Open Access
ARTICLE
Functional Analysis of the Genotypic Differences in Response of Pea (Pisum sativum L.) to Calcareous-Induced Iron Deficiency
1 Laboratory of Ecosystems and Biodiversity in Arid Land of Tunisia, Faculty of Sciences, University of Sfax, Sfax, 3000, Tunisia
2 Centre of Biotechnology of Borj Cedria, Hammam Lif, 2050, Tunisia
3 Faculty of Sciences and Techniques, University of Kairouan, Kairouan, 9100, Tunisia
* Corresponding Author: Abdelmajid Krouma. Email:
(This article belongs to the Special Issue: Plant Ecophysiology: Recent Trends and Advancements)
Phyton-International Journal of Experimental Botany 2023, 92(2), 521-536. https://doi.org/10.32604/phyton.2022.023555
Received 01 May 2022; Accepted 16 June 2022; Issue published 12 October 2022
Abstract
Lime-induced iron chlorosis is a major nutritional disorder causing severe plant growth and yield reduction in the calcareous soils of Tunisia. The understanding the behavior of key metabolic functions of peas on calcareous soils, the identification of useful traits of tolerance, and the exploration of the genotypic differences in response to this constraint remain the most efficient approaches due to their coast, environmental benefits, and sustainability. For this purpose, a greenhouse experiment was conducted on three pea genotypes (Alexandra: Alex, Douce de provence: DP, and Merveille de Kelvedon: MK) cultivated on calcareous soil (Fe-deficient) and fertile soil (control). Plant growth, SPAD index, iron nutrition and distribution, photosynthesis, and antioxidant enzymes were deeply analyzed to discriminate genotypic differences. Calcareous-induced iron deficiency reduced SPAD index, plant growth, net photosynthesis, and tissue Fe content against a significant stimulation of the oxidative stress indicators, H2O2 and Malondialdehyde (MDA). Moreover, we reported a significant induction of SOD and CAT activity in shoots and roots of the Alexandra genotype. Fe use efficiency increased on calcareous soil and clearly discriminated the studied genotypes. Alexandra genotype was found to be the most tolerant to lime-induced iron chlorosis. This genotype protects its tissues against oxidative stress by stimulating enzyme activities (SOD and CAT) and develops significant efficiency of Fe uptake, translocation to shoots and use when cultivated on calcareous soil.Keywords
Nomenclature
CAT | catalase |
CS | calcareous soil |
FeT | Fe translocation |
FeUE-An | Fe use efficiency for photosynthesis |
FeUE-SOD-Sh | Fe use efficiency for SOD activity in shoots |
FeUE-SOD-R | Fe use efficiency for SOD activity in roots |
FeUE-CAT-Sh | Fe use efficiency for CAT activity in shoots |
FeUE-CAT-R | Fe use efficiency for CAT activity in roots |
FS | fertile soil |
MDA | malondialdehyde |
MTT | 3-(4,5-dimethylthiazol-2-yl)-2,5-diphenyltetrazolium bromide |
SOD | superoxide dismutase |
Iron chlorosis is the yellowing of plant leaves due to iron deficiency. The primary symptom is interveinal chlorosis, the development of a yellow leaf with a network of dark green veins. It affects many agricultural crops in the calcareous soils of Tunisia [1]. In these soils having bicarbonate, high pH, and ferric form of iron, Fe deficiency is a major nutritional disorder for plants [1]. These physicochemical conditions of soil affect the bioavailability of Fe and hamper plant growth and yield through the inhibition of Fe-dependent functions such as chlorophyll biosynthesis, photosynthesis, respiration, and protein formation [1]. Ammari et al. [2] indicated that measured Fe concentrations in calcareous soils exceed from a way the plant’s demand and mentioned that 50%–90% of this Fe content was complexed by organic molecules.
The physiological role of iron (Fe) in plants is well documented. Its main functions concern respiration, the synthesis of chlorophyll and photosynthesis [3]. The first consequence of limited iron availability is the lack of chlorophyll, followed by young leaf yellowing [1]. The function of various cytochromes containing heme Fe in the electron transport of photosynthesis is well established, as well as the role of ferredoxin. In fact, the photosynthetic electron transport chain requires three forms of Fe as protein cofactors (heme, nonheme, and Fe-S clusters [4]). The highest demand is for Fe-S clusters, with Photosystem I (PSI) subunits requiring three 4Fe-4S clusters, each Rieske subunit of the Cytochrome-b6f (Cyt-b6f) complex requiring a 2Fe-2S cluster and, Ferredoxin (Fd) requiring a 2Fe-2S cluster [3], the Cyt-b6f complex also contains multiple heme cofactors for electron transport and exists as a dimer, for a total of 12 Fe atoms spanning the subunits [5]. Photosystem II (PSII) requires one nonheme Fe cofactor, but, unlike Fe in the rest of the photosynthetic electron transport chain, it is unlikely that this cofactor is involved in electron transport [4].
Due to its close relationship with some antioxidant enzymes (some of which contain Fe, either in heme, POD and CAT, or non-heme, Fe-SOD [6]), iron catalyzes the free radical generation through the Fenton reaction. Krouma et al. [7] demonstrated that a significant stimulation of POD activity was observed in the tolerant genotype of common bean subjected to iron deficiency. SOD and CAT activities were maintained at high levels. In another context, the high respiratory activity of plant cells can cause a problem of oxygen conversion to reactive forms such as hydrogen peroxide (H2O2), superoxide anion (O2.) and hydroxyl radical (HO.) which are highly destructive to lipid membranes. Iron deficiency induces oxidative stress and generates reactive oxygen species (ROS) in plants, thus causing cell membranes peroxidation [2,7,8]. In order to defend themselves against oxidants, plants have developed protective mechanisms including antioxidant enzymes such as GPX, CAT, SOD, and non-enzymatic antioxidant substances [9]. It has been suggested that activities of these enzymes could be used as biochemical indicators of Fe availability in plants [10]. Prity et al. [8] demonstrated that antioxidant defense either through metabolites or antioxidant enzymes are not efficient in counteracting oxidative damage in Fe-deprived sorghum. Despite all these nutritional problems observed in calcareous soils, differences among species, varieties, and genotypes in response to Fe deficiency have been reported. Indeed, Krouma [1] demonstrated that Alex showed high tolerance to Fe deprivation as compared to other genotypes. Important H-ATPase and Fe-chelate reductase activities are the main reasons for this tolerance [1].
In order to understand some physiological and biochemical attributes of pea plants in response to iron deficiency and identify useful traits of tolerance, three pea genotypes were cultivated on fertile soil (control) and calcareous soil (known as lime-induced iron deficiency). Plant growth, photosynthesis, iron nutrition, oxidative stress, and antioxidant activity were investigated in order to identify their relationships and explain the tolerance of some genotypes.
2.1 Plant Material and Experimental Design
Three pea genotypes were used; Alexandra (Alex), Douce de Provence (DP) and Merveille de Kelvedon (MK) largely cultivated in Tunisia and provided for us by the Ministry of Agriculture and Water Resources. These genotypes introduced in Tunisia from France are subscribed in the common catalogue of varieties of vegetable species and published in the Official Journal of the European Union (2009/C 248 A/01). A greenhouse was used to conduct experiments under natural light with 16 h photoperiod and a temperature of 25°C/17°C (±2°C, day/night), relative humidity about 75%. Uniform seeds were disinfected with 2% hypochlorite calcium solution and sown individually in 1 kg pots filled with a fine, moistered near field capacity and mixed fertile soil (FS) sampled in the region of Gatrana (Sidi Bouzid: Long = 9.64301650° Lat = 35.14827290° WGS84 (GPS): Long = 9.64301650° Lat = 35.14827290°) or calcareous soil (CS) sampled in the region of Faiedh (sidi Bouzid: Long = 9.67560230° Lat = 35.07737120° WGS84 (GPS): Long = 9.67560230° Lat = 35.07737120°). The main soil characteristics are given in Table 1. At the beginning of flowering, 45 days after germination, non-destructive measurements (SPAD index and gas exchange) were made then plants were harvested and separated into shoots and roots. The last organs are soaked in 0.01 M CaCl2 solution and washed thoroughly and successively in 3 baths of ultra-pure water in order to avoid the contamination of roots with iron and elements from the soil [3]. Organs intended for biochemical analysis (MDA, proteins, enzymes) were harvested in liquid nitrogen and then stored at −80°C until use.
Relative leaf chlorophyll concentrations were estimated in vivo using a SPAD-502 (Konica-Minolta, Japan) prior to measurements of gas exchange, on the third fully expanded apical leaves. Measurements were made on ten plants of each soil and genotype. Values are presented as SPAD units.
The same third fully expanded apical leaves used for Spad index were used for gas exchange measurements using an LI-6400 (LI-COR, Inc., Lincoln, Nebraska, USA) portable gas exchange system. Ten plants of each genotype and soil were used. A saturating light of 1000 μmol m−2 s−1 was used to induce photosynthesis. This light was fitted to the standard 6 cm2 clamp on the leaf chamber [11]. The other parameters were maintained constant, sample pCO2 at 362 mbar, flow rate at 500 μmol s−1, and temperature at 25°C [11].
25 mg of fine powder of dry plant organ were shacked in 10 ml of 1N HCl for 24 h then filtered. Active iron (Fe2+) content was determined by the atomic absorption spectrophotometry method according to Köseoglu et al. [12].
Protein concentration was determined according to Bradford [13], using bovine serum albumin as a standard. Aliquots of frozen plant material (100 mg FW) were ground to a fine powder with liquid nitrogen and extracted at 4°C in 300 µL of 100 mM Tris HCl buffer (pH 8.0) containing 10 mM EDTA, 50 mM KCl, 20 mM MgCl2, 0.5 mM PMSF, 1 mM DTT, 0.1% (V/V) Triton X-100, and 10% (W/W) PVP. After centrifugation at 14,000 g, 30 min, 4°C, the supernatant was recovered for protein concentration and enzymes activity determination. Three replicates per treatment and genotype were used.
Total superoxide dismutase (SOD) activity was assayed according to Scebba et al. [14]. Increasing volumes (5, 10, 20, and 40 µl) of tissue crude extract were added to the reaction mixture at a final volume of 3 ml. The reaction mixture contained 50 mM potassium phosphate buffer (pH 7.8), 0.1 mM EDTA, 13 mM L-methionine, 2 µM riboflavin and 75 µM MTT (3-(4,5-dimethylthiazol-2-yl)-2,5- diphenyltetrazolium bromide). The reaction was started by exposing the mixture to cool white, fluorescent light at a photosynthetic photon flux of 50 µmol m−2 s−1 for 15 min. The developed blue colour in the reaction mixture was measured spectrophotometrically at 560 nm. The volume of sample causing 50% inhibition in colour development was taken as one unit of SOD activity [15] and the activity was presented as units per mg protein in each plant organ.
Catalase (CAT) activity was measured spectrophotometrically according to the method of Aebi [16], by monitoring the decline in the absorbance at 240 nm, as H2O2 was consumed. The final volume (3 mL) of the reaction mixture contained 50 mM sodium phosphate buffer (pH 7.0), to which 30 mM H2O2 (1 mL) was added (OD = 0.52–0.55 at 240 nm). The reaction was activated by adding 100 µL of the tissue extract to this solution. CAT activity was presented as units (mmol of H2O2 decomposed per min) per mg protein in each plant organ.
2.7 Malondialdehyde Concentration
Membrane lipid peroxidation was assessed by measuring the amount of malonyldialdehyde (MDA) in tissue as follow: Fresh tissues samples were homogenized in 0.1% (w/v) TCA solution. The homogenate was centrifuged at 15,000 g for 10 min. An aliquot of the supernatant was added to 0.5% TBA in 20% TCA. The mixture was heated at 90°C for 30 min in a shaking water bath, and then cooled in an ice bath. The samples were centrifuged at 10,000 g for 5 min, and the absorbance of the supernatant was read at 532 and 600 nm [17]. The MDA concentration was calculated as the difference of absorbance at
2.8 Hydrogen Peroxide (H2O2) Concentration
Hydrogen peroxide concentration of the plant tissue was determined using the method of Alexieva et al. [18]. A fresh tissues sample (0.1 g) was homogenized with 1 mL 0.1% (w/v) TCAA on ice. After centrifugation at 12,000 g for 15 min, 0.5 mL of the supernatant transferred to 0.5 mL of K-phosphate buffer (100 mM, pH 7.0) and 2.0 mL of 1 M potassium iodide (KI). The reaction was kept for 1 h in darkness and absorbance of incubation mixture measured at 390 nm. The amount of H2O2 was calculated based on a standard curve prepared with known concentrations of H2O2.
- FeUE-An: Fe use efficiency for photosynthesis, calculated as the ratio of net photosynthesis (µmol m−2 s−1) to Fe content in shoots (µg g−1 DW)
- FeUE-SOD-Sh: Fe use efficiency for SOD activity in shoots, calculated as the ratio of SOD activity in shoots (unit mg−1 protein) to Fe content in shoots (µg g−1 DW)
- FeUE-SOD-R: Fe use efficiency for SOD activity in roots, calculated as the ratio of SOD activity in roots (unit mg-1 protein) to Fe content in roots (µg g−1 DW)
- FeUE-CAT-Sh: Fe use efficiency for CAT activity in shoots, calculated as the ratio of CAT activity in shoots (unit mg-1 protein) to Fe content in shoots (µg g−1 DW)
- FeUE-CAT-R: Fe use efficiency for CAT activity in roots, calculated as the ratio of CAT activity in roots (unit mg−1 protein) to Fe content in roots (µg g−1 DW)
- FeT: Fe translocation calculated as the ratio shoot Fe quantity (µg) to total plant Fe quantity (µg) and expressed as a percentage
Data and statistical analyses were performed using the software StatPlus Pro. All data are presented as mean ± standard error. Analysis of variance (ANOVA) was performed to check whether effects of soil quality (FS and CS) on the respective factor were significant. The significance of differences among treatments was determined by Fisher’s least significant difference test (LSD) at 5%. Means were declared significantly different when the difference between any two treatments was greater than the LSD value generated from the ANOVA. They are marked by different letters in the figures.
3.1 Plant Growth, Chlorophyll and Gas Exchange
All plants cultivated on calcareous soil (CS) developed typical iron chlorosis on young leaves, more severe in MK than in DP and Alex (Fig. 1). Chlorophyll analysis estimated by SPAD index confirmed this phenotypic observation. In fact, SPAD index significantly decreased in calcareous soil, as compared to fertile soil (Fig. 2a). This decrease was estimated to 32% in Alex, 60% in DP and 47% in MK. On this problematic soil, Alex accumulates 70% more chlorophyll than DP and 30% more chlorophyll than MK.
Figure 1: Morphological aspect of Pea (Pisum sativum L.) plants cultivated on fertile and calcareous soils
Figure 2: SPAD index (a) and biomass production (b, mg plant−1) in Pea plants (Pisum sativum L.) cultivated on on fertile soil (FS) or calcareous soil (CS). Within columns, means with the same letter are not significantly different at α = 0.05 according to Fisher’s Least Significant Difference. Bars on the columns represent the standard error of the means (n = 10)
On calcareous soil, all plants exhibited a significant decrease in biomass production as compared to those cultivated on fertile soil (FS). Nevertheless, the negative effect of calcareous-induced iron deficiency was more pronounced in MK than in Alex and DP. The decrease in biomass production was estimated to be 30% in MK subjected to calcareous soil, 25% in DP and 10% in Alex (Fig. 2b). Even when significantly decreased on calcareous soil, plant growth in Alex remained 30% more important than that of DP and 40% than that of MK.
As compared to fertile soil, the cultivation of pea plants on calcareous soil significantly decreased net photosynthesis assimilation (An) in all genotypes. This decrease was estimated to be −33% in Alex, −55% in MK and −67% in DP (Fig. 3a). Even when grown on calcareous soil, An was 2.2 higher in Alex than DP and 1.4 higher in Alex than MK.
Figure 3: Net photosynthesis activity (An, µmol CO2 m−2 s−1, a), evapotranspiration (ET, mol H2O m−2 s−1, b) and stomatal conductance (SC, mol H2O m−2 s−1, c) in pea genotypes cultivated on fertile soil (FS) or calcareous soil (CS). Within columns, means with the same letter are not significantly different at α = 0.05 according to Fisher’s Least Significant Difference. Bars on the columns represent the standard error of the means (n = 10)
The measurements made on evapotranspiration (ET, Fig. 3b) and stomatal conductance (SC, Fig. 3c) showed the same scheme of variation with a less pronounced effect than An. Alex remained the least affected genotype on calcareous soil.
The analysis of the extractible active fraction of iron in plant organs demonstrated that roots represent the main organ of Fe accumulation, as compared to leaves, particularly in fertile soil (Fig. 4). On calcareous soil, the significant inhibitory effect of Fe uptake was more significant in roots (Fe concentration decreased by 43% in Alex, 57% in DP and 64% in MK, Fig. 4b) than in shoots (Fe concentration decreased by 37% in Alex, 57% in DP and 68% in MK, Fig. 4a).
Figure 4: Active Fe concentration (µg g−1 DW) in shoots (a) and roots (b) of Pea plants (Pisum sativum L.) cultivated on fertile soil (FS) or calcareous soil (CS). Within columns, means with the same letter are not significantly different at α = 0.05 according to Fisher’s Least Significant Difference. Bars on the columns represent the standard error of the means (n = 10)
All genotypes exhibited a significant increase in H2O2 accumulation in plant organs when cultivated on calcareous soil, with some genotypic differences (Fig. 5). In Alex, H2O2 increased by 10% in shoots (Fig. 5a) and by 23% in roots (Fig. 5b) of plants cultivated on calcareous soil, as compared to fertile soil. In DP, H2O2 was 2.5 times in shoots (Fig. 5a) and 1.56 times in roots (Fig. 5b) more important in calcareous soil as compared to fertile soil. In MK, H2O2 was 6.80 and 2.16 times more important in calcareous soil as compared to fertile soil, respectively in shoots (Fig. 5a) and roots (Fig. 5b). This phenomenon reflects the existence of an oxidative stress in these organs and Alex was the less affected genotype.
Figure 5: H2O2 concentration (µmol g−1 FW) in shoots (a) and roots (b) of Pea plants (Pisum sativum L.) cultivated on fertile soil (FS) or calcareous soil (CS). Within columns, means with the same letter are not significantly different at α = 0.05 according to Fisher’s Least Significant Difference. Bars on the columns represent the standard error of the means (n = 6, three replicates repeated twice)
When comparing plant organs, we noticed that shoots were more affected than roots. Values of H2O2 concentration were more important in shoots than in roots. Therefore, we analyzed the MDA concentrations in similar organs (Fig. 6). This substance reflected the lipid peroxidation of the cell membranes. Obtained results demonstrated a substantial increase of MDA in shoots and roots of DP and MK cultivated on calcareous soil (+50% in shoots and +36% in roots of DP and +70% in shoots and +96% in roots of MK, Figs. 6a and 6b). In Alex, we noticed an inverse behavior, MDA decreased by 10% in shoots and 20% in roots (Figs. 6a and 6b).
Figure 6: MDA concentration (µmol g−1 FW) in shoots (a) and roots (b) of Pea plants (Pisum sativum L.) cultivated on fertile soil (FS) or calcareous soil (CS). Within columns, means with the same letter are not significantly different at α = 0.05 according to Fisher’s Least Significant Difference. Bars on the columns represent the standard error of the means (n = 6, three replicates repeated twice)
The analysis of superoxide dismutase activity demonstrated no clear modifications in shoots of DP and MK cultivated on calcareous soil, as compared to fertile soil with a substantial increase in Alex (+60%) (Fig. 7a). In roots, all genotypes exhibited a clear decrease on calcareous soil (Fig. 7b). The above discriminated genotype (Alex) showed SOD activity about twice as important in shoots than in roots on calcareous soil. This behavior was lacking in the other genotypes (values of SOD are comparable).
Figure 7: SOD activity (unit mg−1 protein) in shoots (a) and roots (b) of Pea plants (Pisum sativum L.) cultivated on fertile soil (FS) or calcareous soil (CS). Within columns, means with the same letter are not significantly different at α = 0.05 according to Fisher’s Least Significant Difference. Bars on the columns represent the standard error of the mean (n = 6, three replicates repeated twice)
For shoot catalase, we measured a significant increase of this enzyme in Alex cultivated in calcareous soil, as compared to fertile soil. In DP and MK, CAT exhibited a slight increase (Fig. 8a). In fact, CAT activity was 2.0, 1.3 and 1.1 times more important in calcareous than in fertile soil, respectively, in Alex, DP and MK. In roots, the same scheme of variation was observed with a significant increase in Alex against slight stimulation in DP and MK (Fig. 8b). CAT activity was 1.8, 1.3 and 1.3 times higher in calcareous soil than that of fertile soil, respectively, in Alex, DP and MK.
Figure 8: CAT activity (unit mg−1 protein) in shoots (a) and roots (b) of Pea plants (Pisum sativum L.) cultivated on fertile soil (FS) or calcareous soil (CS). Within columns, means with the same letter are not significantly different at α = 0.05 according to Fisher’s Least Significant Difference. Bars on the columns represent the standard error of the means (n = 6, three replicates repeated twice)
In order to identify other traits of pea response to iron deficiency and explore the genotypic variability, we calculated the Fe use efficiency for photosynthesis (FeUE-An), Fe use efficiency for SOD activity in shoots (FeUE-SOD-Sh) and roots (FeUE-SOD-R) and Fe use efficiency for CAT activity in shoots (FeUE-CAT-Sh) and roots (FeUE-CAT-R) (Table 2). FeUE-An decreased in all genotypes cultivated on calcareous soil (Table 2). Nevertheless, Alex remained the least affected (FeUE-An decreased by 7%), as compared to DP (FeUE-An decreased by 54%) and MK (FeUE-An decreased by 47%). FeUE-SOD-Sh increased significantly in plants cultivated on calcareous soil, as compared to fertile soil (+20% in DP, +40% in MK and +120% in Alex) (Table 2). In roots, this parameter decreased in Alex and DP, and increased slightly in MK. For catalase, FeUE-CAT increased significantly in shoots and roots of all genotypes, with a more pronounced effect in Alex (Table 2). FeUE-CAT-Sh increased significantly in plants cultivated on calcareous soil, as compared to fertile soil (2.8 times in DP, 1.8 times in MK and 1.6 times in Alex) (Table 2). FeUE-CAT-R increased significantly in plants cultivated on calcareous soil, as compared to fertile soil (2.5 times in DP, 1.7 times in MK and 1.8 times in Alex) (Table 2).
Finally, we calculated Fe translocation (FeT) calculated as the ratio of shoot Fe content to total plant Fe content (Table 2) and expressed as percentage. Obtained results demonstrated that FeT increased in plants cultivated on calcareous soil, as compared to fertile one. Alex demonstrated higher capacities of Fe allocation to its shoots than the other genotypes.
In this study, we investigated the influence of bicarbonate induced Fe-deficiency stress on some physiological parameters and antioxidant enzyme activities in three pea genotypes. Under these experimental conditions, plant growth, chlorophyll accumulation, net photosynthesis, and iron concentrations were significantly decreased, while these reductions were less pronounced in Alex. In fact, it is well established that chloroplasts benefit from the most important amount of leaf iron. Thus, any degree of Fe deficiency affects the structure, function, and chlorophyll content of the chloroplasts [19]. In accordance with our results, Angulo et al. [20] reported that in calcareous soils, Fe is readily oxidized and forms insoluble ferric oxide, resulting in Fe deficiency-induced growth inhibition and leaf chlorosis. The influence of Fe on leaf chlorophyll content has been reported in several studies. For example, the presence of bicarbonate in the nutrient solution decreased leaf chlorophyll concentration in Arabidopsis [21] and in apple [22]. Karimi et al. [23] reported that leaf SPAD, plant dry weight, and root volume decreased dramatically in vines under bicarbonate conditions. The relationship between Fe and chlorophyll can be explained by its implication in the biosynthesis of the chlorophyll precursors δ-aminolevulinic acid and protochlorophyllide [24]. El-Gioushy et al. [25] demonstrated that the reduced mobility of the Fe-carrying system restricts chlorophyll production due to a decline in the mitochondrial cell charge for the excretion of mugineic acid. The lower leaf sapd index in the calcareous-induced Fe-deficiency may also be explained by the prevented or retarded thylakoid formation [26]. Ristic et al. [27] observed a negative linear correlation between chlorophyll content and damage to thylakoid membranes in wheat. Since Fe is directly involved in the biosynthesis of chlorophyll, any decrease in its availability leads to the disruption of the biosynthesis of this pigment [28]. Liu et al. [29] indicated that growth inhibition and photosynthesis depression under iron deficiency are mediated by systemic auxin signaling. Chaves et al. [30] demonstrated that photosynthesis is among the most affected parameters by iron deficiency, leading to reduced growth. About 80% of iron is found in photosynthetic apparatus and serves for the biosynthesis of cytochromes and other heme molecules, including chlorophyll, the electron transport chain and the biosynthesis of the complex Fe-S [31]. In fact, Fe is required for biological processes because of its role as a protein cofactor. Fe cofactors exist in three main forms (heme, nonheme, and Fe-S clusters) to allow proteins to carry out their metabolic functions. The photosynthetic electron transport chain requires all three forms of Fe cofactors. In the photosynthetic apparatus, two or three iron atoms are found in molecules directly related to photosystem II (PSII), 12 atoms in photosystem I (PSI), five in the cytochrome complex, and two in the ferredoxin molecule [32]. This distribution confirms the direct involvement of iron in photosynthesis and, consequently, plant yield [31].
In the present study, the observed genotypic differences consists in the relative tolerance of Alex that maintains higher plant growth and photosynthetic activity on calcareous soil as compared to DP and MK. This genotype developed a better capacity of iron uptake and allocation to shoots (Fe concentration was 1.7 and 2.1 times higher in shoots of Alex as compared to DP and MK, respectively, and 1.4 and 2.2 times higher in roots of Alex as compared to DP and MK, respectively). Thus, the relative tolerance of Alex can be explained by its capacity for iron remobilization, uptake, and distribution in the plant to support chlorophyll accumulation, photosynthesis, and plant growth. Previously, Briat et al. [33] demonstrated that the main nutrient that limits plant growth on calcareous soils is iron.
It is well established that iron deficiency induces oxidative stress in plant tissues due to the increased accumulation of ROS such as superoxide radicals and H2O2 [34]. In fact, plants starving with Fe are more prone to oxidative stress as Fe is a co-factor of many antioxidant enzymes. MDA is an indicator of these oxidative radicals that damage lipid membranes [7]. In the present study, H2O2 accumulation increased significantly in all plant organs grown on calcareous soil, except the shoot of Alex. MDA increased also in DP and MK organs, except shoots and roots of Alex. According to these results, Karimi et al. [23] observed a lower production of MDA and hydrogen peroxidase in Fe-chlorosis tolerant genotypes. Thus, the present results suggest that the relative tolerance of Alex to calcareous-induced Fe-deficiency spotted on some physiological levels can also be explained by a particular ability of this genotype to protect its photosynthetic organs against injury by ROS. However, analyses made on antioxidant enzyme activity demonstrated a significant increase of shoot SOD and shoots and roots CAT in Alex (activity less pronounced in DP and MK). Gretchen et al. [35] announced that upon Fe deficiency, the expression of specific ROS-scavenging molecules is up-or-down regulated, suggesting a need to prevent potential ROS-induced damage. In accordance with our results, several studies demonstrated an SOD activity increase in quince rootstocks subjected to direct and bicarbonate induced Fe-deficiency [9]. An increase of the SOD activity in response to Fe-deficiency was also observed in other plants [22,34,36]. Page et al. [37] suggested that the increase of SOD activity in the chloroplast under Fe-deficiency is due to the de novo biosynthesis of Fe-SOD over other plastid proteins and, the induction of a chloroplastic Mn-SOD. In the literature, SOD is usually considered the first line of defense against oxidative stress [38]. By controlling the SteadyState superoxide levels, SOD plays an important protective role against cellular oxidative damage, because superoxide acts as a precursor of more cytotoxic or highly reactive oxygen derivatives, such as peroxynitrite or hydroxyl radical [38]. CAT, APX, and GPX are also H2O2 scavengers that are able to convert H2O2 to H2O [9,39]. Krouma et al. [7] reported impaired photosystem II efficiency under Fe deprivation that is correlated with hydrogen peroxide accumulation, higher superoxide dismutase activity and a significant decrease in catalase activity together with rising levels of dehydroascorbic acid indicated a strong disturbance of the redox homeostasis. In our study, SOD activity increased in shoots while CAT increased in shoots and roots of Alex cultivated on calcareous soil (no clear behavior in the other genotypes). It becomes clear that in addition to its ability to mobilize and uptake more iron, Alex has an efficient antioxidant mechanism that protects its tissues against ROS. SOD and CAT plays a key role in shoots while CAT takes over this role in roots. Previousely, Rahman et al. [40] reported that iron deficiency impairs photosynthetic efficiency, plant growth and biomass yield. In contrast, Fe deficiency was found to regulate antioxidant mechanisms in Prunus rootstocks, where SOD, POD, and CAT activities were differentially induced [14].
By progressing in the elucidation of the mechanisms of Pea tolerance to calcareous-induced Fe-deficiency, we noticed an important increase in Fe use efficiency for photosynthesis and SOD activity in shoots and for CAT activity in shoots and roots, particularly in Alex. This result adds to our investigation another tool to discern Alex tolerance to induced Fe-deficiency. The calculated FeT demonstrated that Alex allocated 20% more iron than DP and 23% more iron than MK to its shoots on calcareous soil. This genotype also efficiently uses the low available amount of iron to develop the maximum potentialities of photosynthesis, plant growth and antioxidant activity.
Taken together, our results demonstrated that calcareous-induced iron deficiency stimulates young leaf chlorosis, decreases chlorophyll and plant growth, and disrupts photosynthesis and Fe nutrition in the studied pea genotypes. Some genotypic differences were observed, and Alex was found to be relatively tolerant. This genotype is characterized by (1) high capacity of iron uptake and translocation to shoots (FeT) on calcareous soil, (2) an important ability to stimulate SOD and CAT activities, protecting tissues against injury by ROS, and (3) better Fe use efficiency for photosynthesis and CAT and SOD activities. Calcareous soils, largely abundant in Tunisia are problematic for agricultural production because of the low availability of iron. Screening of tolerant genotypes or genotypes based on their efficiency of Fe uptake, translocation to shoots, and use for the key metabolic reaction remains the low-coast, eco-friendly and efficient approach, other than chemical fertilization.
Authorship: The authors confirm contribution to the paper as follows: study conception and design: K. Abdelmajid; data collection: B. Sameh; analysis and interpretation of results: B. Sameh, E. Hasna; draft manuscript preparation: B. Sameh, K. Abdelmajid. All authors reviewed the results and approved the final version of the manuscript.
Funding Statement: This study was supported by the Ministry of Higher Education and Scientific Research and conducted within the framework of the Partnership for Research and Innovation in the Mediterranean Area (PRIMA), Project DiVicia: Use and management of Vicia species for sustainability and resilience in biodiversity-based farming systems.
Conflicts of Interest: The authors declare that they have no conflicts of interest to report regarding the present study.
References
1. Krouma, A. (2021). Differential response of Pea (Pisum sativum L.) genotypes to iron deficiency in relation to the growth, rhizosphere acidification and ferric chelate reductase activities. Australian Journal of Crop Science, 15(6), 925–932. DOI 10.3316/informit.157871497907046. [Google Scholar] [CrossRef]
2. Ammari, A., Mengel, K. (2006). Total soluble Fe in soil solutions of chemically different soils. Geoderma, 136(3–4), 876–885. DOI 10.1016/j.geoderma.2006.06.013. [Google Scholar] [CrossRef]
3. Tang, C., Robson, A., Dilworth, M. I. (1990). The role of iron in nodulation and nitrogen fixation in Lupinus angustifolius L. New Phytologyst, 114, 173–182. DOI 10.1111/j.1469-8137.1990.tb00388.x. [Google Scholar] [CrossRef]
4. Shevela, D., Eaton-Rye, J. J., Shen, J. R. (2012). Photosystem II and the unique role of bicarbonate: A historical perspective. Biochimica et Biophysica Acta (BBA)–Bioenergetics, 1817(8), 1134–1151. DOI 10.1016/j.bbabio.2012.04.003. [Google Scholar] [CrossRef]
5. Merchant, S., Sawaya, M. R. (2005). The light reactions: A guide to recent acquisitions for the picture gallery. Plant Cell, 17(3), 648–663. DOI 10.1105/tpc.105.030676. [Google Scholar] [CrossRef]
6. Becana, M., Dalton, D. A., Moran, J. F., Iturbe-Ormaetxe, I., Matamoros, M. A. et al. (2000). Reactive oxygen species and antioxydants in legume nodules. Physiologia Plantarum, 10(4), 372–381. DOI 10.1034/j.1399-3054.2000.100402. [Google Scholar] [CrossRef]
7. Krouma, A., Ben Hamed, K., Abdelly, C. (2008). Symbiotic response of common bean (Phaseolus vulgaris L.) to iron deficiency. Acta Physiologiea Plantarum, 30(1), 27–34. DOI 10.1007/s11738-007-0087-5. [Google Scholar] [CrossRef]
8. Prity, S. A., El-Shehawi, A. M., Elseehy, M. M., Tahura, S., Kabir, A. H. (2021). Early-stage iron deficiency alters physiological processes and iron transporter expression, along with photosynthetic and oxidative damage to sorghum. Saudi Journal of Biological Sciences, 28(8), 4770–4777. DOI 10.1016/j.sjbs.2021.04.092. [Google Scholar] [CrossRef]
9. Pandey, S., Fartyal, D., Agarwal, A., Shukla, T., James, D. et al. (2017). Abiotic stress tolerance in plants myriad role of ascorbate peroxidase. Frontiers in Plant Science, 20(8), 581. DOI 10.3389/fpls.2017.00581. [Google Scholar] [CrossRef]
10. Molassiotis, A., Tanou, G., Diamantidis, G., Patakas, A., Therios, I. (2006). Effects of 4-month Fe deficiency exposure on Fe reduction mechanism, photosynthetic gas exchange, chlorophyll fluorescence and antioxidant defence in two peach rootstocks differing in Fe deficiency tolerance. Journal of Plant Physiology, 163(2), 176–185. DOI 10.1016/j.jplph.2004.11.016. [Google Scholar] [CrossRef]
11. Ouled Youssef, I., Krouma, A. (2021). Functional dissection of magnesium nutrition and use efficiency in common bean. Agronomy Journal, 113(1), 261–269. DOI 10.1002/agj2.20506. [Google Scholar] [CrossRef]
12. Köseoglu, A. T., Açikgöz, V. (1995). Determination of iron chlorosis with extractable iron analysis in peach leaves. Journal of Plant Nutrition, 8(1), 153–161. DOI 10.1080/01904169509364892. [Google Scholar] [CrossRef]
13. Bradford, M. (1976). A rapid and sensitive method for the quantitation of microgram quantities of protein utilizing the principle of protein-dye binding. Analytical Biochemistry, 72(1–2), 248–254. DOI 10.1016/0003-2697(76)90527-3. [Google Scholar] [CrossRef]
14. Scebba, F., Sebastiani, L., Vitagliano, C. (1999). Protective enzymes against activated oxygen species in wheat (Triticum aestivum L.) seedlings: Responses to cold acclimation. Plant Physiology, 155(6), 762–768. DOI 10.1016/S0176-1617(99)80094-7. [Google Scholar] [CrossRef]
15. Beauchamp, C., Fridovich, I. (1971). Superoxide dismutase: Improved assay and an assay applicable to acrylamide gels. Analytical Biochemistry, 44(1), 276–287. DOI 10.1016/0003-2697(71)90370-8. [Google Scholar] [CrossRef]
16. Aebi, H. (1984). Catalase in vitro. Methods Enzymology, 105, 121–126. DOI 10.1016/S0076-6879(84)05016-3. [Google Scholar] [CrossRef]
17. Esterbauer, H. (1996). Estimation of peroxidative damage. A critical review. Pathologie-Biologie, 44(1), 25–28. DOI 10.1007/978-3-642-85011-0-5. [Google Scholar] [CrossRef]
18. Alexieva, V., Sergiev, I., Mapelli, S., Karanov, E. (2001). The effect of drought and ultraviolet radiation on growth and stress markers in pea and wheat. Plan, Cell and Environment, 24(12), 1337–1344. DOI 10.1046/j.1365-3040.2001.00778.x. [Google Scholar] [CrossRef]
19. Fu, L., Chai, L., Ding, D., Pan, Z., Peng, S. (2016). A novel citrus rootstock tolerant to iron deficiency in calcareous soil. American Society for Horticultural Science, 141(2), 112–118. DOI 10.21273/JASHS.141.2.112. [Google Scholar] [CrossRef]
20. Angulo, M., García, M. J., Alcántara, E., Pérez-Vicente, R., Romera, F. J. (2021). Comparative study of several Fe deficiency responses in the Arabidopsis thaliana ethylene insensitive mutants ein2-1 and ein2-5. Plants, 10(2), 262. DOI 10.3390/plants10020262. [Google Scholar] [CrossRef]
21. Msilini, N., Attia, H., Bouraoui, N., M’rah, S., Ksouri, R. et al. (2009). Responses of Arabidopsis thaliana to bicarbonate-induced iron deficiency. Acta Physiologiae Plantarum, 31(4), 849–853. DOI 10.1007/s11738-009-0318-z. [Google Scholar] [CrossRef]
22. Sahin, O., Gunes, A., Taskin, M. B., Inal, A. (2017). Investigation of responses of some apple (Mallus x domestica Borkh.) genotypes grafted on MM106 and M9 rootstocks to lime-induced chlorosis and oxidative stress. Scientia Horticulturae, 219, 79–89. DOI 10.1016/j.scienta.2017.03.006. [Google Scholar] [CrossRef]
23. Karimi, R., Salimi, F. (2021). Iron-chlorosis tolerance screening of 12 commercial grapevine (Vitis vinifera L.) genotypes based on phytochemical indices. Scientia Horticulturae, 283, 110–111. DOI 10.1016/j.scienta.2021.110-111. [Google Scholar] [CrossRef]
24. Marschner, H. (1995). Mineral nutrition of higher plants. 2nd editionLondon: Academic Press. [Google Scholar]
25. El-Gioushy, S. F., Ding, Z., Bahloul, A. M. E., Gawish, M. S., Abou El Ghit, H. M. et al. (2021). Foliar application of nano, chelated, and conventional iron forms enhanced growth, nutritional status, fruiting aspects, and fruit quality of Washington navel orange trees (Citrus sinensis L. Osbeck). Plants, 10(12), 2577. DOI 10.3390/plants10122577. [Google Scholar] [CrossRef]
26. Terry, N. (1981). Physiology of trace element toxicity and its relation to iron stress. Journal of Plant Nutrition, 3(1–4), 561–578. DOI 10.3390/plants10122577. [Google Scholar] [CrossRef]
27. Ristic, Z., Bukovnik, U., Prasad, P. V. (2007). Correlation between heat stability of thylakoid membranes and loss of chlorophyll in winter wheat under heat stress. Crop Science, 47(5), 2067–2073. DOI 10.2135/cropsci2006.10.0674. [Google Scholar] [CrossRef]
28. Pestana, M., Correia, P. J., Saavedra, T., Gama, F., Abadía, A. (2001). Effectiveness of different foliar iron application to control iron chlorosis in orange trees grown on a calcareous soil. Journal of Plant Nutrition, 24(4–5), 613–622. DOI 10.1081/PLN-100103656. [Google Scholar] [CrossRef]
29. Liu, K., Yue, R., Yuan, C., Liu, J., Zhang, L. et al. (2015). Auxin signaling is involved in iron deficiency-induced photosynthetic inhibition and shoot growth defect in rice (Oryza sativa L.). Journal of Plant Biology, 58(6), 391–401. DOI 10.1007/s12374-015-0379-z. [Google Scholar] [CrossRef]
30. Chaves, M. M., Flexas, J., Pinheiro, C. (2009). Photosynthesis under drought and salt stress: Regulation mechanisms from whole plant to cell. Annals of Botany, 103(4), 551–560. [Google Scholar]
31. Briat, J. F., Curie, C., Gaymard, F. (2007). Iron utilization and metabolism in plants. Current Opinion in Plant Biology, 10(3), 276–282. DOI 10.1016/j.pbi.2007.04.003. [Google Scholar] [CrossRef]
32. Varotto, C., Maiwald, D., Pesaresi, P., Jahns, P., Salamini, F. (2002). The metal ion transporter IRT1 is necessary for iron homeostasis and efficient photosynthesis in Arabidopsis thaliana. Plant Journal, 31(5), 589–599. DOI 10.1046/j.1365-313x.2002.01381.x. [Google Scholar] [CrossRef]
33. Briat, J. F., Dubos, C., Gaymard, F. (2015). Iron nutrition, biomass production, and plant product quality. Trends in Plant Science, 20(1), 33–40. DOI 10.1016/j.tplants.2014.07.005. [Google Scholar] [CrossRef]
34. Salama, Z. A. E., El-Beltagi, H. S., El-Hariri, D. M. (2009). Effect of Fe deficiency on antioxidant system in leaves of three flax genotypes. Notulae Botanicae Horti Agrobotanici Cluj-Napoca, 37(1), 122–128. DOI 10.15835/nbha3713107. [Google Scholar] [CrossRef]
35. Gretchen, E. K., Marinus, P. (2020). Regulation of iron homeostasis and use in chloroplasts. International Journal of Molecular Sciences, 21(9), 3395. DOI 10.3390/ijms21093395. [Google Scholar] [CrossRef]
36. Kabir, A. H., Rahman, M. M., Haider, S. A., Paul, N. K. (2015). Mechanisms associated with differential tolerance to Fe deficiency in okra (Abelmoschus esculentus Moench). Environmental and Experimental Botany, 112(9), 16–26. DOI 10.1016/j.envexpbot.2014.11.011. [Google Scholar] [CrossRef]
37. Page, M. D., Allen, M. D., Kropat, J., Urzica, E. I., Karpowicz, S. J. et al. (2012). Fe sparing and Fe recycling contribute to increased superoxide dismutase capacity in iron-starved Chlamydomonas reinhardtii. Plant Cell, 24(6), 2649–2665. DOI 10.1105/tpc.112.098962. [Google Scholar] [CrossRef]
38. Halliwell, B., Gutteridge, J. M. C. (1989). Free radicals in biology and medicine. 2nd editionNew York, USA: Oxford University Press. [Google Scholar]
39. Das, K., Roychoudhury, A. (2014). Reactive oxygen species (ROS) and response of antioxidants as ROS-scavengers during environmental stress in plants. Frontiers in Environmental Science, 2, 53. DOI 10.3389/fenvs.2014.00053. [Google Scholar] [CrossRef]
40. Rahman, M. A., Kabir, A. H., Song, Y., Lee, S. H., Hasanuzzaman, M. et al. (2021). Nitric oxide prevents Fe deficiency-induced photosynthetic disturbance, and oxidative stress in alfalfa by regulating Fe acquisition and antioxidant defense. Antioxidants, 10(10), 1556. DOI 10.3390/antiox10101556. [Google Scholar] [CrossRef]
Cite This Article
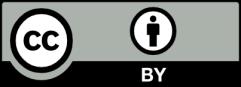
This work is licensed under a Creative Commons Attribution 4.0 International License , which permits unrestricted use, distribution, and reproduction in any medium, provided the original work is properly cited.