Open Access
REVIEW
Plant-Derived Enzymes Producing Chiral Aroma Compounds and Potential Application
1 Guangdong Food and Drug Vocational College, Guangzhou, 510520, China
2 National Navel Orange Engineering Research Center, College of Life Sciences, Gannan Normal University, Ganzhou, 341000, China
3 Tropical Agriculture and Forestry Department, Guangdong AIB Polytechnic College, Guangzhou, 510650, China
4 Key Laboratory of South China Agricultural Plant Molecular Analysis and Genetic Improvement & Guangdong Provincial Key Laboratory of Applied Botany, South China Botanical Garden, Chinese Academy of Sciences, Guangzhou, 510650, China
* Corresponding Authors: Xinguo Su. Email: ; Lanting Zeng. Email:
(This article belongs to the Special Issue: Biotechnology of Plant Secondary Metabolites)
Phyton-International Journal of Experimental Botany 2023, 92(2), 383-398. https://doi.org/10.32604/phyton.2022.023185
Received 14 April 2022; Accepted 30 May 2022; Issue published 12 October 2022
Abstract
Aroma (volatile) compounds play important ecological functions in plants, and also contribute to the quality of plant-derived foods. Moreover, chiral aroma compounds affect their functions in plants and lead to different flavor quality properties. Formations of chiral aroma compounds are due to the presence of enzymes producing these compounds in plants, which are generally involved in the final biosynthetic step of the aroma compounds. Here, we review recent progress in research on the plant-derived enzymes producing chiral aroma compounds, and their changes in response to environmental factors. The chiral aroma enzymes that have been reported produce (R)-linalool, (S)-linalool, (R)-limonene, and (S)-limonene, etc., and these enzymes are found in various plant species. We also discuss the origins of enantioselectivity in the plant-derived enzymes producing chiral aroma compounds and summarize the potential use of plants containing enzymes producing chiral aroma compounds for producing chiral flavors/fragrances.Keywords
1 Chiral Aroma Compounds and Enzymes Producing Chiral Aroma Compounds in Plants
A number of aroma compounds with different enantioforms have been identified in plants. These can be divided into different categories, including volatile terpenes, volatile phenylpropanoids/benzenoids, and volatile fatty acid derivatives, based on their different metabolic pathways. The most common chiral aroma compounds described in the literature are volatile terpenes, such as dihydro-α-ionone, dihydro-γ-ionone, α-ionone, γ-ionone, linalool, linalool oxide, and α-terpineol [1–8].
Among the chiral aroma compounds, ionones and lactones generally have lower olfactory detection limits (ng/L level) [1,9]; and different enantioforms have different sensory and olfactory detection limits [2]. In addition, the fragrance delicately varies from isomer to isomer [2]. For example, (S)-(+)-γ-ionone has floral, green, and woody sensory properties, and has an olfactory detection limit of 0.07 ng/L, while (R)-(-)-γ-ionone possesses weak green, fruity, and pineapple-like properties, and has an olfactory detection limit of 11 ng/L [1,2]. Moreover, (1S, 2S)-methyl jasmonate and its (1S, 2R)-isomer are odorless, while their (1R) isomers show typical floral, jasminic, slightly fruity properties, and have low detection limits [2,10]. In this review, Table 1 summarizes the sensory properties and olfactory detection limits of some chiral aroma compounds which are widely distributed in a diverse range of plants. Moreover, chiral aroma compounds occur in different plants with different enantioform ratios. In some plants, some chiral aroma compounds reach 100% enantioform ratio. Enantiomeric distributions of linalool in major plant essential oil sources have been reviewed previously [11,12]. Linalool is found in the essential oils of over 200 monocotyledonous and dicotyledonous plant species, accounting for more than 50% of plant families [12,13]. Over 90% of (R)-linalool is found in Cinnamomum camphora Nees & Eberm var. linaloolifera, and Aniba rosaeodora Ducke [14], while over 80% of (S)-linalool distributes in Orthodon linalooliferum Fujita and Coriandrum sativum L. [12]. Overall, (R)-linalool is more common in nature, compared with (S)-linalool [12]. In practical terms, the true assessment of the ratio of chiral aroma compounds may be affected by different chemical structures, vegetal matrix, processing methods and analytical techniques [12]. Therefore, it is important to study the enantiomeric distributions in plants themselves, not in the oils or flavor sources. In fact, the methods have been established to avoid the interference of extraction and analysis. Organic reagents, such as dichloromethane, ethyl acetate, were used in the extraction of chiral aroma compounds at room temperature (nearly 25°C). After the extraction, the samples were subjected to the gas chromatography–mass spectrometry analysis.
The enantiomeric distributions of linalool have been investigated in plants themselves, especially in flowers, e.g., in flower scents [21–23]. In this review, reports of chiral aroma compounds investigated only in plants themselves are summarized in Table 2. Consistent with the studies in oil sources, linalool is also the most widely studied in plants. Dötterl et al. investigated the flower scent of 15 plant species and found that 5 plant species only contain one isomer of linalool, i.e., (S)-linalool [21]. Furthermore, the enantiomeric composition of linalool in the flowers of kiwifruit (Actinidia) species has also been thoroughly investigated [23]. It was found that floral linalool from some species were not consistent inter-species enantiomeric ratios [23]. Chiral aroma compounds jasmine lactone and 1-phenylethanol have been reported in Camellia sinensis [24–26]. Ninety-two percent of (S)-jasmine lactone is distributed in C. sinensis, which is nearly the same as the amount of (S)-jasmine lactone found in an oil made from Mangifera indica [16]. An interesting phenomenon has been observed in C. sinenesis; the chiral ratio of the emitted 1-phenylethanol is different from that of the internal 1-phenylethanol [25,26].
In contrast to identification of aroma compounds with different configurations in plants, very little information on the aroma synthases which produce these aroma compounds is available. The aroma enzymes that have been reported produce (R)-linalool, (S)-linalool, (R)-limonene, and (S)-limonene, and these enzymes are found in various plant species (Table 3) [22,30,33–35]. There is more than one enzyme responsible for the biosynthesis of each aroma compound isomer in a plant species, such as Fragaria ananassa and Citrus unshiu [30,33]. The co-occurrence of two enantiomers is a common feature in nature. For example, Arabidopsis thaliana is reported to produce both (R)-linalool and (S)-linalool. Gene coexpression analysis revealed the complex metabolism of linalool in Arabidopsis flowers, and revealed that there are two TPS enzymes that form the two linalool enantiomers [34].
2 Influence of Environment Factors on the Plant-Derived Enzymes Producing Chiral Aroma Compounds
There are many reports concerning the effects of environmental factors, including biotic and abiotic factors, on plant volatiles and their formation-related enzymes. In most cases, the environmental factors activate phytophormone synthesis, which affects the genes encoding plant volatile synthases, and thus changes in plant volatile synthesis and emission [50,51]. As very few enzymes producing chiral aroma compounds have been identified and functionally characterized in plants, less is known about the influences of environmental factors on the plant-derived enzymes producing chiral aroma compounds. Pragadheesh et al. [22] investigated changes in linalool enantiomers at various developmental stages of Jasminum grandiflorum flowers. Linalool predominated as the (R) form in floral buds, whereas (S)-linalool was the major enantiomer found in the mature flowers. An (R)-linalool synthase gene was isolated and identified from J. grandiflorum flowers, and its expression correlated well with the high (R)-linalool emission at the bud stage. The change in the enantiomeric ratio from the (R)- to (S)-linalool moving from the bud to the mature stage may be due to the enantiospecific transformation and temporal decline of the (R)-linalool synthase gene in J. grandiflorum. We also investigated the effects of abiotic factors, such as mechanical damage and temperature, on stereochemical configurations of jasmine lactone in tea leaves (C. sinensis) [24]. (S)-Jasmine lactone was the major enantiomer, and it was found that different treatments affected the enantiomeric ratio of jasmine lactone in tea leaves. Under continuous mechanical damage, the ratio of (R) to (S) jasmine lactone was 23:77, but the ratio became 8:92 in tea leaves exposed to a combination of mechanical damage and low temperature (15°C). As the enzymes which produce (R) or (S)-jasmine lactone are unknown in tea leaves, it remains to be determined if the genes encoding (R) or (S)-jasmine lactone synthases are affected by these abiotic factors.
There have been much fewer reports on the effects of biotic factors, such as insect attacks, on the stereochemical configurations of volatiles from vegetative or floral plant parts, although it is well known that insect visits/attacks change the quantity of volatiles emitted from these parts. An attack by the insect Thrips hawaiiensis (Morgan) was found to induce changes in the (R)-/(S)-1-phenylethanol ratio emitted from tea flowers [26]. In addition, the proportion of jasmonic acid (JA) was increased by the T. hawaiiensis attack. Interestingly, exposing flowers to T. hawaiiensis attack induced an (R)-/(S)-1-phenylethanol pattern that was similar to that emitted from flowers after exogenous JA treatment, suggesting that JA may be involved in the insect-induced changes in the (R)-/(S)-1-phenylethanol ratio [26].
3 Origins of Stereoselectivity in the Plant-Derived Enzymes Producing Chiral Aroma Compounds
Terpene synthases are a family of aroma synthases, which produce volatile terpene compounds with different stereoselectivities. Köllner et al. reported the stereoselectivity origins of two closely related maize terpene synthases encoding multiple stereoselective product enzymes [52]. The two terpene synthases in maize, TPS4 and TPS5, were found to produce volatile sesquiterpenes from the precursor farnesyl diphosphate, but with different proportions and stereoselectivity of products. TPS4 and TPS 5 share 98% identity at the amino acid level, and such high sequence similarity made it possible to determine which amino acid residues were responsible for the different stereoselectivity of products using site-directed mutagenesis. Four amino acids (positions 407, 409, 410, and 411) were different between TPS4 and TPS5, and these were located at the bottom of the active site cavity. Amino acid exchange experiments suggested that these amino acids located in the catalytic center determine the stereoselectivity of TPS4 and TPS5. In contrast to volatile sesquiterpene synthases, the origins of the volatile monoterpene synthase stereoselectivity are still unclear. Ginglinger et al. reported that the two TPS enzymes in Arabidopsis flowers, TPS10 and TPS14, can produce (-)-(R)-linalool and (+)-(S)-linalool, respectively [34]. However, phylogenetic analysis shows that the two TPSs are not closed class and belong to different clusters of TPS enzymes [53]. Therefore, their abilities to produce linalool may have evolved independently [34].
Ketoreductases are a family of enzymes which can reduce a wide range of ketones to alcohols with different stereoselectivities. These are the most commonly used enzymes for the manufacture of chiral alcohols in industrial pharmaceutical synthesis [54]. The stereoselectivity origins of plant-derived ketoreductases have not been reported, but there may be some hints from the reported findings in microorganisms. Recently, Noey et al. reported origins of stereoselectivity in evolved ketoreductases that reduce almost-symmetrical 3-oxacyclopentanone and 3-thiacyclopentanone in Lactobacillus kefir [55]. Certain point mutations in the active site, such as A94F and Y190F, lead to conformational variations in the active site that enlarge the small binding pocket, so that the larger S atom becomes easier to accommodate, and thus S-selectivity with 3-thiacyclopentanone is increased. In contrast, E145S shrinks the small binding pocket and amplifies the difference in size between an S atom and a CH2 group. Shrinking the small binding pocket promotes R-selectivity, and also destabilizes the pro-S orientation.
4 Potential Use of the Plants Containing Enzymes Producing Chiral Aroma Compounds for Producing Chiral Flavors/Fragrances
In recent years, biocatalysis, especially plant-derived enzymatic catalysis, has attracted increased interest for the preparation of chiral alcohols. In contrast to a chemical synthesis approach, biocatalysis using plants has several advantages, including being environmentally-friendly, simple procedures, low cost, high efficiency, and excellent enantioselectivity [56]. In addition, plant extracts contain the oxido-reductase, cofactor (NAD(P)H) and its regeneration system, meaning that the enzyme-catalyzed process avoids the addition of the expensive cofactor [57]. So far, carrot root (Daucus carota), apple (Malus pumila), cucumber (Cucumis sativus), onion (Allium cepa), potato (Solanum tuberosum), radish (Raphanus sativus), sweet potato (Ipomoea batatas), tea flowers (C. sinensis), clementine mandarin fruit (Citrus reticulate), strawberry tree (Arbutus unedo L.), and ginger roots (Zingiber officinale) have been reported for the production of chiral alcohols [25,56–61]. Among these plant resources, carrot root is the most frequently reported.
All the plant tissues should be used fresh to keep the enzymes active. The fresh plant tissues are simply extracted by water or buffer or directly used for the bioreductions. The plant-derived enzymes have a wide substrate-selectivity, and they not only catalyze the substrates present in the source plant itself to produce chiral alcohols but also are functional with substrates that may not occur in the source plant. Excellent yields and high enantioselectivities are the most important evaluation indices for biocatalysts. Low cost or abundant waste plant resources, such as strawberry trees and tea flowers, are being investigated as materials for industrial large-scale production [25,57]. Different plant resources have different substrate selectivities and enantioselectivities (Table 4), and each plant resource can form products with different enantioselectivity when using different substrates. The plant’s geographical location, growing environment, and seasonal variation may result in the same plant resource catalyzing a substrate to give products with different enantioselectivities. Currently, plant tissues are mostly used to transform ketones to different chiral alcohols, while less is known about the potential for these plant tissues to be used for the production of other types of chiral aromas, not derived from ketones. As crude plant extracts are used for biocatalysis, there is a high likelihood of producing many by-products, which may cause issues for isolation and purification of the target products.
5 Concluding Remarks and Perspectives
In this review, we have summarized the current knowledge of plant-derived enzymes producing chiral aroma compounds, their changes in response to environmental factors, origins of stereoselectivity in the plant-derived enzymes producing chiral aroma compounds, and the potential use of these enzymes for the stereoselective synthesis of important flavors/fragrances. The huge variety of enzymes producing chiral aroma compounds in different plants and their complex catalysis properties suggest that there is still much to learn. Several important points should be addressed in future studies:
(1) Authentic standards of aroma compounds with different stereoselectivities are not available to buy, but are usually synthesized by the researchers. Establishing a database detailing synthetic methods and identification information of the known aroma compounds with different stereoselectivities will be very helpful for characterization of the aroma synthases.
(2) Current research has shown which plants contain which type of enzymes producing chiral aroma compounds. However, it is still unknown which exact enzyme is responsible for the production of chiral aroma compounds. Some plants may contain more than one particular enzymes with stereoselectivity. Identification of the exact enzymes and their subcellular locations will be helpful for us to understand their occurrences in plants.
(3) Not all plants contain enzymes producing chiral aroma compounds. Why do some enzymes producing chiral aroma compounds occur in many plants and some others are only present in certain plants? Why do some enzymes form products with different enantioselectivities with different substrates? In addition, what are the origins of stereoselectivity in the plant-derived enzymes producing chiral aroma compounds? Based on protein 3D structure experimental and computational theoretical studies, more direct evidence is needed in plants, and not simply by referring from microorganisms.
(4) For the future application of plant-derived enzymes for the stereoselective synthesis of important flavors/fragrances, several issues should be addressed. These include: establishing standard quality control for plant materials, reasonable design of plant resources as biocatalysts, and sequential design of multiple processes with sample pre-treatment, biocatalysis, extraction, isolation, and purification of the chiral target products.
(5) The directions of further studies will establish reliably the enantiomeric composition of aromatic compounds. In addition, an important point should be the study of the role of aroma synthases and their complex catalysis properties for the production of chiral aroma compounds.
Authorship: The authors confirm contribution to the paper as follows: constructed the manuscript outline: Lanting Zeng; wrote the manuscript: Fang Dong, Qian Fan, Xinguo Su and Lanting Zeng. All authors reviewed and approved the final version of the manuscript.
Funding Statement: A part of the research aspects done by the authors was supported by the financial supports from the Guangdong Basic and Applied Basic Research Foundation (2020A1515010539), the Medical Science and Technology Research Foundation of Guangdong Province, China (A2019046), Guangdong Province Higher Vocational Colleges & Schools Pearl River Scholar (50117G25002), and the Key Project of Universities in Guangdong Province (2021ZDZX4066). Because of space limitations, we could not cite all publications in the field; we apologize to all colleagues whose work has not been mentioned.
Conflicts of Interest: The authors declare that they have no conflicts of interest to report regarding the present study.
References
1. Brenna, E., Fuganti, C., Serra, S., Kraft, P. (2002). Optically active ionones and derivatives: Preparation and olfactory properties. European Journal of Organic Chemistry, 6, 967–978. DOI 10.1002/(ISSN)1099-0690. [Google Scholar] [CrossRef]
2. Brenna, E., Fuganti, C., Serra, S. (2003). Enantioselective perception of chiral odorants. Tetrahedron Asymmetry, 14(1), 1–42. DOI 10.1016/S0957-4166(02)00713-9. [Google Scholar] [CrossRef]
3. Xu, H., Dai, Y. F., Qiu, S. Y., Sun, B. G., Zeng, X. Y. (2021). Distribution and quantification of 1, 2-propylene glycol enantiomers in baijiu. Foods, 10(12), 3039. DOI 10.3390/foods10123039. [Google Scholar] [CrossRef]
4. Padrayuttawat, A., Yoshizawa, T., Tamura, H., Tokunaga, T. (1997). Optical isomers and odor thresholds of volatile constituents in Citrus sudachi. Food Science and Technology Research, 3(4), 402–408. [Google Scholar]
5. Dongrnei, W., Kiyoshi, A., Kae Morita, K. K. (1994). Optical isomers of linalool and linalool oxides in tea aroma. Bioscience, Biotechnology, and Biochemistry, 58(11), 2050–2053. DOI 10.1271/bbb.58.2050. [Google Scholar] [CrossRef]
6. Rapp, A. (1990). Natural flavours of wine: Correlation between instrumental analysis and sensory perception. Fresenius Journal of Analytical Chemistry, 333(7), 777–785. DOI 10.1007/BF00322252. [Google Scholar] [CrossRef]
7. Boelens, M., Boelens, H., van Gemert, L. (1993). Sensory properties of optical isomers. Perfumer and Flavorist, 18(6), 1–16. [Google Scholar]
8. Cacho, J., Moncayo, L., Palma, J. C., Ferreira, V., Culleré, L. (2012). Characterization of the aromatic profile of the italia variety of Peruvian pisco by gas chromatography-olfactometry and gas chromatography coupled with flame ionization and mass spectrometry detection systems. Food Research International, 49(1), 117–125. DOI 10.1016/j.foodres.2012.07.065. [Google Scholar] [CrossRef]
9. Bartschat, D., Lehmann, D., Dietrich, A., Mosandl, A., Kaiser, R. (2010). Chiral compounds of essential oils XIX. 4-methyl-5-decanolide: Chirospecific analysis, structure and properties of the stereoisomers. Phytochemical Analysis, 6(3), 130–134. DOI 10.1002/(ISSN)1099-1565. [Google Scholar] [CrossRef]
10. Acree, T. E., Nishida, R., Fukami, H. (1985). Odor thresholds of the stereoisomers of methyl jasmonate. Journal of Agricultural Food Chemistry, 33(3), 425–427. DOI 10.1021/jf00063a025. [Google Scholar] [CrossRef]
11. Casabianca, H., Graff, J. B., Faugier, V., Fleig, F., Grenier, C. (1998). Enantiomeric distribution studies of linalool and linalyl acetate. A powerful tool for authenticity control of essential oils. Journal of High Resolution Chromatography, 21(2), 107–112. DOI 10.1002/(ISSN)1521-4168. [Google Scholar] [CrossRef]
12. Aprotosoaie, A. C., Hăncianu, M., Costache, I. I., Miron, A. (2014). Linalool: A review on a key odorant molecule with valuable biological properties. Flavour and Fragrance Journal, 29(4), 193–219. DOI 10.1002/ffj.3197. [Google Scholar] [CrossRef]
13. Stashenko, E. E., Martínez, J. R. (2008). Sampling flower scent for chromatographic analysis. Journal of Separation Science, 31(11), 2022–2031. DOI 10.1002/jssc.200800151. [Google Scholar] [CrossRef]
14. Sell, C. S. (2003). The chemistry of fragrances: From perfumer to consumer, 2nd edition, pp. 54–63. UK: Royal Society of Chemistry. [Google Scholar]
15. Zhu, J. C., Zhu, Y., Wang, K., Niu, Y. W., Xiao, Z. B. (2021). Characterization of key aroma compounds and enantiomer distribution in Longjing tea. Food Chemistry, 361, 130096. DOI 10.1016/j.foodchem.2021.130096. [Google Scholar] [CrossRef]
16. Werkhoff, P., Brennecke, S., Bretschneider, W., Güntert, M., Hopp, R. et al. (1993). Chirospecific analysis in essential oil, fragrance and flavor research. Zeitschrift für Lebensmittel-Untersuchung und Forschung, 196(4), 307–328. DOI 10.1007/BF01197930. [Google Scholar] [CrossRef]
17. Yamamoto, T., Matsuda, H., Utsumi, Y., Hagiwara, T., Kanisawa, T. (2002). Synthesis and odor of optically active rose oxide. Tetrahedron Letters, 43(50), 9077–9080. DOI 10.1016/S0040-4039(02)02311-0. [Google Scholar] [CrossRef]
18. Zhu, Y., Yan, H., Zhang, Z. F., Zeng, J. M., Zhang, Y. et al. (2021). Assessment of the contribution of chiral odorants to aroma property of baked green teas using an efficient sequential stir bar sorptive extraction approach. Food Chemistry, 365, 130615. DOI 10.1016/j.foodchem.2021.130615. [Google Scholar] [CrossRef]
19. Friedman, L., Miller, J. G. (1971). Odor incongruity and chirality. Science, 172(3987), 1044–1046. DOI 10.1126/science.172.3987.1044. [Google Scholar] [CrossRef]
20. Fráter, G., Müller, U., Kraft, P. (1999). Preparation and olfactory characterization of the enantiomerically pure isomers of the perfumery synthetic Galaxolide®. Helvetica Chimica Acta, 82(10), 1656–1665. DOI 10.1002/(ISSN)1522-2675. [Google Scholar] [CrossRef]
21. Dötterl, S., Burkhardt, D., Weißbecker, B., Jürgens, A., Schütz, S. et al. (2006). Linalool and lilac aldehyde/alcohol in flower scents: Electrophysiological detection of lilac aldehyde stereoisomers by a moth. Journal of Chromatography A, 1113(1), 231–238. [Google Scholar]
22. Pragadheesh, V. S., Chanotiya, C. S., Rastogi, S., Shasany, A. K. (2017). Scent from Jasminum grandiflorum flowers: Investigation of the change in linalool enantiomers at various developmental stages using chemical and molecular methods. Phytochemistry, 140, 83–94. DOI 10.1016/j.phytochem.2017.04.018. [Google Scholar] [CrossRef]
23. Matich, A. J., Bunn, B. J., Hunt, M. B. (2010). The enantiomeric composition of linalool and linalool oxide in the flowers of kiwifruit (Actinidia) species. Chirality, 22(1), 110–119. DOI 10.1002/chir.20713. [Google Scholar] [CrossRef]
24. Zeng, L. T., Zhou, Y., Fu, X. M., Liao, Y. Y., Yuan, Y. F. et al. (2018). Biosynthesis of jasmine lactone in tea (Camellia sinensis) leaves and its formation in response to multiple stresses. Journal of Agricultural Food Chemistry, 66(15), 3899–3909. DOI 10.1021/acs.jafc.8b00515. [Google Scholar] [CrossRef]
25. Dong, F., Zhou, Y., Zeng, L. T., Watanabe, N., Su, X. G. et al. (2017). Optimization of production of 1-phenylethanol using enzymes from flowers of tea (Camellia sinensis) plants. Molecules, 22(1), 131. DOI 10.3390/molecules22010131. [Google Scholar] [CrossRef]
26. Zhou, Y., Zeng, L. T., Liao, Y. Y., Dong, F., Peng, Q. Y. et al. (2017). Insect (Thrips hawaiiensis (Morgan)) change the stereochemical configuration of 1-phenylethanol emitted from tea (Camellia sinensis) flowers. RSC Advances, 7 (51), 32336–32343. DOI 10.1039/C7RA03219F. [Google Scholar] [CrossRef]
27. Chen, F., Tholl, D., D’Auria, J. C., Farooq, A., Pichersky, E. et al. (2003). Biosynthesis and emission of terpenoid volatiles from Arabidopsis flowers. The Plant Cell, 15(2), 481–494. DOI 10.1105/tpc.007989. [Google Scholar] [CrossRef]
28. Parachnowitsch, A., Burdon, R. C. F., Raguso, R. A., Kessler, A. (2013). Natural selection on floral volatile production in Penstemon digitalis: Highlighting the role of linalool. Plant Signaling & Behavior, 8(1), e22704. DOI 10.4161/psb.22704. [Google Scholar] [CrossRef]
29. Kreck, M., Püschel, S., Wüst, M., Mosandl, A. (2003). Biogenetic studies in Syringa vulgaris L.: Synthesis and bioconversion of deuterium-labeled precursors into lilac aldehydes and lilac alcohols. Journal of Agricultural Food Chemistry, 51(2), 463–469. [Google Scholar]
30. Aharoni, A., Giri, A. P., Verstappen, F. W. A., Bertea, C. M., Sevenier, R. et al. (2004). Gain and loss of fruit flavor compounds produced by wild and cultivated strawberry species. The Plant Cell, 16(11), 3110–3131. DOI 10.1105/tpc.104.023895. [Google Scholar] [CrossRef]
31. Hong, J. H., Khan, N., Jamila, N., Hong, Y. S., Nho, E. Y. et al. (2017). Determination of volatile flavour profiles of Citrus spp. fruits by SDE-GC–MS and enantiomeric composition of chiral compounds by MDGC–MS. Phytochemical Analysis, 28(11), 392–403. DOI 10.1002/pca.2686. [Google Scholar] [CrossRef]
32. Machynakova, A., Khvalbota, L., Spanik, I. (2021). Enantiomer distribution of major chiral volatile organic compounds in botrytized grapes and wines. European Food Research and Technology, 247(9), 2321–2331. DOI 10.1007/s00217-021-03792-0. [Google Scholar] [CrossRef]
33. Shimada, T., Endo, T., Fujii, H., Hara, M., Ueda, T. et al. (2004). Molecular cloning and functional characterization of four monoterpene synthase genes from Citrus unshiu Marc. Plant Science, 166(1), 49–58. DOI 10.1016/j.plantsci.2003.07.006. [Google Scholar] [CrossRef]
34. Ginglinger, J. F., Boachon, B., Höfer, R., Paetz, C., Köllner, T. G. et al. (2013). Gene coexpression analysis reveals complex metabolism of the monoterpene alcohol linalool in Arabidopsis flowers. The Plant Cell, 25(11), 4640–4657. DOI 10.1105/tpc.113.117382. [Google Scholar] [CrossRef]
35. Landmann, C., Fink, B., Festner, M., Dregus, M., Engel, K. H. et al. (2007). Cloning and functional characterization of three terpene synthases from lavender (Lavandula angustifolia). Archives of Biochemistry and Biophysics, 465(2), 417–429. DOI 10.1016/j.abb.2007.06.011. [Google Scholar] [CrossRef]
36. van Schie, C. C. N., Haring, M. A., Schuurink, R. C. (2007). Tomato linalool synthase is induced in trichomes by jasmonic acid. Plant Molecular Biology, 64, 251–263. DOI 10.1007/s11103-007-9149-8. [Google Scholar] [CrossRef]
37. Crowell, A. L., Williams, D. C., Davis, E. M., Wildung, M. R., Croteau, R. (2002). Molecular cloning and characterization of a new linalool synthase. Archives of Biochemistry and Biophysics, 405, 112–121. DOI 10.1016/S0003-9861(02)00348-X. [Google Scholar] [CrossRef]
38. Iijima, Y., Gang, D. R., Fridman, E., Lewinsohn, E., Pichersky, E. (2004). Characterization of geraniol synthase from the peltate glands of sweet basil. Plant Physiology, 134, 370–379. DOI 10.1104/pp.103.032946. [Google Scholar] [CrossRef]
39. Zhou, Y., Deng, R. F., Xu, X. L., Yang, Z. Y. (2020). Enzyme catalytic efficiencies and relative gene expression levels of (R)-linalool synthase and (S)-linalool synthase determine the proportion of linalool enantiomers in Camellia sinensis var. sinensis. Journal of Agricultural and Food Chemistry, 68(37), 10109–10117. DOI 10.1021/acs.jafc.0c04381. [Google Scholar] [CrossRef]
40. Nagegowda, D. A., Gutensohn, M., Wilkerson, C. G., Dudareva, N. (2008). Two nearly identical terpene synthases catalyze the formation of nerolidol and linalool in snapdragon flowers. The Plant Journal, 55, 224–239. DOI 10.1111/j.1365-313X.2008.03496.x. [Google Scholar] [CrossRef]
41. Yuan, J. S., Köllner, T. G., Wiggins, G., Grant, J., Degenhardt, J. et al. (2008). Molecular and genomic basis of volatile-mediated indirect defense against insects in rice. The Plant Journal, 55, 491–503. DOI 10.1111/j.1365-313X.2008.03524.x. [Google Scholar] [CrossRef]
42. Maruyama, T., Saeki, D., Ito, M., Honda, G. (2002). Molecular cloning, functional expression and characterization of d-limonene synthase from Agastache rugosa. Biological & Pharmaceutical Bulletin, 25, 661–665. DOI 10.1248/bpb.25.661. [Google Scholar] [CrossRef]
43. Lucker, J., El Tamer, M. K., Schwab, W., Verstappen, F. W. A., van der Plas, L. H. W. et al. (2002). Monoterpene biosynthesis in lemon (Citrus limon)–cDNA isolation and functional analysis of four monoterpene synthases. European Journal of Biochemistry, 269, 3160–3171. DOI 10.1046/j.1432-1033.2002.02985.x. [Google Scholar] [CrossRef]
44. Shimada, T., Endo, T., Fujii, H., Hara, M., Omura, M. (2005a). Isolation and characterization of (E)-beta-ocimene and 1, 8 cineole synthases in Citrus unshiu Marc. Plant Science, 168, 987–995. DOI 10.1016/j.plantsci.2004.11.012. [Google Scholar] [CrossRef]
45. Bohlmann, J., Steele, C. L., Croteau, R. (1997). Monoterpene synthases from grand fir (Abies grandis)–cDNA isolation, characterization, and functional expression of myrcene synthase, (-)-(4S)-limonene synthase, and (-)-(1S, 5S)-pinene synthase. Journal of Biological Chemistry, 272, 21784–21792. DOI 10.1074/jbc.272.35.21784. [Google Scholar] [CrossRef]
46. Martin, D. M., Bohlmann, J. (2004). Identification of Vitis vinifera (-)-α-terpineol synthase by in silico screening of full-length cDNA ESTs and functional characterization of recombinant terpene synthase. Phytochemistry, 65, 1223–1229. DOI 10.1016/j.phytochem.2004.03.018. [Google Scholar] [CrossRef]
47. Byun-McKay, A., Godard, K. A., Toudefallah, M., Martin, D. M., Alfaro, R. et al. (2006). Wound-induced terpene synthase gene expression in Sitka spruce that exhibit resistance or susceptibility to attack by the white pine weevil. Plant Physiology, 140, 1009–1021. DOI 10.1104/pp.105.071803. [Google Scholar] [CrossRef]
48. Arimura, G. I., Garms, S., Maffei, M., Bossi, S., Schulze, B. et al. (2008). Herbivore-induced terpenoid emission in Medicago truncatula: Concerted action of jasmonate, ethylene and calcium signaling. Planta, 227, 453–464. DOI 10.1007/s00425-007-0631-y. [Google Scholar] [CrossRef]
49. Zhou, Y., Peng, Q. Y., Zhang, L., Cheng, S. H., Zeng, L. T. et al. (2019). Characterization of enzymes specifically producing chiral flavor compounds (R)- and (S)-1-phenylethanol from tea (Camellia sinensis) flowers. Food Chemistry, 280, 27–33. DOI 10.1016/j.foodchem.2018.12.035. [Google Scholar] [CrossRef]
50. Wu, J. Q., Hettenhausen, C., Meldau, S., Baldwin, I. T. (2007). Herbivory rapidly activates MAPK signaling in attacked and unattacked leaf regions but not between leaves of Nicotiana attenuate. The Plant Cell, 19(3), 1096–1122. DOI 10.1105/tpc.106.049353. [Google Scholar] [CrossRef]
51. Dong, F., Fu, X. M., Watanabe, N., Su, X. G., Yang, Z. Y. (2016). Recent advances in the emission and functions of plant vegetative volatiles. Molecules, 21(2), 124. DOI 10.3390/molecules21020124. [Google Scholar] [CrossRef]
52. Köllner, T. G., Schnee, C., Gershenzon, J., Degenhardt, J. (2004). The variability of sesquiterpenes emitted from two Zea mays cultivars is controlled by allelic variation of two terpene synthase genes encoding stereoselective multiple product enzymes. The Plant Cell, 16(5), 1115–1131. DOI 10.1105/tpc.019877. [Google Scholar] [CrossRef]
53. Aubourg, S., Lecharny, A., Bohlmann, J. (2002). Genomic analysis of the terpenoid synthase (AtTPS) gene family of Arabidopsis thaliana. Molecular Genetics and Genomics, 267(6), 730–745. DOI 10.1007/s00438-002-0709-y. [Google Scholar] [CrossRef]
54. Huisman, G. W., Liang, J., Krebber, A. (2010). Practical chiral alcohol manufacture using ketoreductases. Current Opinion in Plant Biology, 14(2), 122–129. DOI 10.1016/j.cbpa.2009.12.003. [Google Scholar] [CrossRef]
55. Noey, E. L., Tibrewal, N., Jiménez-Osés, G., Osuna, S., Park, J. et al. (2015). Origins of stereoselectivity in evolved ketoreductases. Proceedings of the National Academy of Sciences, 112(51), E7065–E7072. DOI 10.1073/pnas.1507910112. [Google Scholar] [CrossRef]
56. Suárez-Franco, G., Hernández-Quiroz, T., Navarro-Oca, A., Oliart-Ros, R. M., Valerio-Alfaro, G. (2010). Plants as a green alternative for alcohol preparation from aromatic aldehydes. Biotechnology and Bioprocess Engineering, 15(3), 441–445. DOI 10.1007/s12257-009-0207-8. [Google Scholar] [CrossRef]
57. Bennamane, M., Razi, S., Zeror, S., Aribi-Zouioueche, L. (2018). Preparation of chiral phenylethanols using various vegetables grown in Algeria. Biocatalysis and Agricultural Biotechnolog, 14, 52–56. DOI 10.1016/j.bcab.2018.02.003. [Google Scholar] [CrossRef]
58. Yadav, J. S., Nanda, S., Reddy, P. T., Rao, A. B. (2010). Efficient enantioselective reduction of ketones with Daucus carota root. Journal of Organic Chemistry, 67(11), 3900–3903. DOI 10.1021/jo010399p. [Google Scholar] [CrossRef]
59. Utsukihara, T., Watanabe, S., Tomiyama, A., Chai, W., Akira Horiuchi, C. (2006). Stereoselective reduction of ketones by various vegetables. Journal of Molecular Catalysis B: Enzymatic, 41(3), 103–109. DOI 10.1016/j.molcatb.2006.05.001. [Google Scholar] [CrossRef]
60. Yang, Z. H., Zeng, R., Yang, G., Wang, Y., Li, L. Z. et al. (2008). Asymmetric reduction of prochiral ketones to chiral alcohols catalyzed by plants tissue. Journal of Industrial Microbiology & Biotechnology, 35(9), 1047–1051. DOI 10.1007/s10295-008-0381-2. [Google Scholar] [CrossRef]
61. Orden, A. A., Bisogno, F. R., Cifuente, D. A., Giordano, O. S., Sanz, M. K. (2006). Asymmetric bioreduction of natural xenobiotic diketones by Brassica napus hairy roots. Journal of Molecular Catalysis B: Enzymatic, 42(3–4), 71–77. DOI 10.1016/j.molcatb.2006.06.010. [Google Scholar] [CrossRef]
Cite This Article
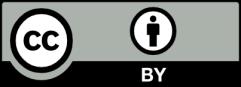
This work is licensed under a Creative Commons Attribution 4.0 International License , which permits unrestricted use, distribution, and reproduction in any medium, provided the original work is properly cited.