Open Access
ARTICLE
Effects of Heat Stress during Seed Filling Stage on Brassica napus Seed Oil Accumulation and Chlorophyll Fluorescence Characteristics
1 State Key Laboratory for Managing Biotic and Chemical Threats to the Quality and Safety of Agro-Products, Institute of Virology and Biotechnology, Zhejiang Academy of Agricultural Sciences, Hangzhou, 310021, China
2 Institute of Crop Science and Nuclear Technology Utilization, Zhejiang Academy of Agricultural Sciences, Hangzhou, 310021, China
* Corresponding Author: Hua Wang. Email:
(This article belongs to the Special Issue: Symbiotic Associations for Nutrients Management and Complexes Formation for Better Agricultural Crops Productivity under Biotic and Abiotic Stresses)
Phyton-International Journal of Experimental Botany 2023, 92(2), 333-348. https://doi.org/10.32604/phyton.2022.023252
Received 17 April 2022; Accepted 22 May 2022; Issue published 12 October 2022
Abstract
As global temperature rise, the threat of heat stress to rapeseed production is becoming more obvious. Exploring the response characteristics of two important biological pathways, oil accumulation and photosynthesis, to heat stress during B. napus seed filling is helpful in the genetic improvement of heat-tolerant rapeseed. The effects of heat stress on seed oil accumulation and chlorophyll fluorescence characteristics of 29 B. napus germplasms with different oil content and environmental sensitivity, including 6 rapeseed varieties which exhibited environment-sensitive/insensitive and with high, medium or low oil content, were tested by whole plant heat stress or the in vitro silique culture system. Both assay exhibited similar trend on oil content of the rapeseed germplasms. The heat effect on the chlorophyll fluorescence kinetic parameters Fv/Fm, ETR and Y(II) were also consistent. Heat stress significantly decreased oil content, although there was abundant genetic variation on heat tolerance among the genotypes. Correlation analysis showed that the decrease rate of Fv/Fm of silique heat-stressed B. napus developing seed was positive correlative to the decrease rate of mature seed oil content of the whole plant heat-stressed rapeseed (R = 0.9214, P-value < 0.01). Overall, the results indicated that heat stress inhibited oil accumulation and photosynthesis in B. napus developing seed. The decrease rate of chlorophyll fluorescence parameter Fv/Fm of heat-stressed developing seed could be used as the index of heat tolerant rapeseed identification. Further, two heat insensitive rapeseed varieties with high oil content were identified.Keywords
Nomenclature
Fv/Fm | Maximal quantum yield of PSII photochemistry |
ETR | Electron transport rate |
Y(II) | Quantum yield of photochemical energy conversion in PSII |
RFv/Fm | Decreased rate of Fv/Fm |
CV | Coefficient of variation |
Seed development is a critical stage in the life cycle of oil crops. The genetic and environmental factors which involved in this process might directly or indirectly affect the accumulation of rapeseed storage substances [1–4]. As an abiotic stress, heat stress is a major factor limiting crop yield and quality [5–7]. In winter rapeseed production area, especially in the Yangtze River Basin, the temperature rises rapidly during the ripening period of rapeseed, which is easy to cause heat-forced maturity of rapeseed [8]. With the impact of global climatic changes and the frequency of heat wave increased, the negative effects of heat stress on yield and quality of rapeseed are more significant [9,10]. Reduce the harm of heat damage has become an urgent request for the sustainable development of oilseed rape industry [11,12].
The response of plants to heat stress is an extremely complex process. Transcriptome changes resulted from heat stress in the seed of B. napus at filling stage had been analyzed by microarray and RNA-seq approaches. It was demonstrated that in addition to a large number HSFs and HSPs, some genes related to lipid degradation were also up-regulated [7,13,14]. A heat-induced BnGLYI gene, which encoded a glyoxalase I enzyme, was demonstrated to participate in B. napus seed thermal tolerance [15]. High night temperature up-regulated the expression of genes related to fatty acid catabolism, including genes associated with β-oxidation and glyoxylate metabolism, thereby causing the lower seed oil content [6]. The heat induced DNA methylation has been indicated as key factors in B. napus adaptation to heat stress [16]. Additionally, the differences in homoeologous gene expression and alternative splicing patterns in allotetraploid plants such as B. napus might improve their adaptability to heat stresses [17].
The embryos of rapeseed, soybean and Arabidopsis are green during their seed development. Although such “green seeds” are still heterotrophic sink tissues in general, their chloroplasts have thylakoid structure and the same chlorophyll protein complex as leaves [18]. Hence, these green seeds still have certain photosynthetic carbon fixation capacity [19]. In B. napus developing seeds, the peak of the photosynthetic capacity coincides with the period of oil accumulation rapidly [20]. BnWRI1, the key regulator of B. napus seed oil content, synergistically controlled two important biological pathways: oil biosynthesis and photosynthesis during seed development [3].
Photosynthesis is the basis of crop yield formation and one of the most heat vulnerable physiological processes [21–25]. Heat stress at seedling stage resulted in irreversible damage to PSII and decrease of net photosynthetic rate in C3 sunflower and C4 maize leaves [26]. Our previous studies have found that heat stress at seed filling stage down-regulates the expression of BnWRI1, a key regulator of oil biosynthesis, and its downstream genes (including those of the de novo fatty acid synthesis pathway), thus blocking the carbon flows to triacylglycerol biosynthesis. Furthermore, heat stress induced the photoinhibition pathway, which inhibited photosynthesis, respiration and the maximum photoperiod quantum yield of PSII (Fv/Fm) in B. napus developing seeds. Consequently, the inhibition of BnWRI1 mediated fatty acid biosynthesis pathway and the destruction of PSII might be the main reasons for the decreased oil content in heat-stressed B. napus seeds [14]. Here, we examined the effects of heat stress on seed oil content and the chlorophyll fluorescence parameters of B. napus germplasms with significant differences in environmental sensitivity, to explore the physiological mechanism of rapeseed yield and quality reduction caused by heat stress, and to seek suitable evaluation index of heat tolerance during B. napus seed filling stage. This study will provide useful information about the cultivation of heat-tolerant rapeseed varieties.
The 29 tested B. napus accessions were provided by the Zhejiang Academy of Agricultural Sciences, Hunan Agricultural University; Institute of Oil Crops, Chinese Academy of Agricultural Sciences; Southwest University; Yunnan Agricultural University and Zhejiang University (China) (Table S1). Among them, HS1, HS2, HS3, HS4, HS5 and HS6 are the previously screened B. napus germplasm resources of environmentally insensitive or sensitive genotype with different oil content [4,11,27]. The detailed information is given in Table S2.
2.2.1 Whole Plant Heat Stress of B. napus
This study was conducted in the Zhejiang Academy of Agricultural Sciences, Hangzhou, China (120°11′E, 30°18′N) for three consecutive years (2015–2017). The B. napus accessions were sown on October 8th each year and potted with 1 plant per pot and 10 plants per germplasm. All plants were grown in a greenhouse under the same conditions according to the conventional cultivation management of rapeseed. At the seed filling stage (April 06 every year), six plants of similar size were selected from each tested rapeseed germplasm, and then transferred in pairs into two growth chambers for heat stress (HT) or control treatment (CK), respectively. The parameters of growth chambers were set as described by Huang et al. [14]. Briefly, the growth chambers were set as under a 14 h-light (5:00 to 19:00) photoperiod with light intensity of 300 μmol m−2 s−1 and relative humidity of 70%. Night temperature was 18°C. For the control chamber, daytime temperature kept constant at 23°C. The heat-stress chamber was set to gradually heat up from 23°C to 35°C at 5:00 to 10:00, maintain at 35°C for 4 h until 14:00, gradually cool down from 35°C to 23°C during 14:00 to 19:00, and then rapidly cool down to 18°C. After 15 days of treatment, all plants were transferred back to the greenhouse and continued to grow under the same conventional conditions until each rapeseed plant matured normally. Mature seeds were harvested by each rapeseed plant individually when siliques desiccation, and their oil contents were measured.
2.2.2 In Vitro Heat Stress of B. napus Silique
Silique heat-stress treatments were performed using the method from Huang with minor modifications [14]. All rapeseed accessions were grown in the experimental field at the Zhejiang Academy of Agricultural Sciences, Hangzhou, China, during the normal growing seasons. At full bloom stage, ten plants of similar size from each rapeseed accession were selected to mark the flowers that open in the day. On the 20 days after flowering (DAF), 180 siliques derived from the marked flowers were excised from the marked plants for each rapeseed accession, and randomly divided into heat-stress and control groups. All siliques were incubated on petri dishes containing Murashige & Skoog medium with 2% sucrose by the pedicels inserted into the medium. Siliques were cultivated in growth chambers under a 14 h-light (5:00 to 19:00) photoperiod with light intensity of 120 μmol m−2 s−1 and relative humidity of 60%. Night temperature maintained at 18°C. For the control group, daytime temperature remained constant at 23°C. For the heat-stress group, daytime temperature regime was as follows: increased from 18°C to 23°C at 5:00, maintained at 23°C for 3 h, increased from 23°C to 37°C at 8:00, maintained at 37°C for 7 h, decreased from 37°C to 23°C at 15:00, maintained at 23°C for 4 h, decreased from 23°C to 18°C at 19:00 (Fig. S1). After the second heat treatment (15:00), the seeds were separated from each rapeseed sample for analysis of seed chlorophyll fluorescence and oil content.
2.2.3 Chlorophyll Fluorescence Measurement
Chlorophyll fluorescence was determined with an MAXI version Imaging-PAM (Heinz Walz GmbH, Effeltrich, Germany) following the manufacturer. For each accession, no less than 20 seeds were used. For the convenience of comparison, the seeds of the same B. napus germplasm with or without heat stress were placed adjacent to each other. The chlorophyll fluorescence images and Fv/Fm were collected according to Huang et al. [14]. The experiments were arranged with three biological replicates. The chlorophyll fluorescence parameters at 3 positions were read from each sample, and the average values were taken as the results. The Fv/Fm decreased rate of the heat-stressed B. napus developing seeds (RFv/Fm) was calculated as:
where Fv/Fm (CK) and Fv/Fm (HT) denote the Fv/Fm of heat-stress B. napus developing seeds or the respective control.
Oil content of B. napus mature seed was determined by Soxhlet extraction method [28]. Oil content and oil per seed of B. napus developing seeds were determined according to the method of Wu et al. [3].
All of the experiments were conducted with three independent biological replicates and two technical replicates unless otherwise specified. The data was statistically analyzed by Student’s t-test using Microsoft Excel 2010.
3.1 Effects of Whole Plant Heat Stress on B. napus Mature Seed Oil Yield
In this study, we investigated the effects of heat stress on rapeseed at seed filling stage. To this aim, we firstly checked the heat effects on the B. napus germplasms HS1 to HS6, which have obvious differences in seed oil content and environmental sensitivity (Table S2). As displayed in Figs. 1A and 1C, the seed oil content and oil yield per plant of the tested B. napus germplasms decreased after the whole plant heat stress, except for the insignificant change of seed oil content of HS1. Notably, the influence of heat stress on oil yield per plant was more pronounced. These results demonstrated that heat stress is an important environmental factor affecting B. napus seed oil accumulation and reducing its economic benefits.
Figure 1: Effects of whole plant heat stress on B. napus mature seed oil yield. (A) Mature seed oil content of B. napus germplasms with different environmental sensitivity that were exposed to whole plant heat stress (HT) or grown in control (CK) condition. The value represented mean ± SD, n = 3 biological replicates (with 2 technical replicates). (B) Decrease rate (%) of mature seed oil content of whole plant heat-stressed B. napus plants relative to those of the corresponding control. (C) Oil yield per plant of B. napus genotypes with different environmental sensitivity exposure to whole plant heat stress (HT) or grown in control (CK) condition. The value represented mean ± SD, n = 3 biological replicates. (D) Decrease rate (%) of oil yield per plant of whole plant heat-stressed B. napus plants relative to those of the corresponding control. Asterisks indicate significant differences in the marked comparisons calculated using t-test: *, P-value < 0.05; **, P-value < 0.01
Pairwise comparisons were performed between the environmentally insensitive and sensitive rapeseeds with high, medium or low seed oil content respectively. It was noticed that the decrease rate of mature seed oil content and oil yield per plant of heat-stressed environmental insensitive B. napus germplasms HS1, HS3 and HS5 were lower than the decrease rate in corresponding environmental sensitive B. napus germplasms HS2, HS4 and HS6 (Figs. 1B and 1D). These results indicated that HS1, HS3 and HS5 were more resistant to heat damage than HS2, HS4 and HS6 respectively at seed filling stage, and the heat tolerance of rapeseed accessions was an important factor affecting their environmental adaptability.
3.2 Effects of in Vitro Silique Heat Stress on B. napus Developing Seed Oil Accumulation
At 20 days after flowering (DAF), rapeseed has entered the stage of rapid accumulation of oil and other storage reserves, which is also a sensitive period to heat stress [14]. The oil content and oil per seed of developing seed collected from siliques subjected to heat stress (HT) decreased significantly compared with those of the corresponding temperature-suitable culture samples (CK) in all tested B. napus germplasms (Fig. 2), indicating that even short-term heat stress during seed development had obvious adverse effects on B. napus seed oil accumulation.
Figure 2: Effects of in vitro silique heat stress on B. napus developing seed oil accumulation. (A) Effects of silique heat stress on developing seed oil content of B. napus germplasms with different environmental sensitivity. The developing seeds were isolated from siliques exposure to heat-stress (HT) or cultured under control condition (CK). The value represented mean ± SD, n = 3 biological replicates (with 2 technical replicates). (B) Decrease rate (%) of oil content in developing seeds isolated from heat-stressed B. napus siliques relative to those of the corresponding control. (C) Effects of in vitro silique heat stress on oil per seed in developing seeds of B. napus germplasms with different environmental sensitivity. The value represented mean ± SD, n = 3 biological replicates. (D) Decrease rate (%) of oil per seed of developing seeds isolated from heat-stressed B. napus siliques relative to those of the corresponding control. Asterisks indicate significant differences in the marked comparisons calculated using t-test: *, P-value < 0.05; **, P-value < 0.01
The environmentally insensitive and sensitive rapeseeds with high, medium or low seed oil content were also pairwisely compared. The results showed that the decrease rate in developing seed oil content and oil per seed of in vitro silique heat-stressed environmental insensitive B. napus germplasms HS1, HS3 and HS5 were lower than the decrease rate in corresponding environmental sensitive B. napus germplasms HS2, HS4 and HS6 (Figs. 2B and 2D), which was consistent with that of the whole plant heat stress to mature seed oil yield in these rapeseed germplasms (Fig. 1). Taken together, the change rate of seed oil accumulation of each B. napus accessions exposure to silique heat stress compared with that of the corresponding suitable cultured sample could reflect the heat tolerance of the rapeseed germplasm during seed filling stage.
3.3 Effects of in Vitro Silique Heat Stress on Chlorophyll Fluorescence Characteristics of B. napus Developing Seeds
The changes of seed chlorophyll fluorescence parameters caused by silique heat stress in B. napus germplasms HS1 to HS6 were measured at the same interface by modulated chlorophyll fluorescence system Imaging-PAM. As shown in Table 1, the effects of heat stress were apparent in the chlorophyll fluorescence characteristics of B. napus developing seeds. The Fv/Fm, ETR and Y(II) were all decreased in heat-stressed seed of each rapeseed accessions. Again, the chlorophyll fluorescence parameters of the environmentally insensitive rapeseed germplasms HS1, HS3 and HS5 were more stable to heat stress than those of the corresponding environmentally sensitive rapeseed germplasms HS2, HS4 and HS6, implying the changes in these chlorophyll fluorescence parameters were correlated with the heat sensitivity at B. napus seed filling stage.
The coefficient of variation (CV) of parameters in B. napus accessions were compared. The CVs of seed Fv/Fm, ETR and Y(II) in B. napus silique cultured under moderate temperatures ranged from 1.12 to 3.10, 7.50 to 18.28 and 6.74 to 24.76, respectively. Meanwhile, the CVs of seed Fv/Fm, ETR and Y(II) in heat-stressed B. napus silique ranged from 1.95 to 10.74, 14.75 to 34.92 and 11.84 to 49.49, respectively (Table 1). Overall, the CVs of each parameter of the HT seed exhibited an increasing trend compared with the CVs of the corresponding CK seed in the rapeseed accessions, suggesting that heat stress not only inhibited photosynthetic activity of B. napus developing seed, but also increased its variation within B. napus germplasm. It is noteworthy that, in the same B. napus accession, the CVs of Fv/Fm were significantly less than the CVs of ETR or Y (II) under both suitable and heat-stress conditions, indicating that the dispersion degree of this photosynthetic index was small within same rapeseed germplasm. Among the chlorophyll fluorescence parameters detected, Fv/Fm was relatively uniform among different seeds of the same rapeseed accession, which could be used as an important index for the heat response of rapeseed during seed filling.
3.4 Response of B. napus Germplasms to Heat Stress at Seed Filling Stage
For further validation of the effects of heat stress on B. napus seed photosynthetic characteristic and oil content, we determined the changes of mature seed oil content of whole plant heat-stressed B. napus plant and the RFv/Fm of silique heat-stressed B. napus developing seeds in 29 rapeseed accessions with different genetic backgrounds. The seed Fv/Fm of heat-stressed silique decreased compared to that of the corresponding temperature-suitable culture sample (CK) in all the test rapeseed germplasm materials except HS17 (Table 2), showing that heat stress damaged the PSII of B. napus developing seeds and disturbed their photosynthetic activity. The changes of Fv/Fm in heat-stressed developing seeds were varied between the rapeseed accessions, indicating that the seed photosystem for each B. napus germplasms had different sensitivity to heat stress at filling stage. Similarly, the mature seed oil content of 15 day heat-stressed plants decreased compared with that of the corresponding control grown under suitable temperature (Table 3). However, the decrease rate of mature seed oil content varied with B. napus germplasm.
The coefficient of variation of the developing seed RFv/Fm in silique heat-stress and the coefficient of variation of the decrease rate of the mature seed oil content of whole plant heat-stressed B. napus plants were 88.35% and 68.48%, respectively (Table 4), suggesting that there were abundant genetic variations in heat tolerance of seed photosynthesis and oil accumulation of these rapeseed germplasms.
The correlation analysis revealed that seed RFv/Fm of the heat-stressed 20 DAF siliques was significantly positively correlated with the decrease rate of mature seeds oil content of the whole plant heat-stressed rapeseed (R = 0.9214, P-value < 0.01). Therefore, RFv/Fm could be used as an important reference index for screening and evaluating the B. napus heat tolerance during seed filling stage (Fig. 3).
Figure 3: The RFv/Fm of silique heat-stressed B. napus developing seed was positive correlated with the decrease rate of mature seed oil contentin whole plant heat-stressed B. napus
Combining the results of Tables 2 and 3, it was found that, ten B. napus accessions (HS1, HS7, HS8, HS15, HS17, HS20, HS23, HS27, HS28 and HS29) had RFv/Fm < 5% in developing seeds isolated from heat-stressed siliques. After 15 days of whole plant heat-stress at the critical period of oil accumulation, the decrease rate of mature seed oil content of these B. napus accessions were all less than 3%, exhibiting stable oil content in response to heat-stress. Among them, the mature seed oil contents of rapeseed germplasms HS27 and HS29 heat-stressed for 15 days were still higher than 52%. These two germplasms with high oil content and heat insensitive could be used as excellent resources in rapeseed breeding for increase heat tolerance.
The seed oil content of rapeseed is a complex quantitative trait controlled by both genetics and environmental factors [29,30]. The seed filling period is the critical stage in formation of rapeseed yield and quality [3,31,32]. The analysis of thermotolerance of B. nupus germplasms with different genetic backgrounds showed that heat stress at seed filling stage generally reduced the seed oil content of the tested accessions (Table 3; Figs. 1A and 1C), which indicated that heat stress was an important environmental factor limiting rapeseed oil yield. It was observed that only 7 h × 2 heat stresses at 37°C had resulted significant decreased oil content (%) of developing seed (Fig. 2). Considering the tiny seed mass of 20 DAF B. napus seed [14], this is a reasonable result. The pairwise comparison of the heat responses of environmental insensitive or sensitive B. nupus germplasms with high, medium or low oil content respectively showed that, whether the heat-stress were performed with the whole plant treatment or the in vitro silique culture system, the thermotolerance of B. napus seed oil accumulation was accordant with the insensitive or sensitivity characteristic of seed oil content to the environment in general. These results suggested that the stability of seed oil content to heat stress was an important phenotype of B. napus environmental adaptability.
Although the seed of B. napus is not a typical photosynthetic organ, the photosynthetic activity of the developing seed itself plays an important role in oil accumulation [18,19]. In B. napus developing embryos, the Rubisco bypass metabolic route increased the carbon usage efficiency that yields 20% more acetyl-CoA for oil biosynthesis [33]. In soybean (Glycine max L.), broad bean (Vicia faba L.) and pea (Pisum sativum L.), which are also green seeds, the embryogenic photosynthesis contributed to their oxygen and energy supply and was coupled to oil biosynthetic pathways [34,35]. In order to develop in the limited space inside the seed, the plant embryo must adjust its shape and size, which simultaneously affects the photosynthetic activity of different parts of the embryo and establishes an oil deposition gradient within the B. napus embryo [36]. During B. napus seed development, the two important biological pathways of oil anabolism and photosynthesis are co-regulated by the key oil content regulatory gene BnWRI1 [3]. Heat stress during seed filling stage would irreversibly damage the seed photosystem and up-regulated the genes of photoinhibition pathway, which inhibited the photosynthetic activity of B. napus developing seed. Meanwhile, heat stress down-regulated the transcription factor BnWRI1, thus inhibiting the oil biosynthesis pathway. Furthermore, seed-specific overexpression of BnWRI1 in B. napus up-regulated the expression of genes related to fatty acid biosynthesis and photosynthesis pathway under both suitable and heat stress conditions, which not only significantly increased seed oil content under normal temperature, but also stabilized B. napus seed oil accumulation and photosynthetic activity under heat stress [14]. In B. napus exposure to heat stress, the stability of oil content and the maintenance of seed photosynthetic activity was intrinsically related [14].
FV/FM was the widely used parameter for determination of the photosystems damage caused by stress. The phenotype by Fv/Fm had been used to identification of heat tolerant in wheat [37]. In seed of B. napus, the relationship between heat stress and Fv/Fm reduction was also established [14]. In the present survey, the effects of heat stress on chlorophyll fluorescence characteristics of B. napus seeds PSII were analyzed. Evidences that the Fv/Fm, ETR and Y(II) for heat-stressed developing seeds of each assayed B. napus accessions were significantly reduced,suggested that the processes of light energy capture, the Calvin cycle and the photosynthetic electron transport were all inhibited by heat stress. A similar thermal damage of PSII was reported for leaves of heat stressed C3 sunflower and C4 maize [26]. By pairwise comparison, it was found that the sensitivity of these chlorophyll fluorescence parameters to heat stress was consistent with the heat sensitivity at seed filling stage of the B. napus germplasms (Table 1; Fig. 2). The photosynthesis of the developing seed itself provided energy and reductant for fatty acid biosynthesis [17–19]. The results of this study further indicated that the decrease of seed photosynthetic activity might be one of the important reasons of heat stress affecting B. napus seed oil content. Investigating the changes of photosynthetic physiology in heat-stressed B. napus developing seeds was helpful to improve the accuracy of heat tolerance selection during seed filling stage.
The main production purpose of rapeseed is to harvest seeds. So it is urgent to establish a method for screening and identification of B. napus heat tolerance during seed filling. Due to the tallness and large size of rapeseed plants, how to carry out large-scale and repeatable heat-stress treatment in seed filling stage under controllable conditions has always been an obstacle to the selection of heat-tolerant B. nupus germplasms. In this study, the in vitro silique culture system, the method for analysis of metabolic flux under defined conditions [14], was used to investigate the effects of heat stress on B. napus developing seed oil accumulation. We showed that the decrease rates of seed oil content or oil per seed in developing seeds isolated from heat-stressed siliques could be used to evaluate the B. napus heat sensitivity at seed filling stage. The evaluation results are similar to the identification results which using the decrease rate of mature seeds oil content or decrease rate of oil per plant in whole plant heat-stressed B. napus as evaluating indicators. This facilitated the efficient and accurate comparison of the B. napus heat tolerance during seed filling. Combined with the modulated chlorophyll fluorescence image analysis technology, which allows bulk comparison the changes of photosynthetic characteristics in heat-stressed seed of different B. napus accessions at the same interface, and with reference to changes in important traits such as oil content of mature and immature seeds, oil yield per plant, and oil per seed, it was further showed that the decrease rate of seed Fv/Fm, ETR and Y(II) in heat-stressed silique could reflect the sensitivity of the tested rapeseed germplasms to heat stress during seed filling. Moreover, seed RFv/Fm could be used as a key index for screening and evaluating of B. napus heat tolerance at seed filling stage. This newly established system facilitated the comparison and identification of heat tolerance of B. napus germplasm.
Heat stress during seed filling inhibited oil accumulation and photosynthesis in B. napus developing seeds. Particularly, the chlorophyll fluorescence parameters such as Fv/Fm, the maximum photon yield of PSII reaction center, were reduced, and the decrease rates different significant among germplasms, indicating that heat damage of B. napus developing seed photosystem to varying degrees. It was found that there were extensive genetic variations in the responses of seed photosynthetic activity and oil content to heat stress at the seed filling stage. The effects of two heat stress methods, silique heat-stressed at seed oil rapidly deposition stage and whole plant heat-stressed during seed filling, had consistent influence trend on B. napus seed oil accumulation. Furthermore, the RFv/Fm of silique heat-stressed B. napus developing seed was correlated with the decrease rate of mature seed oil content of the whole plant heat-stressed rapeseed (R = 0.9214, P-value < 0.01), which could be used to predict the heat sensitivity of oil accumulation during B.napus seed filling stage. Finally two heat-tolerant B. napus germplasms with high oil content were screened out.
Acknowledgement: We thank Chunyun Guan and Zhongsong Liu (Hunan Agricultural University), Xinfa Wang (Oil Crop Research Institute of Chinese Academy of Agricultural Sciences), Jiana Li (Southwest University), Liangbin Lin (Yunnan Agricultural University) and Dongqing Zhang (Zhejiang Academy of Agricultural Sciences) for providing B. napus germplasms for this research.
Authorship: The authors confirm contribution to the paper as follows: study conception and design: RH, HY, HW.; data collection: RH, YY, HL, ZL, HH, WW; analysis and interpretation of results: RH, HY, HL, XW, GW, HW; draft manuscript preparation: RH, HL, XW, HW. All authors reviewed the results and approved the final version of the manuscript.
Funding Statement: This research was funded by the Natural Science Foundation of Zhejiang Province (LY20C130006), the National Natural Science Foundation of China (32172018) and the State Key Laboratory for Managing Biotic and Chemical Threats to the Quality and Safety of Agro-Products (2010DS700124-ZZ1805).
Conflicts of Interest: The authors declare that they have no conflicts of interest to report regarding the present study.
References
1. Hammac, W. A., Maaz, T. M., Koenig, R. T., Burke, I. C., Pan, W. L. (2017). Water and temperature stresses impact canola (Brassica napus L.) fatty acid, protein, and yield over nitrogen and sulfur. Journal of Agricultural and Food Chemistry, 65(48), 10429–10438. DOI 10.1021/acs.jafc.7b02778. [Google Scholar] [CrossRef]
2. Weselake, R. J., Shah, S., Tang, M. G., Quant, P. A., Snyder, C. L. (2008). Metabolic control analysis is helpful for informed genetic manipulation of oilseed rape (Brassica napus) to increase seed oil content. Journal of Experimental Botany, 59(13), 3543–3549. DOI 10.1093/jxb/ern206. [Google Scholar] [CrossRef]
3. Wu, X. L., Liu, Z. H., Hu, Z. H., Huang, R. Z. (2014). BnWRI1 coordinates fatty acid biosynthesis and photosynthesis pathways during oil accumulation in rapeseed. Journal of Integrative Plant Biology, 56(6), 582–593. DOI 10.1111/jipb.12158. [Google Scholar] [CrossRef]
4. Xiao, Z. C., Zhang, C., Tang, F., Yang, B., Zhang, L. Y. et al. (2019). Identification of candidate genes controlling oil content by combination of genome-wide association and transcriptome analysis in the oilseed crop. Brassica napus. Biotechnology for Biofuels, 12(1), 216. DOI 10.1186/s13068-019-1557-x. [Google Scholar] [CrossRef]
5. Zheng, X. T., Wang, C. L., Lin, W. X., Lin, C. F., Han, D. L. et al. (2022). Importation of chloroplast proteins under heat stress is facilitated by their SUMO conjugations. New Phytologist, 235(1), 173–187. DOI 10.1111/nph.18121. [Google Scholar] [CrossRef]
6. Zhou, L. H., Yan, T., Chen, X., Li, Z. L., Wu, D. Z. et al. (2018). Effect of high night temperature on storage lipids and transcriptome changes in developing seeds of oilseed rape. Journal of Experimental Botany, 69(7), 1721–1733. DOI 10.1093/jxb/ery004. [Google Scholar] [CrossRef]
7. Zhu, Y. N., Cao, Z. Y., Xu, F., Huang, Y., Chen, M. X. et al. (2012). Analysis of gene expression profiles of two near-isogenic lines differing at a QTL region affecting oil content at high temperatures during seed maturation in oilseed rape (Brassica napus L.). Theoretical and Applied Genetics, 124(3), 515–531. DOI 10.1007/s00122-011-1725-2. [Google Scholar] [CrossRef]
8. Zhou, W. J., Leul, M. (1999). Uniconazole-induced tolerance of rape plants to heat stress in relation to changes in hormonal levels, enzyme activities and lipid peroxidation. Plant Growth Regulation, 27, 99–104. [Google Scholar]
9. Ismaili, A., Salavati, A., Mohammadi, P. P. (2014). A comparative proteomic analysis of responses to high temperature stress in hypocotyl of canola (Brassica napus L.). Protein & Peptide Letters, 22(3), 285–299. DOI 10.2174/0929866521666141124102755. [Google Scholar] [CrossRef]
10. Dikšaitytė, A., Viršilė, A., Žaltauskaitė, J., Januškaitienė, I., Juozapaitienė, G. (2019). Growth and photosynthetic responses in Brassica napus differ during stress and recovery periods when exposed to combined heat, drought and elevated CO2. Plant Physiology and Biochemistry, 142(4), 59–72. DOI 10.1016/j.plaphy.2019.06.026. [Google Scholar] [CrossRef]
11. Yao, M., Guan, M., Yang, Q., Huang, L. Y., Xiong, X. H. et al. (2021). Regional association analysis coupled with transcriptome analyses reveal candidate genes affecting seed oil accumulation in Brassica napus. Theoretical and Applied Genetics, 134(5), 1545–1555. DOI 10.1007/s00122-021-03788-0. [Google Scholar] [CrossRef]
12. Dubas, E., Moravčíková, J., Libantová, J., Matušíková, I., Benková, E. et al. (2014). The influence of heat stress on auxin distribution in transgenic B. napus microspores and microspore-derived embryos. Protoplasma, 251(5), 1077–1087. DOI 10.1007/s00709-014-0616-1. [Google Scholar] [CrossRef]
13. Yu, E. R., Fan, C. C., Yang, Q. Y., Li, X. D., Wan, B. X. et al. (2014). Identification of heat responsive genes in Brassica napus siliques at the seed-filling stage through transcriptional profiling. PLoS One, 9(7), e101914. DOI 10.1371/journal.pone.0101914. [Google Scholar] [CrossRef]
14. Huang, R. Z., Liu, Z. H., Xing, M. Q., Yang, Y., Wu, X. L. et al. (2019). Heat stress suppresses Brassica napus seed oil accumulation by inhibition of photosynthesis and BnWRI1 pathway. Plant & Cell Physiology, 60(7), 1457–1470. DOI 10.1093/pcp/pcz052. [Google Scholar] [CrossRef]
15. Yan, G. X., Lv, X. D., Gao, G. Z., Li, F., Li, J. et al. (2016). Identification and characterization of a Glyoxalase I gene in a rapeseed cultivar with seed thermotolerance. Frontiers in Plant Science, 7, 150. DOI 10.3389/fpls.2016.00150. [Google Scholar] [CrossRef]
16. Gao, G. Z., Li, J., Li, H., Li, F., Xu, K. et al. (2014). Comparison of the heat stress induced variations in DNA methylation between heat-tolerant and heat-sensitive rapeseed seedlings. Breeding Science, 64(2), 125–133. DOI 10.1270/jsbbs.64.125. [Google Scholar] [CrossRef]
17. Lee, J. S., Adams, K. L. (2020). Global insights into duplicated gene expression and alternative splicing in polyploid Brassica napus under heat, cold, and drought stress. Plant Genome, 13(3), e20057. DOI 10.1002/tpg2.20057. [Google Scholar] [CrossRef]
18. Ruuska, S. A., Schwender, J., Ohlrogge, J. B. (2004). The capacity of green oilseeds to utilize photosynthesis to drive biosynthetic processes. Plant Physiology, 136(1), 2700–2709. DOI 10.1104/pp.104.047977. [Google Scholar] [CrossRef]
19. Goffman, F. D., Alonso, A. P., Schwender, J., Shachar-Hill, Y., Ohlrogge, J. B. (2005). Light enables a very high efficiency of carbon storage in developing embryos of rapeseed. Plant Physiology, 138(4), 2269–2279. DOI 10.1104/pp.105.063628. [Google Scholar] [CrossRef]
20. Molina, I., Ohlrogge, J. B., Pollard, M. (2008). Deposition and localization of lipid polyester in developing seeds of Brassica napus and Arabidopsis thaliana. The Plant Journal, 53(3), 437–449. DOI 10.1111/j.1365-313X.2007.03348.x. [Google Scholar] [CrossRef]
21. Chen, S. T., He, N. Y., Chen, J. H., Guo, F. Q. (2016). Identification of core subunits of photosystem II as action sites of HSP21 that is activated by the GUN5-mediated retrograde pathway in Arabidopsis. The Plant Journal, 89(6), 1106–1118. DOI 10.1111/tpj.13447. [Google Scholar] [CrossRef]
22. Zhong, L. L., Zhou, W., Wang, H. J., Ding, S. H., Lu, Q. T. et al. (2013). Chloroplast small heat shock protein HSP21 interacts with plastid nucleoid protein pTAC5 and is essential for chloroplast development in Arabidopsis under heat stress. Plant Cell, 25(8), 2925–2943. DOI 10.1105/tpc.113.111229. [Google Scholar] [CrossRef]
23. Tan, W., Meng, Q. W., Brestic, M., Olsovska, K., Yang, X. H. (2011). Photosynthesis is improved by exogenous calcium in heat-stressed tobacco plants. Journal of Plant Physiology, 168(17), 2063–2071. DOI 10.1016/j.jplph.2011.06.009. [Google Scholar] [CrossRef]
24. Qiu, Z. M., Kang, S. J., He, L., Zhao, J., Zhang, S. et al. (2018). The newly identified heat-stress sensitive albino 1 gene affects chloroplast development in rice. Plant Science, 267, 168–179. DOI 10.1016/j.plantsci.2017.11.015. [Google Scholar] [CrossRef]
25. Sghaier, D. B., Bankaji, I., Pedro, S., Caçador, I., Sleimi, N. (2019). Photosynthetic behaviour and mineral nutrition of Tamarix gallica cultivated under aluminum and NaCl combined stress. Phyton-International Journal of Experimental Botany, 88(3), 239–252. DOI 10.32604/phyton.2019.06887. [Google Scholar] [CrossRef]
26. Killi, D., Raschi, A., Bussotti, F. (2020). Lipid peroxidation and chlorophyll fluorescence of photosystem II performance during drought and heat stress is associated with the antioxidant capacities of C3 sunflower and C4 maize varieties. International Journal of Molecular Sciences, 21(14), 4846. DOI 10.3390/ijms21144846. [Google Scholar] [CrossRef]
27. He, X., Liu, W., Li, W. Q., Liu, Y., Wang, W. P. et al. (2020). Genome-wide identification and expression analysis of CaM/CML genes in Brassica napus under abiotic stress. Journal of Plant Physiology, 255(6199), 153251. DOI 10.1016/j.jplph.2020.153251. [Google Scholar] [CrossRef]
28. Tan, H. L., Yang, X. H., Yang, F. X., Zheng, X., Qu, C. M. et al. (2011). Enhanced seed oil production in canola by conditional expression of Brassica napus LEAFY COTYLEDON1 and LEC1-LIKE in developing seeds. Plant Physiology, 156(3), 1577–1588. DOI 10.1104/pp.111.175000. [Google Scholar] [CrossRef]
29. Hua, W., Li, R. J., Zhan, G. M., Liu, J., Li, J. et al. (2012). Maternal control of seed oil content in Brassica napus: the role of silique wall photosynthesis. The Plant Journal, 69(3), 432–444. DOI 10.1111/j.1365-313X.2011.04802.x. [Google Scholar] [CrossRef]
30. Tang, S., Zhao, H., Lu, S. P., Yu, L. Q., Zhang, G. F. et al. (2021). Genome- and transcriptome- wide association studies provide insights into the genetic basis of natural variation of seed oil content in Brassica napus. Molecular Plant, 14(3), 470–487. DOI 10.1016/j.molp.2020.12.003. [Google Scholar] [CrossRef]
31. Hajduch, M., Casteel, J. E., Hurrelmeyer, K. E., Song, Z., Agrawal, G. K. et al. (2006). Proteomic analysis of seed filling in Brassica napus. Developmental characterization of metabolic isozymes using high-resolution two-dimensional gel electrophoresis. Plant Physiology, 141(1), 32–46. DOI 10.1104/pp.105.075390. [Google Scholar] [CrossRef]
32. Chen, F., Zhang, W., Yu, K., Sun, L., Gao, J. et al. (2018). Unconditional and conditional QTL analyses of seed fatty acid composition in Brassica napus L. BMC Plant Biology, 18(1), 49. DOI 10.1186/s12870-018-1268-7. [Google Scholar] [CrossRef]
33. Schwender, J., Goffman, F., Ohlrogge, J. B., Shachar-Hill, Y. (2004). Rubisco without the calvin cycle improves the carbon efficiency of developing green seeds. Nature, 432(7018), 779–782. DOI 10.1038/nature03145. [Google Scholar] [CrossRef]
34. Rolletschek, H., Weber, H., Borisjuk, L. (2003). Energy status and its control on embryogenesis of legumes. Embryo photosynthesis contributes to oxygen supply and is coupled to biosynthetic fluxes. Plant Physiology, 132(3), 1196–1206. DOI 10.1104/pp.102.017376. [Google Scholar] [CrossRef]
35. Rolletschek, H., Radchuk, R., Klukas, C., Schreiber, F., Wobus, U. et al. (2005). Evidence of a key role for photosynthetic oxygen release in oil storage in developing soybean seeds. New Phytologist, 167(3), 777–786. DOI 10.1111/j.1469-8137.2005.01473.x. [Google Scholar] [CrossRef]
36. Borisjuk, L., Neuberger, T., Schwender, J., Heinzel, N., Sunderhaus, S. et al. (2013). Seed architecture shapes embryo metabolism in oilseed rape. Plant Cell, 25(5), 1625–1640. DOI 10.1105/tpc.113.111740. [Google Scholar] [CrossRef]
37. Sharma, D., Torp, A., Rosenqvist, E., Ottosen, C., Andersen, S. (2017). QTLs and potential candidate cenes for heat stress tolerance identified from the mapping populations specifically segregating for Fv/Fm in wheat. Frontiers in Plant Science, 8, 1668. DOI 10.3389/fpls.2017.01668. [Google Scholar] [CrossRef]
Appendix
Figure S1: Temperature regime for heat stress of B. napus silique
Cite This Article
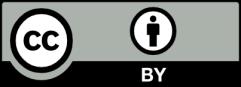
This work is licensed under a Creative Commons Attribution 4.0 International License , which permits unrestricted use, distribution, and reproduction in any medium, provided the original work is properly cited.