.chemosphere.2017.10.035. [Google Scholar] [CrossRef]
5. Ahmad, A., Khan, W. U., Shah, A. A., Yasin, N. A., Naz, S. et al. (2021). Synergistic effects of nitric oxide and silicon on promoting plant growth, oxidative stress tolerance and reduction of arsenic uptake in Brassica juncea. Chemosphere, 262(1), 128384. DOI 10.1016/j.chemosphere.2020.128384. [Google Scholar] [CrossRef]
6. Abedi, T., Mojiri, A. (2020). Arsenic uptake and accumulation mechanisms in rice species. Plants, 9(2), 129. DOI 10.3390/plants9020129. [Google Scholar] [CrossRef]
7. Rosas-Castor, J. M., Guzmán-Mar, J. L., Hernández-Ramírez, A., Garza-González, M. T., Hinojosa-Reyes, L. (2014). Arsenic accumulation in maize crop (Zea maysA review. Science of the Total Environment, 488, 176–187. DOI 10.1016/j.scitotenv.2014.04.075. [Google Scholar] [CrossRef]
8. Singh, R., Upadhyay, A. K., Singh, D. P. (2018). Regulation of oxidative stress and mineral nutrient status by selenium in arsenic treated crop plant Oryza sativa. Ecotoxicology and Environmental Safety, 148(7), 105–113. DOI 10.1016/j.ecoenv.2017.10.008. [Google Scholar] [CrossRef]
9. Domingos, P., Prado, A. M., Wong, A., Gehring, C., Feijo, J. A. (2015). Nitric oxide: A multitasked signaling gas in plants. Molecular Plant, 8(4), 506–520. DOI 10.1016/j.molp.2014.12.010. [Google Scholar] [CrossRef]
10. Iqbal, N., Umar, S., Khan, N. A., Corpas, F. J. (2021). Nitric oxide and hydrogen sulfide coordinately reduce glucose sensitivity and decrease oxidative stress via ascorbate-glutathione cycle in heat-stressed wheat (Triticum aestivum L.) plants. Antioxidants, 10(1), 108. DOI 10.3390/antiox10010108. [Google Scholar] [CrossRef]
11. Bhat, J. A., Ahmad, P., Corpas, F. J. (2021). Main nitric oxide (NO) hallmarks to relieve arsenic stress in higher plants. Journal of Hazardous Materials, 406(1), 124289. DOI 10.1016/j.jhazmat.2020.124289. [Google Scholar] [CrossRef]
12. Emamverdian, A., Ding, Y., Barker, J., Mokhberdoran, F., Ramakrishnan, M. et al. (2021). Nitric oxide ameliorates plant metal toxicity by increasing antioxidant capacity and reducing Pb and Cd translocation. Antioxidants, 10(12), 1981. [Google Scholar]
13. Zheng, C., Jiang, D., Liu, F., Dai, T., Liu, W. et al. (2009). Exogenous nitric oxide improves seed germination in wheat against mitochondrial oxidative damage induced by high salinity. Environmental and Experimental Botany, 67(1), 222–227. [Google Scholar]
14. Groß, F., Durner, J., Gaupels, F. (2013). Nitric oxide, antioxidants and prooxidants in plant defence responses. Frontiers in Plant Science, 4, 419. [Google Scholar]
15. Sun, C., Lu, L., Liu, L., Liu, W., Yu, Y. et al. (2014). Nitrate reductase-mediated early nitric oxide burst alleviates oxidative damage induced by aluminum through enhancement of antioxidant defenses in roots of wheat (Triticum aestivum). New Phytologist, 201(4), 1240–1250. [Google Scholar]
16. Singh, H. P., Kaur, S., Batish, D. R., Sharma, V. P., Sharma, N. et al. (2009). Nitric oxide alleviates arsenic toxicity by reducing oxidative damage in the roots of Oryza sativa (rice). Nitric Oxide, 20, 289–297. [Google Scholar]
17. Souri, Z., Karimi, N., Sandalio, L. M. (2017). Arsenic hyperaccumulation strategies: An overview. Frontiers in Cell and Developmental Biology, 5, 67. DOI 10.3389/fcell.2017.00067. [Google Scholar] [CrossRef]
18. Terrón-Camero, L. C., Peláez-Vico, M. Á., Del-Val, C., Sandalio, L. M., Romero-Puertas, M. C. (2019). Role of nitric oxide in plant responses to heavy metal stress: Exogenous application versus endogenous production. Journal of Experimental Botany, 70(17), 4477–4488. DOI 10.1093/jxb/erz184. [Google Scholar] [CrossRef]
19. Souri, Z., Karimi, N., Farooq, M. A., Sandalio, L. M. (2020). Nitric oxide improves tolerance to arsenic stress in Isatis cappadocica desv. Shoots by enhancing antioxidant defenses. Chemosphere, 239(6), 124523. DOI 10.1016/j.chemosphere.2019.124523. [Google Scholar] [CrossRef]
20. Abbas, G., Murtaza, B., Bibi, I., Shahid, M., Niazi, N. K. et al. (2018). Arsenic uptake, toxicity, detoxification, and speciation in plants: Physiological, biochemical, and molecular aspects. International journal of Environmental Research and Public Health, 15(1), 59. DOI 10.3390/ijerph15010059. [Google Scholar] [CrossRef]
21. Huang, H., Li, M., Rizwan, M., Dai, Z., Yuan, Y. et al. (2021). Synergistic effect of silicon and selenium on the alleviation of cadmium toxicity in rice plants. Journal of Hazardous Materials, 401(6), 123393. DOI 10.1016/j.jhazmat.2020.123393. [Google Scholar] [CrossRef]
22. Mir, R. A., Bhat, B. A., Yousuf, H., Islam, S. T., Raza, A. et al. (2022). Multidimensional role of silicon to activate resilient plant growth and to mitigate abiotic stress. Frontiers in Plant Science, 13, 819658. DOI 10.3389/fpls.2022.819658. [Google Scholar] [CrossRef]
23. Mundada, P. S., Barvkar, V. T., Umdale, S. D., Kumar, S. A., Nikam, T. D. et al. (2021). An insight into the role of silicon on retaliation to osmotic stress in finger millet (Eleusine coracana (L.) Gaertn). Journal of Hazardous Materials, 403(2), 124078. DOI 10.1016/j.jhazmat.2020.124078. [Google Scholar] [CrossRef]
24. Bhardwaj, S., Kapoor, D. (2021). Fascinating regulatory mechanism of silicon for alleviating drought stress in plants. Plant Physiology and Biochemistry, 166(6), 1044–1053. DOI 10.1016/j.plaphy.2021.07.005. [Google Scholar] [CrossRef]
25. Bakhat, H. F., Bibi, N., Zia, Z., Abbas, S., Hammad, H. M. et al. (2018). Silicon mitigates biotic stresses in crop plants: A review. Crop Protection, 104(7), 21–34. DOI 10.1016/j.cropro.2017.10.008. [Google Scholar] [CrossRef]
26. Khan, A., Bilal, S., Khan, A. L., Imran, M., Al-Harrasi, A. et al. (2020). Silicon-mediated alleviation of combined salinity and cadmium stress in date palm (Phoenix dactylifera L.) by regulating physio-hormonal alteration. Ecotoxicology and Environmental Safety, 188, 109885. DOI 10.1016/j.ecoenv.2019.109885. [Google Scholar] [CrossRef]
27. Tripathi, D. K., Singh, V. P., Prasad, S. M., Chauhan, D. K., Dubey, N. K. et al. (2015). Silicon-mediated alleviation of Cr(VI) toxicity in wheat seedlings as evidenced by chlorophyll florescence, laser induced breakdown spectroscopy and anatomical changes. Ecotoxicology and Environmental Safety, 113(7), 133–144. DOI 10.1016/j.ecoenv.2014.09.029. [Google Scholar] [CrossRef]
28. Singh, S., Singh, V. P., Prasad, S. M., Sharma, S., Ramawat, N. et al. (2019). Interactive effect of silicon (Si) and salicylic acid (SA) in maize seedlings and their mechanisms of cadmium (Cd) toxicity alleviation. Journal of Plant Growth Regulation, 38(4), 1587–1597. DOI 10.1007/s00344-019-09958-1. [Google Scholar] [CrossRef]
29. Bhat, J. A., Shivaraj, S. M., Singh, P., Navadagi, D. B., Tripathi, D. K. et al. (2019). Role of silicon in mitigation of heavy metal stresses in crop plants. Plants, 8(3), 71. DOI 10.3390/plants8030071. [Google Scholar] [CrossRef]
30. Vaculík, M., Kováč, J., Fialová, I., Fiala, R., Jašková, K. et al. (2021). Multiple effects of silicon on alleviation of nickel toxicity in young maize roots. Journal of Hazardous Materials, 415, 125570. DOI 10.1016/j.jhazmat.2021.125570. [Google Scholar] [CrossRef]
31. Vaculík, M., Lukačová, Z., Bokor, B., Martinka, M., Tripathi, D. K. et al. (2020). Alleviation mechanisms of metal (loid) stress in plants by silicon: A review. Journal of Experimental Botany, 71(21), 6744–6757. DOI 10.1093/jxb/eraa288. [Google Scholar] [CrossRef]
32. Schutzendubel, A., Polle, A. (2002). Plant responses to abiotic stresses: Heavy metal-induced oxidative stress and protection by mycorrhization. Journal of Experimental Botany, 53(372), 1351–1365. [Google Scholar]
33. Sharma, A., Shahzad, B., Rehman, A., Bhardwaj, R., Landi, M. et al. (2019). Response of phenylpropanoid pathway and the role of polyphenols in plants under abiotic stress. Molecules, 24(13), 2452. [Google Scholar]
34. Banihani, S. A. (2017). Radish (Raphanus sativus) and diabetes. Nutrients, 9(9), 1014. [Google Scholar]
35. Smith, P. G., Koch, I., Reimer, K. J. (2008). Uptake, transport and transformation of arsenate in radishes (Raphanus sativus). Science of the Total Environment, 390(1), 188–197. [Google Scholar]
36. Auobi Amirabad, S., Behtash, F., Vafaee, Y. (2020). Selenium mitigates cadmium toxicity by preventing oxidative stress and enhancing photosynthesis and micronutrient availability on radish (Raphanus sativus L.) cv. Cherry Belle. Environmental Science and Pollution Research, 27(11), 12476–12490. [Google Scholar]
37. Kapoor, D., Rattan, A., Gautam, V., Bhardwaj, R. (2016). Alleviation of cadmium and mercury stress by supplementation of steroid hormone to Raphanus sativus seedlings. Proceedings of the National Academy of Sciences, India Section B: Biological Sciences, 86(3), 661–666. [Google Scholar]
38. Biteur, N., Aoues, A., Kharoubi, O., Slimani, M. (2011). Oxidative stress induction by lead in leaves of radish (Raphanus sativus) seedlings. Notulae Scientia Biologicae, 3(4), 93–99. [Google Scholar]
39. Ramakrishna, B., Rao, S. S. R. (2015). Foliar application of brassinosteroids alleviates adverse effects of zinc toxicity in radish (Raphanus sativus L.) plants. Protoplasma, 252(2), 665–677. [Google Scholar]
40. Bali, A. S., Sidhu, G. P. S. (2021). Arsenic acquisition, toxicity and tolerance in plants-from physiology to remediation: A review. Chemosphere, 283, 131050. [Google Scholar]
41. Liu, X., Yin, L., Deng, X., Gong, D., Du, S. et al. (2020). Combined application of silicon and nitric oxide jointly alleviated cadmium accumulation and toxicity in maize. Journal of Hazardous Materials, 395, 122679. [Google Scholar]
42. Singh, S., Prasad, S. M., Sharma, S., Dubey, N. K., Ramawat, N. et al. (2020). Silicon and nitric oxide-mediated mechanisms of cadmium toxicity alleviation in wheat seedlings. Physiologia Plantarum, 168(1), 1–28. [Google Scholar]
43. Heath, R. L., Packer, L. (1968). Photoperoxidation in isolated chloroplasts: I. Kinetics and stoichiometry of fatty acid peroxidation. Archives of Biochemistry and Biophysics, 125(1), 189–198. [Google Scholar]
44. Velikova, V., Yordanov, I., Edreva, A. (2000). Oxidative stress and some antioxidant system in acid rain treated bean plants: Protective role of exogenous polyamines. Plant Science, 151, 59–66. [Google Scholar]
45. Kim, M. S., Kim, C., Jo, D. H., Ryu, Y. W. (1999). Effect of fungal elicitor and heavy metals on the production of flavonol glycosides in the cell cultures of Ginko biloba. Journal Micrbiology Biotechnology, 9, 661–667. [Google Scholar]
46. Malick, C. P., Singh, M. B. (1980). Phenolics, plant enzymology and histoenzymology. New Delhi: Kalyani Publishers. [Google Scholar]
47. Mancinelli, A. L. (1984). Photoregulation of anthocyanin synthesis: Effect of light pretreatments. Plant Physiology, 75(2), 447–453. [Google Scholar]
48. Lowry, O. H. (1951). Measurement with the folin phenol reagent. Journal of Biological Chemistry, 193, 265–275. [Google Scholar]
49. Aebi, H. (1983). Catalase. In: Bergmeyer, H. U. (Ed.Methods of enzymatic analysis, pp. 673–684. New York: Verlag Chemie. [Google Scholar]
50. Nakano, Y., Asada, K. (1981). Hydrogen peroxide is scavenged by ascorbate-specific peroxidase in spinach chloroplasts. Plant Cell and Physiology, 22(5), 867–880. [Google Scholar]
51. Putter, J. (1974). Peroxidase. In: Bergmeyer, H. U. (Ed.Methods of enzymatic analysis, vol. 2, pp. 685–690. New York: Verlag Chemie. [Google Scholar]
52. Kumar, K. B., Khan, P. A. (1982). Peroxidase and polyphenol oxidase in excised ragi (Eleusine coracana cv. PR 202) leaves during senescence. Indian Journal of Experimental Botany, 20, 412–416. [Google Scholar]
53. Roe, J. H., Kuether, C. A. (1943). The determination of ascorbic acid in whole blood and urine through the 2, 4-dinitrophenylhydrazine derivative of dehydroascorbic acid. The Journal of Biological Chemistry, 147, 399–407. [Google Scholar]
54. Sedlak, J., Lindsay, R. H. (1968). Estimation of total, protein-bound, and nonprotein sulfhydryl groups in tissue with Ellman’s reagent. Analytical Biochemistry, 25, 192–205. [Google Scholar]
55. Maghsoudi, K., Arvin, M. J., Ashraf, M. (2020). Mitigation of arsenic toxicity in wheat by the exogenously applied salicylic acid, 24-epi-brassinolide and silicon. Journal of Soil Science and Plant Nutrition, 20(2), 577–588. [Google Scholar]
56. Yang, Z., Chen, Z., He, N., Yang, D., Liu, M. (2022). Effects of silicon and iron application on arsenic absorption and physiological characteristics of rice (Oryza sativa L.). Bulletin of Environmental Contamination and Toxicology, 108(6), 1–10. [Google Scholar]
57. Zali, M. R., Lin, W., Zhan, W., Fang, C. (2020). The role of silicon to increase arsenic tolerance in rice (Oryza sativa L.) seedlings by reinforcing anti-oxidative defense. Bioagro, 32(3), 159–168. [Google Scholar]
58. Gill, R. A., Zang, L., Ali, B., Farooq, M. A., Cui, P. et al. (2015). Chromium-induced physio-chemical and ultrastructural changes in four cultivars of Brassica napus L. Chemosphere, 120, 154–164. [Google Scholar]
59. Amooaghaie, R., Tabatabaei, F., Ahadi, A. (2018). Alterations in HO-1 expression, heme oxygenase activity and endogenous NO homeostasis modulate antioxidant responses of Brassica nigra against nano silver toxicity. Journal of Plant Physiology, 228, 75–84. [Google Scholar]
60. Amooaghaie, R., Zangene-Madar, F., Enteshari, S. (2017). Role of twosided crosstalk between NO and H2S on improvement of mineral homeostasis and antioxidative defense in Sesamum indicum under lead stress. Ecotoxicology and Environmental Safety, 139(3), 210–218. DOI 10.1016/j.ecoenv.2017.01.037. [Google Scholar] [CrossRef]
61. Verma, K., Mehta, S. K., Shekhawat, G. S. (2013). Nitric oxide (NO) counteracts cadmium induced cytotoxic processes mediated by reactive oxygen species (ROS) in Brassica juncea: crosstalk between ROS, NO and antioxidant responses. Biometals, 26(2), 255–269. DOI 10.1007/s10534-013-9608-4. [Google Scholar] [CrossRef]
62. Wu, Z., Xu, S., Shi, H., Zhao, P., Liu, X. et al. (2018). Comparison of foliar silicon and selenium on cadmium absorption, compartmentation, translocation and the antioxidant system in Chinese fowering cabbage. Ecotoxicology and Environmental Safety, 166(4), 157–164. DOI 10.1016/j.ecoenv.2018.09.085. [Google Scholar] [CrossRef]
63. Ashfaque, F., Inam, A., Iqbal, S., Sahay, S. (2017). Response of silicon on metal accumulation, photosynthetic inhibition and oxidative stress in chromium-induced mustard (Brassica juncea L.). South African Journal of Botany, 111, 153–160. DOI 10.1016/j.sajb.2017.03.002. [Google Scholar] [CrossRef]
64. Saleem, M. H., Parveen, A., Khan, S. U., Hussain, I., Wang, X. et al. (2022). Silicon fertigation regimes attenuates cadmium toxicity and phytoremediation potential in two maize (Zea mays L.) cultivars by minimizing its uptake and oxidative stress. Sustainability, 14(3), 1462. DOI 10.3390/su14031462. [Google Scholar] [CrossRef]
65. Keller, C., Rizwan, M., Davidian, J. C., Pokrovsky, O. S., Bovet, N. et al. (2015). Effect of silicon on wheat seedlings (Triticum turgidum L.) grown in hydroponics and exposed to 0 to 30 μM Cu. Planta, 241(4), 847–860. [Google Scholar]
66. Khaliq, A., Ali, S., Hameed, A., Farooq, M. A., Farid, M. et al. (2016). Silicon alleviates nickel toxicity in cotton seedlings through enhancing growth, photosynthesis, and suppressing Ni uptake and oxidative stress. Archives of Agronomy and Soil Science, 62(5), 633–647. [Google Scholar]
67. Luyckx, M., Hausman, J. F., Lutts, S., Guerriero, G. (2017). Impact of silicon in plant biomass production: Focus on bast fibres, hypotheses, and perspectives. Plants, 6(3), 37. [Google Scholar]
68. Singh, A. P., Dixit, G., Kumar, A., Mishra, S., Singh, P. K. et al. (2016a). Nitric oxide alleviated arsenic toxicity by modulation of antioxidants and thiol metabolism in rice (Oryza sativa L.). Frontiers in Plant Science, 6, 1272. [Google Scholar]
69. Rezayian, M., Ebrahimzadeh, H., Niknam, V. (2020). Nitric oxide stimulates antioxidant system and osmotic adjustment in soybean under drought stress. Journal of Soil Science and Plant Nutrition, 20(3), 1122–1132. [Google Scholar]
70. Ren, S. C., Sun, J. T. (2014). Changes in phenolic content, phenylalanine ammonia-lyase (PAL) activity, and antioxidant capacity of two buckwheat sprouts in relation to germination. Journal of Functional Foods, 7(1), 298–304. DOI 10.1016/j.jff.2014.01.031. [Google Scholar] [CrossRef]
71. Pirooz, P., Amooaghaie, R., Ahadi, A., Sharififar, F., Torkzadeh-Mahani, M. (2022). Silicon and nitric oxide synergistically modulate the production of essential oil and rosmarinic acid in Salvia officinalis under Cu stress. Protoplasma, 259(4), 905–916. DOI 10.1007/s00709-021-01708-z. [Google Scholar] [CrossRef]
72. Dar, F. A., Tahir, I., Hakeem, K. R., Rehman, R. U. (2022). Silicon application enhances the photosynthetic pigments and phenolic/flavonoid content by modulating the phenylpropanoid pathway in common buckwheat under aluminium stress. Silicon, 14(1), 323–334. DOI 10.1007/s12633-021-01501-w. [Google Scholar] [CrossRef]
73. Fatemi, H., Esmaiel Pour, B., Rizwan, M. (2021). Foliar application of silicon nanoparticles affected the growth, vitamin C, flavonoid, and antioxidant enzyme activities of coriander (Coriandrum sativum L.) plants grown in lead (Pb)-spiked soil. Environmental Science and Pollution Research, 28(2), 1417–1425. DOI 10.1007/s11356-020-10549-x. [Google Scholar] [CrossRef]
74. Collin, B., Doelsch, E., Keller, C., Cazevieille, P., Tella, M. et al. (2014). Evidence of sulfur-bound reduced copper in bamboo exposed to high silicon and copper concentrations. Environmental Pollution, 187, 22–30. DOI 10.1016/j.envpol.2013.12.024. [Google Scholar] [CrossRef]
75. Wang, Y., Stass, A., Horst, W. J. (2004). Apoplastic binding of aluminum is involved in silicon-induced amelioration of aluminum toxicity in maize. Plant Physiology, 136, 3762–3770. [Google Scholar]
76. Kidd, P. S., Llugany, M., Poschenrieder, C. H., Gunse, B., Barcelo, J. (2001). The role of root exudates in aluminium resistance and silicon-induced amelioration of aluminium toxicity in three varieties of maize (Zea mays L.). Journal of Experimental Botany, 52(359), 1339–1352. [Google Scholar]
77. Praveen, A., Pandey, C., Ehasanullah, K. H. A. N., Panthri, M., Gupta, M. (2020). Silicon-mediated genotoxic alterations in Brassica juncea under arsenic stress: A comparative study of biochemical and molecular markers. Pedosphere, 30(4), 517–527. [Google Scholar]
78. Moussa, H. R. (2006). Influence of exogenous application of silicon on physiological response of salt-stressed maize (Zea mays L.). International Journal of Agriculture and Biology, 8(3), 293–297. [Google Scholar]
79. Song, A., Li, P., Li, Z., Fan, F., Nikolic, M. et al. (2011). The alleviation of zinc toxicity by silicon is related to zinc transport and antioxidative reactions in rice. Plant and Soil, 344(1), 319–333. [Google Scholar]
80. Abd_Allah, E. F., Hashem, A., Alam, P., Ahmad, P. (2019). Silicon alleviates nickel-induced oxidative stress by regulating antioxidant defense and glyoxalase systems in mustard plants. Journal of Plant Growth Regulation, 38(4), 1260–1273. DOI 10.1007/s00344-019-09931-y. [Google Scholar] [CrossRef]
81. Silveira, N. M., de Oliveira, J. A., Ribeiro, C., Canatto, R. A., Siman, L. et al. (2015). Nitric oxide attenuates oxidative stress induced by arsenic in lettuce (Lactuca sativa) leaves. Water, Air, and Soil Pollution, 226(11), 379. DOI 10.1007/s11270-015-2630-0. [Google Scholar] [CrossRef]
82. Raza, A., Salehi, H., Rahman, M. A., Zahid, Z., Madadkar, H. M. et al. (2022). Plant hormones and neurotransmitter interactions mediate antioxidant defenses under induced oxidative stress in plants. Frontiers in Plant Science, 13, 961872. DOI 10.3389/fpls.2022.961872. [Google Scholar] [CrossRef]
83. Berni, R., Luyckx, M., Xu, X., Legay, S., Sergeant, K. et al. (2019). Reactive oxygen species and heavy metal stress in plants: Impact on the cell wall and secondary metabolism. Environmental and Experimental Botany, 161(1), 98–106. DOI 10.1016/j.envexpbot.2018.10.017. [Google Scholar] [CrossRef]
84. Mittler, R. (2017). ROS are good. Trends in Plant Science, 22(1), 11–19. DOI 10.1016/j.tplants.2016.08.002. [Google Scholar] [CrossRef]
85. Gill, S. S., Tuteja, N. (2010). Reactive oxygen species and antioxidant machinery in abiotic stress tolerance in crop plants. Plant Physiology and Biochemistry, 48(12), 909–930. [Google Scholar]
86. Nadgórska-Socha, A., Kafel, A., Kandziora-Ciupa, M., Gospodarek, J., Zawisza Raszka, A. (2013). Accumulation of heavy metals and antioxidant responses in Vicia faba plants grown on monometallic contaminated soil. Environmental Science and Pollution Research, 20, 1124–1134. [Google Scholar]
87. Sofy, A. R., Dawoud, R. A., Sofy, M. R., Mohamed, H. I., Hmed, A. A. et al. (2020). Improving regulation of enzymatic and non-enzymatic antioxidants and stress-related gene stimulation in Cucumber mosaic cucumovirus-infected cucumber plants treated with glycine betaine, chitosan and combination. Molecules, 25, 2341. [Google Scholar]
88. Apel, K., Hirt, H. (2004). Reactive oxygen species: Metabolism, oxidative stress, and signaling transduction. Annual Review of Plant Biology, 55, 373. [Google Scholar]
89. Fu, Y. Q., Shen, H., Wu, D. M., Cai, K. Z. (2012). Silicon-mediated amelioration of Fe2+ toxicity in rice Oryza sativa L. roots. Pedosphere, 22, 795–802. [Google Scholar]
90. El-Beltagi, H. S., Sofy, M. R., Aldaej, M. I., Mohamed, H. I. (2020). Silicon alleviates copper toxicity in flax plants by up-regulating antioxidant defense and secondary metabolites and decreasing oxidative damage. Sustainability, 12(11), 4732. DOI 10.3390/su12114732. [Google Scholar] [CrossRef]
91. Nabi, R. B. S., Tayade, R., Hussain, A., Kulkarni, K. P., Imran, Q. M. et al. (2019). Nitric oxide regulates plant responses to drought, salinity, and heavy metal stress. Environmental and Experimental Botany, 161, 120–133. DOI 10.1016/j.envexpbot.2019.02.003. [Google Scholar] [CrossRef]
92. Singh, V. P., Srivastava, P. K., Prasad, S. M. (2013). Nitric oxide alleviates arsenic-induced toxic effects in ridged Luffa seedlings. Plant Physiology and Biochemistry, 71, 155–163. DOI 10.1016/j.plaphy.2013.07.003. [Google Scholar] [CrossRef]
93. Talukdar, D. (2013). Arsenic-induced oxidative stress in the common bean legume, Phaseolus vulgaris L. seedlings and its amelioration by exogenous nitric oxide. Physiology and Molecular Biology of Plants, 19(1), 69–79. DOI 10.1007/s12298-012-0140-8. [Google Scholar] [CrossRef]
94. Shukla, P., Singh, S., Dubey, P., Singh, A., Singh, A. K. (2015). Nitric oxide mediated amelioration of arsenic toxicity which alters the alternative oxidase (Aox1) gene expression in Hordeum vulgare L. Ecotoxicology and Environmental Safety, 120, 59–65. DOI 10.1016/j.ecoenv.2015.05.030. [Google Scholar] [CrossRef]
95. Ismail, G. S. M. (2012). Protective role of nitric oxide against arsenic-induced damages in germinating mung bean seeds. Acta Physiologiae Plantarum, 34(4), 1303–1311. DOI 10.1007/s11738-012-0927-9. [Google Scholar] [CrossRef]
96. Wang, Y., Loake, G. J., Chu, C. (2013). Cross-talk of nitric oxide and reactive oxygen species in plant programed cell death. Frontiers in Plant Science, 4, 1–7. DOI 10.3389/fpls.2013.00314. [Google Scholar] [CrossRef]
97. Tripathi, D. K., Singh, S., Singh, S., Srivastava, P. K., Singh, V. P. et al. (2017). Nitric oxide alleviates silver nanoparticles (AgNps)-induced phytotoxicity in Pisum sativum seedlings. Plant Physiology and Biochemistry, 110(12), 167–177. DOI 10.1016/j.plaphy.2016.06.015. [Google Scholar] [CrossRef]
98. Rizwan, M., Mostofa, M. G., Ahmad, M. Z., Imtiaz, M., Mehmood, S. et al. (2018). Nitric oxide induces rice tolerance to excessive nickel by regulating nickel uptake, reactive oxygen species detoxification and defense-related gene expression. Chemosphere, 191(1), 23–35. DOI 10.1016/j.chemosphere.2017.09.068. [Google Scholar] [CrossRef]
99. Jozefczak, M., Remans, T., Vangronsveld, J., Cuypers, A. (2012). Glutathione is a key player in metal-induced oxidative stress defenses. International Journal of Molecular Sciences, 13(3), 3145–3175. DOI 10.3390/ijms13033145. [Google Scholar] [CrossRef]
100. Hasan, M. K., Liu, C., Wang, F., Ahammed, G. J., Zhou, J. et al. (2016). Glutathione-mediated regulation of nitric oxide, S-nitrosothiol and redox homeostasis confers cadmium tolerance by inducing transcription factors and stress response genes in tomato. Chemosphere, 161, 536–545. [Google Scholar]
101. Zhao, H., Jin, Q., Wang, Y., Chu, L., Li, X. et al. (2016). Effects of nitric oxide on alleviating cadmium stress in Typha angustifolia. Plant Growth Regulation, 78, 243–251. [Google Scholar]
102. Liu, S., Yang, R., Pan, Y., Ren, B., Chen, Q. et al. (2016). Beneficial behavior of nitric oxide in copper-treated medicinal plants. Journal of Hazardous Materials, 314, 140–154. [Google Scholar]
103. Geng, A., Wang, X., Wu, L., Wang, F., Wu, Z. et al. (2018). Silicon improves growth and alleviates oxidative stress in rice seedlings (Oryza sativa L.) by strengthening antioxidant defense and enhancing protein metabolism under arsanilic acid exposure. Ecotoxicology and Environmental Safety, 158, 266–273. [Google Scholar]
104. Sil, P., Biswas, A. K. (2020). Silicon nutrition modulates arsenic-inflicted oxidative overload and thiol metabolism in wheat (Triticum aestivum L.) seedlings. Environmental Science and Pollution Research, 27(36), 45209–45224. [Google Scholar]
105. Tripathi, P., Tripathi, R. D., Singh, R. P., Dwivedi, S., Goutam, D. et al. (2013). Silicon mediates arsenic tolerance in rice (Oryza sativa L.) through lowering of arsenic uptake and improved antioxidant defence system. Ecological Engineering, 52, 96–103. DOI 10.1016/j.ecoleng.2012.12.057. [Google Scholar] [CrossRef]
106. González-Moscoso, M., Martínez-Villegas, N. V., Cadenas-Pliego, G., Benavides-Mendoza, A., Rivera-Cruz, M. D. C. et al. (2019). Impact of silicon nanoparticles on the antioxidant compounds of tomato fruits stressed by arsenic. Foods, 8(12), 612. DOI 10.3390/foods8120612. [Google Scholar] [CrossRef]
Cite This Article
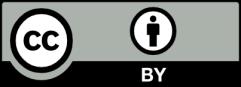
This work is licensed under a Creative Commons Attribution 4.0 International License , which permits unrestricted use, distribution, and reproduction in any medium, provided the original work is properly cited.