Open Access
ARTICLE
Drought-Mediated Modulation in Metabolomic Profiling of Leaf, Growth, Ecophysiology and Antioxidants
1 Department of Biological Sciences, Faculty of Science, King Abdulaziz University, Jeddah, Saudi Arabia
2 Princess Dr. Najla Bint Saud Al-Saud Center for Excellence Research in Biotechnology, King Abdulaziz University, Jeddah, Saudi Arabia
3 Department of Public Health, Daffodil International University, Dhaka, Bangladesh
4 Department of Biotechnology, Jamia Millia Islamia, New Delhi, India
* Corresponding Authors: Khalid Rehman Hakeem. Email: ,
(This article belongs to the Special Issue: Multi-omics Approach to Understand Plant Stress Tolerance)
Phyton-International Journal of Experimental Botany 2023, 92(12), 3323-3344. https://doi.org/10.32604/phyton.2023.030212
Received 26 March 2023; Accepted 02 November 2023; Issue published 28 December 2023
Abstract
Abiotic stresses, including drought, have been found to affect the growth and medicinal quality of numerous herbs. The proposed study aims to study the effects of different drought regimes on the metabolic profile, growth, ecophysiology, cellular antioxidants, and antioxidant potential of Nigella sativa (Black cumin) leaf. Forty-day-old seedlings of N. sativa were exposed to three regimes of drought (control, moderate and high) for a week. UPLC-MS/MS metabolic profile of the leaf reveals the presence of more than a hundred metabolites belonging to anthocyanins, chalcones, dihydro flavonoids, flavonoids, flavanols, flavones, flavonoid carbonoside, isoflavones, etc. Drought was found to alter the contents of identified metabolites. Drought stress-induced oxidative stress and increased production of hydrogen peroxide and superoxide anions. Physiological changes, activities of antioxidant enzymes, contents of antioxidants, and proline were significantly high under drought to protect against the low water regimes. Furthermore, stressed leaf extract had higher antioxidant potential. Thus, N. sativa leaf bears multiple metabolic pathways and can tolerate a higher degree of drought or osmotic stress.
Keywords
Supplementary Material
Supplementary Material FileSeeds of Nigella sativa are medicinally important and known as Hbaẗạlbrkaẗ (Seeds of blessing) or black cumin. These seeds are used in various medicinal purposes and as folk medicine in Arab and many parts of the world [1,2]. It is a prophetic medicine effective against a wide range of diseases and disorders [3]. The seeds and their extract have been shown to protect against oxidative stress, cancer, hyperlipidemia, diabetes, hypertension, asthma, ulcers, etc., [4–6]. Furthermore, it has shown inhibition of chemotherapy-induced nephrotoxicity by seed extract and its’ active ingredient thymoquinone [7]. Literature is full of high therapeutic values of black cumin. However, there are huge gaps in the knowledge about the metabolism and molecular biology of black cumin under stress factors. There are several reports on seed metabolite studies of N. sativa [8,9]. However, to the best of our knowledge, there is no report of stress impact on the metabolic profile of N. sativa leaves.
Abiotic stresses adversely affect plants from molecular to metabolic levels [10]. However, numerous studies are showing a positive impact of stresses on the herbs medicinal quality [11] but at the cost of biological yield [12]. Abiotic stresses are the major cause of reduced yield and quality of crops and medicinal plants [13]. Drought among them is the most prevalent and widespread [14]. Plants respond to drought at numerous fronts including molecular and metabolic [15]. There are no reports yet on the impact of drought, to best of our knowledge, on the medicinal quality of N. sativa leaves. Additionally, the thymoquinone pathway of N. sativa leaf has never been studied in response to the drought. However, impact of long-term stress in respect to the fatty acid profile has been studied in leaf of an important medicinal plant [16]. Changes brought by drought in the metabolic profiling may potentially affect physiochemical parameters of the medicinally important black cumin seeds. Therefore, it is considered an important research gap in the literature on the impact of abiotic stress on the metabolism, growth, and qualitative attributes of N. sativa which should be immediately addressed with the use of advanced techniques and tools.
Antioxidant capacity is the cumulative effect of ascorbate-glutathione antioxidant system, its rate of action, and the expressive potential of the cell [12,14]. As a mere fact, drought causes an osmotic imbalance in the plants hence plants should be able to synthesize and accumulate osmoprotectants including proline. Though there might be many molecules participating in specific activities or as a holistic effect, still at least one metabolic pathway is said to be of enormous consideration. Through this pathway, black cumin produces thymoquinone and dithymoquinone and nigellone [17]. There are several medicinal benefits of black cumin. It is a potent anti-inflammatory and its oil inhibits both lipoxygenase and cyclooxygenase [18] acts as a Ca2+ antagonist, relaxes tracheal and aortic muscles, destroys many vicious chemo-resistant cancer cells and potent anti-diabetic. The use of seeds also activate antioxidant enzymes including superoxide dismutase (SOD), glutathione peroxidase (GPX), and catalase, enforces bradycardia, and lowers the heart rate producing a better effect than diltiazem and lowering hypertension. It reduces cholesterol, lipid, and triglycerides in the blood, beneficial for pulmonary effect, fibrosis, granuloma, necrosis, alveolar edema, and other diseases [1–10]. Besides, it is hepatoprotective, anti-ulcer, nephroprotective, anxiolytic, diuretic, anti-urolithic, anti-spasmolytic, and provides up to 90% protection against autoimmune encephalomyelitis. Other actions such as anthelmintic, anti-schistosomiasis, antibacterial, and anti-fungal have also been reported [19]. Plant pigments mainly chlorophylls (a and b) and carotenoids are considered as health indicators of the plant being associated with photosynthesis. Their ontents are ofter compromised under stresses. Stress inhibits the rate of photosynthesis ultimately affecting the supply of photosynthates to different pathways. This loss in the biosynthesis of source molecules not only affects the concentration of active ingredients but also the biological yields [11]. Thus, any such stress which results in reduced biological and medicinal yields ultimately ends up in a widening of the supply-demand gap and high prices.
The high throughput examination of biomolecules in organisms is made possible by omics technologies. Critical information from metabolomics aids in the identification of metabolites. Any metabolites that are detected and later recognized in biological samples can be quantified. These extremely diverse metabolites may possess significant physicochemical traits. Many of these metabolites may have medicinal importance. There are now many analytical platforms available for the precise identification of phytochemicals. Integration of efficient extraction methods, chromatographic techniques, and computational and huge public metabolite libraries have made metabolomic studies much more reliable and have high throughput results [20]. Liquid chromatography with tandem mass spectrometry (LC-MS/MS) for quantitative and qualitative metabolomic and proteomic studies is gaining trust and attention for robust and quantitative performance [21,22]. Therefore, LC-MS/MS has the potential to serve efficiently for ion annotation, metabolite identification, high resolution to many levels, spectral interpretation, spectral matching, and metabolite prediction.
The present work aimed to study the impact of moderate and severe drought on metabolome, the magnitude of oxidative stress, and the response of cellular antioxidants in Nigella sativa. Other parameters included physiochemical and osmotic stress tolerance markers. Assessment of in vitro total antioxidant capacity, other radical-quenching capacities, and ferric-reducing antioxidant power of N. sativa is also studied. A possible mechanism of thymoquinone biosynthesis regulation has been proposed with the help of simulated molecular docking.
Seeds of Nigella sativa (Batch no. E17G0109) were procured from Europa Gewürze GmbH, Berlin, Germany. Five g of seeds were washed thrice with detergent followed by another 5 washes by double-distilled water (DDW). Then, seeds were placed in 5% sodium hypochlorite for 5 min followed by 10 washes by DDW. Seeds were left in DDW for 3 days for proper imbibition in the dark at 25°C. The seeds were again washed with DDW and placed on 4-layers sterilized blot sheets making a wet bed for germination, in petri-dish (150 mm × 15 mm). There was germination in almost 100% seeds. Five-day-old sprouts were transferred to the pots (100 mm × 75 mm, 20 each) containing 200 g sandy-loam greenhouse soil and Soilrite® and thoroughly mixed in 1:1 proportion. The pots, equipped with a wicking system, were supplied with half-strength Hoagland nutrient media on alternate days.
Pots were placed in a walk-in growth chamber with 70%–80% relative humidity, 30°C/25°C day/night temperature, 250 µmol photons m−2 s−1, 10/14 h light/dark cycles and one month later, plants were exposed to polyethylene glycol (PEG6000) viz. Control = 0.0%, moderate (7.5% PEG6000) and high (15% PEG6000) drought/osmotic stress. Water potential (Ψw) at 25°C/30°C for control, 7.5% PEG6000 and 15% PEG6000 was 0.0/0.0, −0.152/−0.137, and −0.304/−0.274 MPa, respectively (Supplementary Table 1). Stress solutions were prepared by dissolving PEG6000 in DDW and supplied as per the water holding capacity of the soil and through a wicking plate, in a total of 200 mL. For the rest of the growth period, half-strength Hoagland nutrient media was supplied. Five days after stress exposure, all parameters were studied in the fresh leaves. For dry weight estimations, leaves were harvested (40-days-old), weighed, and dried in a hot-air oven at 65°C for 3 days, till constant weight. For protein estimation, the Bradford reagent was used and concentration in the samples was calculated against the bovine serum albumin (BSA) standard curve [23]. All biochemical estimations were made on a dry weight (DW) basis, except metabolomics.
A fresh leaf sample (150 mg) of N. sativa was pulverized in liquid-N2 and extracted twice, 1 h each, in 15 mL HPLC grade EtOH (80%) in a closed vial at room temperature. The extract was filtered through a 0.2 µm membrane syringe filter into a fresh vial. The solvent was evaporated in a Laminar flow chamber. Metabolites were dissolved in 500 µL EtOH as the final volume and used for HPLC-MS/MS analysis.
2.1.2 Metabolome Profiling Employing nanoLC-MS/MS
200 µL of the sample was added to 200 µL 4% phosphoric acid and vortex-mixed. Following centrifugation, a 200 µL sample was loaded onto an OasisTM PRiME HLB µElution plate, and processed further for nanoLC-MS/MS.
2.1.3 Data Processing and Metabolite Identification
The spectrum of both negative and positive ions was fed into the ResPect database and metabolites with the best score were selected. The details of metabolite such as structure, mass, and molecular formula were retrieved from the PubChem database.
2.1.4 Thiobarbituric Acid Reactive Substances (TBARS)
The concentration of TBARS in fresh leaves of N. sativa was estimated by the method [24]. 500 mg of the fresh leaf was homogenized in 5 mL of 0.1 % (w/v) TCA (trichloroacetic acid) and centrifuged at 7826 g for 5 min. To 500 µL aliquot, 2 mL of 0.5 % (w/v) thiobarbituric acid (TBA) was added, and samples were heated at 99°C for 45 min. Samples were cooled and centrifuged at 1957 g for 5 min. The supernatant was read at 532, and 600 nm to correct the values by deducing non-specific turbidity. TBA-reactive substances (TBARS) were calculated using 155 mM−1 cm−1 as the absorbance coefficient. Values were expressed as nmol g−1 DW.
2.1.5 Physiological Stress Markers
The magnitude of osmotic and secondary stresses in the leaves of Nigella sativa leaves was assessed to study the impact of PEG-mediated drought stress. The following parameters were estimated in control and drought (low and high) exposed plants.
The concentration of free proline in leaves of N. sativa was estimated by the method [25]. One gram of fresh sample was homogenized in 20 mL of 3% (w/v) sulphosalicylic acid. Samples were centrifuged at 7826 g at 4°C for 10 min. Four mL of acid ninhydrin reagent, 4 mL of aliquot, and 2 mL of glacial acetic acid were added. The mixture was boiled at 100°C for 30 min in a water bath followed by immediate cooling. In the samples, 8 mL of toluene was added which led to the formation of two distinct layers; the upper layer was read at 520 nm and the concentration of proline was determined against the standard curve of L-proline and expressed in mg g−1 DW.
The concentration of cysteine was estimated in leaves of N. sativa L. 100 mg leaf material was boiled in 0.5 ml of 6 N HCl for 10 min and the volume was made to 2.0 ml with 0.5 N HCl. A 0.3 ml of chilled 5% v/v perchloric acid was added to the mixture which was incubated at 4°C for an hour. The samples were then processed [26]. The optical density of the developed pink colour was read at 560 nm and the amount of cysteine in gel pieces was calculated against the cysteine standard curve obtained under similar conditions and expressed in µmole.
2.1.8 Visual Detection of Superoxide Radicals
Visual detection of superoxide (O2●−) was evaluated by staining leaves and stems with nitro blue tetrazolium (NBT) salt as a method [27]. In this method, the leaves/stems were drifted in 10 mL, 0.2% NBT solution (NBT solution prepared in 50 mM Na3PO4, pH 7.4) for 2 h at room temperature. Next, stained leaves were boiled in absolute ethanol for the elimination of chlorophyll and then cleaned with DDW. Photographs of blue spots containing leaves were taken.
2.2 Rate of Photosynthesis and Stomatal Conductance
Net photosynthetic rate and stomatal conductance were measured in vivo on very thin and minute leaves using a GFS-3000 portable photosynthetic system (Walz, Germany) with light saturating intensity as described [28]. Saturated pulses (600 ms) of white light (4500 E m−2 s−1) were used to determine the rate of photosynthesis (PN) and stomatal conductance (GH2O, Gs). All measurements were carried out between 09:00 and 10:00 A.M. During the measurements, the air relative humidity was about 80%, the leaf temperature ranged from 22°C to 24°C and the ambient CO2 concentration at about 355 mmol mol−1.
2.3 Evaluation of Cellular Antioxidant System
To study the level of defense offered by N. sativa in terms of the activity of cellular antioxidants under drought stress was analyzed.
Superoxide dismutase activity was measured by the method [29]. 200 mg fresh leaf of N. sativa was homogenized in 2 mL of extraction buffer (0.5 M Na-phosphate buffer, pH 7.3, 3 mM EDTA) followed by centrifugation at 11269 g for 5 min at 4°C. The assay of 100 µL enzyme extract was performed in a reaction buffer (0.1 M Na-phosphate buffer, pH 7.5) containing recommended amounts of 20 mM methionine, 1 M NaHCO3, 3 mM EDTA, 2.25 mM NBT, and 60 µM riboflavin. The reaction was performed under a 15 W inflorescent lamp at 28°C. 50% protection of NTB by SOD from photo-reduction was considered as one unit of enzyme activity min−1 mg−1 protein.
The activity of ascorbate peroxidase in the leaf of N. sativa was measured by the method [30]. One g fresh leaf of N. sativa was homogenized in 5 mL of extraction buffer (0.2 M potassium-phosphate, pH 7.1, 3 mM EDTA) and centrifuged at 7826 g for 10 min at 4°C. APX activity was measured at 290 nm for 5 min in 100 µL of enzyme extract in a buffer containing recommended amounts of 0.5 mM ascorbate, 0.1 mM H2O2 and 0.5 mM EDTA. Units were expressed as the amount of APX that degraded 1 μmol of ascorbate min−1 mg−1 protein.
2.3.3 Glutathione Reductase (GR)
The activity of glutathione reductase was measured by the method [31]. One g fresh leaf of N. sativa was homogenized in 5 mL of extraction buffer (0.2 M Na-phosphate, pH 7.1, 3 mM EDTA) and centrifuged at 7826 g at 4°C for 15 min. GR activity was assayed in the supernatant catalyzed by NADPH and read at 340 nm. In brief, 100 µL supernatant was added to the reaction mixture containing recommended volumes of 0.2 mM NADPH and 0.5 mM GSSG at 25°C for 5 min. units were expressed as the amount of enzyme necessary to decompose 1 μmol of NADPH min−1 mg−1 protein.
The activity of catalase was measured by the method [32]. One g fresh leaf of N. sativa was ground in 5 mL of extraction buffer (0.5 M Na-phosphate, pH 7.3) followed by centrifugation at 7826 g/4°C for 15 min. CAT activity was measured in 100 µL of an aliquot by reading the decrease in H2O2 concentration at 240 nm in a reaction buffer (0.4 M Na-phosphate, pH 7.2) for 5 min. Units are expressed as the amount of enzyme required to decompose 1 μmol of H2O2 min−1 mg−1 protein.
2.3.5 Glutathione S-Transferase (GST)
The activity of glutathione S-transferase was measured by the method [33]. One g fresh leaf of N. sativa was homogenized in 5 mL of extraction buffer (0.5 M Na-phosphate, pH 7.4) followed by centrifugation at 7826 g/4°C for 15 min. GST activity was measured at 340 nm in 100 µL of an aliquot in a reaction buffer (150 mM potassium phosphate buffer, pH 6.5), 2 mM EDTA, 1 mM reduced glutathione, 1 mM 1-chloro 2, 4-dinitrobenzene (CDNB). Units were expressed as the amount of enzyme for 1 µmol oxidized CDNB min−1 mg−1 protein.
The activity of ATP-Sulfurylase (ATPS) was measured in vitro by the method [34]. One g fresh leaf of N. sativa was ground in 10 mL of extraction buffer (120 mM Tris–HCl, pH 8.0) containing recommended amounts of MgCl2, KCl, DTE, and MgCl2·6H2O. The homogenate was centrifuged at 7984 g at 4°C for 15 min.
ATPS activity was assayed in 40 µL of an aliquot in 200 µL of the reaction mixture (150 µL of 0.5 M Tris buffer, pH 8.0, 200 µL DDW). The recommended amount of MgCl2·6H2O (40 mM), Na2ATP, Na2MoO4, and inorganic pyrophosphatase was added accordingly. Incubated at 33°C for 30 min, an incubation mixture was added with an appropriate amount of 2.5% ammonium molybdate solution and reducing agent (3 g of Na2SO3·7H2O, 1 g of 1-amino-naphthol sulfonic acid, 6 g of Na2S2O5). The solution was read 660 nm after 20 min and measurements were made against a standard curve of KH2PO4. Units were expressed as the amount of enzyme required for the conversion of 1 µmol of APS and PPi to ATP min−1 mg−1 protein.
2.4 Assessment of Antioxidant Potentials of Seed and Leaf of Nigella sativa
2.4.1 DPPH (1, 1-Diphenyl-2-Picrylhydrazyl) Radical Scavenging
Free radical scavenging activity of N. sativa seed (aqueous extract, 100 mg/mL) and leaves (aqueous extract, 100 mg/mL) was measured in terms of radical scavenging ability using the stable free radical DPPH. Extracts were centrifuged at 1250 g for 5 min and 100 μL of each sample was added with 50 μL of 0.659 mM DPPH (prepared in MeOH) solution. Samples were incubated at 25°C/20 min. The absorbance was recorded at 510 nm.
DPPH Scavenging ability (%) = [Acontrol − Asample/Acontrol] × 100
2.4.2 Total Antioxidant Capacity (TAC)
The total antioxidant capacity of seed and leaf extracts was determined as mentioned by [35]. To 100 μL of extract, 1 mL of reagent [28 mM sodium phosphate, 0.6 M sulphuric acid, and 4 mM ammonium molybdate] was added. The samples were incubated at 95°C for 90 min, cooled in an ice bath, and read at 695 nm.
Experiments were conducted on a randomized block design (RBD) with three biological replicates. Three experimental replicates (n = 3) were considered for each treatment. One-way ANOVA followed by the Brown-Forsythe test was performed using GraphPad Prism version 8.4.3 for Windows, GraphPad Software, La Jolla, California, USA. p-value for comparison among treatments viz. Control vs. MD, Control vs. HD, and MD vs. HD. A significant difference among means is shown as p < 0.05. Actual p-values are mentioned in the legend of every figure.
3.1 Metabolic Profiling of Leaf
In the tertiary leaves of N. sativa, a total of 75 metabolites were detected and identified using UPLC-MS/MS and ReSpect metabolites portal (http://spectra.psc.riken.jp/). Other details associated with the molecular formula, mass, and structure were retrieved from PubChem (https://pubchem.ncbi.nlm.nih.gov/) database. All the detected metabolites have been listed in Table 1.
3.2 Identification of Many Coenzymes A
We identified six types of coenzymes, namely 3’-dephosphocoenzyme A, acetyl coenzyme A, beta-methylcrotonyl coenzyme A, glutaryl coenzyme A, isobutyryl coenzyme A, and n-propionyl coenzyme A.
3.3 Identification of Kaempferol and Quercetin Pathways and Their Interaction with Glycosides
In the present study, both classes of primary and secondary metabolites were identified. Among secondary metabolites, metabolic pathways associated with kaempferol, quercetin, delphinidin, vitexin, cyanidin, and numerous flavonoids were identified. Many metabolites of these groups were forming their rhamnosides and/or glucosides.
Metabolites of kaempferol metabolic pathways included kaempferol-3,7-O-di-rhamnopyranoside, kaempferol-3-glucoside, kaempferol-3-glucoside-2″-p-coumaroyl, kaempferol-3-glucuronoid, kaempferol-3-O-alpha-L-arabinoside, kaempferol-3-O-alpha-L-rhamnopyranosyl(1-2)-beta-D-glucopyranoside-7-O-alpha-L-rhamnopyranoside, kaempferol-3-O-beta-D-glucoside-7-O-alpha-L-rhamnoside, and kaempferol-3-rhamnoside-4″-rhamnoside-7-rhamnoside.
Metabolites of quercetin pathway identified were quercetin-3-O-beta-glucopyranoside, quercetin-3-D-xyloside, quercetin-3,4′-O-di-beta-glucopyranoside, quercetin-3,7-O-alpha-L-dirhamnopyranoside and quercetin-3-galactoside-6″-rhamnoside-3″′-rhamnoside.
Interestingly, several rhamnose-containing metabolites were identified. In addition of comlexing with kaempferol and quercetin, isorhamnetin-3-galactoside-6″-rhamnoside, L-rhamnose monohydrate, Petunidin-3-O-(6″-O-(4″′-O-E-coum)-alpha-rhamnopyranosyl-beta-glucopyranosyl)-5-O-beta-glucopyranoside trifluoroacetate salt and vitexin-2″-O-rhamnoside were identified.
An important apigenin (apigenin—8-C-glucoside, a flavanoid) vitexin was identified along with vitexin-2″-O-rhamnoside.
3.5 Identification of Cinnamic Acid Derivatives
An important metabolic pathway that includes cinnamic acid was identified in the leaves of N. sativa. Cinnamic acid is the first molecule in the phenylpropanoid pathway where hydroxycinnamic acid derivatives are formed. Such metabolites include caumeric compounds and caffeine which were also identified in the present study.
3.6 Identification of Numerous Important Metabolites
Besides the above-mentioned metabolites, numerous derivatives of phenolic, flavonoids, alkaloids, etc., were identified including, fortunellin, daidzein, esculin, linarin, harmaline, petunidin, neodiosmin, puerarin, cyanidin, resveratrol, rosmarinic acid, sebacic acid, and many more (Table 1).
3.7 Drought Affected the Concentrations of Metabolites in the Leaf of Nigella sativa
The severe drought in this study reflected that metabolic compounds of N. sativa were severely modulated. Changes in the concentrations of metabolites are indicative of the impact of drought on the metabolic pathways operating in the leaves. A comparative account of percent (%) changes in drought-exposed plants over control has been shown in Table 1.
Several compounds present in the stressed plants were missing in the control plants. Similarly in drought-exposed ones, many compounds were not detected. However, metabolites that were most impacted under drought were chlorophyll a and b, cyaniding derivative, esculin derivative, fortunellin, kaempferol-3-O-alpha-L-arabinoside, phenylalanine, neodiosmin, syringetin-3-O-glucoside and vitexin. Among other common metabolites, mostly an increase in the contents was noted.
3.8 Impact of Drought on Plant Growth
During the time of the study, there was the formation of three types of leaves viz. cotyledonary, primary and secondary leaves. Initially, the cotyledonary leaf was trifurcated followed by a further trifurcation of each leaf lobe. Therefore, by the time the study was completed nine lobes, 3 in groups of 3 each were formed (Fig. 1). Drought regimes clearly showed an adverse impact on the growth of leaves. Drought-stressed plants had smaller leaves with reduced lamina (Fig. 1).
Figure 1: Impact of moderate and high levels of drought on different leaves of Nigella sativa L. Control (Ψw = 0.0 MPa), MD (Ψw = −152 to −0.137 MPa) and HD (Ψw = −0.304 to −0.274 MPa)
3.9 Physiological Stress Markers
3.9.1 Magnitude of Oxidative Stress
Concentration of TBARS in the leaf of control (Ψw = 0.0 MPa) plants was 8.5 nmol g−1 DW whereas in MD (Ψw = −152 to −0.137 MPa) and HD (Ψw = −0.304 to −0.274 MPa) content of TBARS increased to 13.0 nmol g−1 DW and 15.3 nmol g−1 DW, respectively (Fig. 2).
Figure 2: Impact of moderate and high levels of drought on the magnitude of TBARS concentration, in leaves of Nigella sativa L. Control (Ψw = 0.0 MPa), MD (Ψw = −152 to −0.137 MPa) and HD (Ψw = −0.304 to −0.274 MPa). p-value = 0.2505, *p < 0.0001. n = 3. Error bars represent standard deviation (SD)
3.9.2 Free Proline Accumulation
The concentration of free proline in the leaf of Control (Ψw = 0.0 MPa) plants was 21.84 µmol g−1 DW. In MD (Ψw = −152 to −0.137 MPa) and HD (Ψw = −0.304 to −0.274 MPa) plants the concentration of free proline increased to 72.55 µmol g−1 DW and 82.17 µmol g−1 DW, respectively (Fig. 3A).
Figure 3: (A–B): Impact of MD (Ψw = −152 to −0.137 MPa) and HD (Ψw = −0.304 to −0.274 MPa) on the accumulation of free proline (A) and cysteine (B) in leaves of Nigella sativa L. For free proline, p-value = 0.5247, p < 0.0001. For free cysteine, p-value = 0.9521, *p < 0.0001. n = 3. Error bars represent standard deviation (SD)
The concentration of cysteine in the leaf of Control (Ψw = 0.0 MPa) plants was 414 nmol g−1 DW. In MD (Ψw = −152 to −0.137 MPa) and HD (Ψw = −0.304 to −0.274 MPa) plants the concentration of cysteine increased to 486 and 618 nmol g−1 DW, respectively (Fig. 3B).
3.9.4 Histochemical Localization of Superoxide Radicals
In the leaf and stem of drought-teared plants, there was a dose-dependent change in the localization of superoxide radicals. This directly shows the amount of superoxide radicals was higher in the highest drought regime (Fig. 4).
Figure 4: (A–C): Depiction of impact of different drought regimes in terms of accumulation of superoxide radicals in the leaves and stem of Nigella sativa L. A. Control (Ψw = 0.0 MPa), B. MD (Ψw = −152 to −0.137 MPa) and 3. HD (Ψw = −0.304 to −0.274 MPa)
3.10 Photosynthesis, Stomatal Conductance and Chlorophylls
3.10.1 The Net Rate of Photosynthesis (P N)
The net rate of photosynthesis in the leaf of Control (Ψw = 0.0 MPa) plants was 8.94 µmol CO2 m2 s−1. MD (Ψw = −152 to −0.137 MPa) and HD (Ψw = −0.304 to −0.274 MPa) declined the PN to 7.20 µmol CO2 m2 s−1 and 5.40 µmol CO2 m2 s−1, respectively (Fig. 5A).
Figure 5: (A–B): Impact of MD (Ψw = −152 to −0.137 MPa) and HD (Ψw = −0.304 to −0.274 MPa) drought on the rate of photosynthesis (A) and stomatal activity (B) in leaves of Nigella sativa L. compared to Control (Ψw = 0.0 MPa) plants. For PN, p-value = 0.6133, *p < 0.0001. For Gs, p-value = 0.3410, p < 0.0155. n = 3. Error bars represent standard deviation (SD)
3.10.2 Stomatal Conductance (Gs)
Stomatal conductance in the leaf of Control (Ψw = 0.0 MPa) plants was 0.469 mmol H2O m2 s−1. MD (Ψw = −152 to −0.137 MPa) and HD (Ψw = −0.304 to −0.274 MPa) declined the Gs to 0.367 mmol H2O m2 s−1 and 0.314 mmol H2O m2 s−1, respectively (Fig. 5B).
3.11 Evaluation of Antioxidant Enzymes
The activity of SOD in the leaf of Control (Ψw = 0.0 MPa) plants was 7.55 enzyme unit (EU). MD (Ψw = −152 to −0.137 MPa) and HD (Ψw = −0.304 to −0.274 MPa) droughts increased the activities of SOD to 15.14 EU and 19.57 EU, respectively (Fig. 6A).
Figure 6: (A–D): Impact of MD (Ψw = −152 to −0.137 MPa) and HD (Ψw = −0.304 to −0.274 MPa) droughts on activities of (A) superoxide dismutase (SOD), (B) ascorbate peroxidase (APX), (C) glutathione reductase (GR) and (D) catalase (CAT) in Nigella sativa L. leaves compared to Control (Ψw = 0.0 MPa). For SOD, p-value = 0.5732, p < 0.0001. For APX, p-value = 0.5330, p < 0.0001. For GR, p-value = 0.8540, *p < 0.0001. For CAT, p-value = 0.9485, p < 0.0012. n = 3. Error bars represent standard deviation (SD)
The activity of APX in the leaf of Control (Ψw = 0.0 MPa) plants was 23.57 EU. MD (Ψw = −152 to −0.137 MPa) and HD (Ψw = −0.304 to −0.274 MPa) droughts increased the activities of APX to 26.22 and 30.66 EU, respectively (Fig. 6B).
The activity of GR in the leaf of Control (Ψw = 0.0 MPa) plants was 11.65 EU. MD (Ψw = −152 to −0.137 MPa) and HD (Ψw = −0.304 to −0.274 MPa) droughts increased the activities of GR to 16.53 and 18.89 EU, respectively (Fig. 6C).
The activity of CAT in the leaf of Control (Ψw = 0.0 MPa) plants was 17.16 EU. MD (Ψw = −152 to −0.137 MPa) and HD (Ψw = −0.304 to −0.274 MPa) droughts increased the activities of CAT to 20.80 and 22.66 EU, respectively (Fig. 6D).
3.11.5 Glutathione S-Transferase (GST)
The activity of glutathione S-transferase (GST) in the leaf of Control (Ψw = 0.0 MPa) plants was 41.7 EU. MD (Ψw = −152 to −0.137 MPa) increased the activities of GST to 44.76 EU; however, HD (Ψw = −0.304 to −0.274 MPa) decreased GST activity to 31.11 EU (Fig. 7A).
Figure 7: (A–B): Impact of MD (Ψw = −152 to −0.137 MPa) and HD (Ψw = −0.304 to −0.274 MPa) droughts on activities of (A) glutathione S-transferase (GST) and ATP sulfurylase (ATPS) in Nigella sativa L. leaves compared to Control (Ψw = 0.0 MPa). For GST, p-value = 0.0015, p < 0.05. For ATPS, *p-value = 0.0001. n = 3. Error bars represent standard deviation (SD)
The activity of ATP-sulfurylase (ATPS) in the leaf of Control (Ψw = 0.0 MPa) plants was 2.13 EU. MD (Ψw = −152 to −0.137 MPa) and HD (Ψw = −0.304 to −0.274 MPa) increased the activities of ATPS to 3.86 and 6.74 EU, respectively (Fig. 7B).
3.12 Assessment of Antioxidant Potentials of Leaf of Nigella sativa
3.12.1 DPPH (1, 1-Diphenyl-2-Picrylhydrazyl) Radical Scavenging
Leaf extracts of Control (Ψw = 0.0 MPa), MD (Ψw = −152 to −0.137 MPa) and HD (Ψw = −0.304 to −0.274 MPa) plants showed 10.55%, 12.31% and 18.33% DPPH radical scavenging potential, respectively (Fig. 8A).
Figure 8: (A–B): Impact of MD (Ψw = −152 to −0.137 MPa) and HD (Ψw = −0.304 to −0.274 MPa) droughts on the DPPH radical scavenging (A) and total antioxidant potential (B) in the leaves of Nigella sativa L compared to Control (Ψw = 0.0 MPa). For DPPH, p-value = 0.2505, *p < 0.0001. For TAC, *p-value =< 0.0001, n = 3. Error bars represent standard deviation (SD)
3.12.2 Total Antioxidant Capacity (TAC)
Leaf extracts of Control (Ψw = 0.0 MPa), MD (Ψw = −152 to −0.137 MPa) and HD (Ψw = −0.304 to −0.274 MPa) showed 40.44%, 47.69% and 23.81% total antioxidant capacity, respectively (Fig. 8B).
4.1 Metabolic Profiling of Leaf
The presence of metabolic pathways in the leaves has great variation from plant to plant. Usually, it is a common understanding that colored organs of plants are rich in several flavonoids, anthocyanins, chalcone, and other pigments. To a surprise, perfectly green-looking leaves of N. sativa were found to possess a great variety of metabolites of several pathways. There are numerous studies on the metabolic profiling of seeds and seed oil of N. sativa [36,37]. As far as we know, this is the first study to analyze metabolites in the fresh leaves of Nigella sativa L. by UPLC-MS/MS. A total of 75 metabolites were detected. Besides many primary metabolites, these substances belong to flavonoids, flavonoid carbonoside, dihydroflavonoid, flavonols, dihydroflavonol, isoflavones, flavone, anthocyanin, and chalcone, etc.
Kaempferol is a flavonol (flavonoid) occurring naturally in plants [38] and has been assigned different therapeutic properties against many types of cancers, inflammation, liver injury, obesity, diabetes, etc., [39]. The presence of at least many derivates of kaempferol indicates that the leaf of this plant is of a wide spectrum therapeutic. 4-Hydroxy-3-methoxycinnamaldehyde treats atopic dermatitis improves immune response and is a potent anti-inflammatory [40]. Hydroxycinnamic acids (such as ferulic, caffeic, sinapic, and p-coumaric acids) are a group of compounds highly abundant in food that may account for about one-third of the phenolic compounds in our diet. Hydroxycinnamic acids have gained an increasing interest in health because they are known to be potent antioxidants [41]. 2-Hydroxycinnamic acid can detoxify organophosphates and reduce oxidative stress [42,43]. Drought has increased most of such metabolites indicating an important role of kaempferol metabolism under abiotic stress.
The flavonol 7-O-rhamnosyltransferase catalyzes the formation of kaempferol-3,7-bis-O-α-L-rhamnoside from kaempferol-3-O-α-L-rhamnopyranoside, kaempferol-3-O-β-D-glucopyranoside-7-O-α-L-rhamnopyranoside from kaempferol 3-O-β-D-glucoside, kaempferol-3-O-β-gentiobioside-7-O-α-L-rhamnoside from kaempferol 3-O-β-gentiobioside and kaempferol 3-O-β-D-glucosyl-(1->2)-β-D-glucoside-7-O-α-L-rhamnoside from kaempferol 3-O-β-D-glucosyl-(1->2)-β-D-glucoside.
When quercetin mono- and diglucosides were used as substrates the flavonol 7-O-rhamnosyltransferase converted with lower efficiency quercetin 3-O-rhamnoside to quercetin-3,7-O-dirhamnoside and with high efficiency quercetin-3-glucoside to quercetin-3-O-glucoside-7-O-rhamnoside, quercetin 3-O-β-D-glucosyl-(1->2)-β-D-glucoside to quercetin 3-O-glucosyl-(1->2)-glucoside-7-O-rhamnoside and quercetin-3-gentiobioside to quercetin 3-O-gentiobioside-7-O-rhamnoside [44]. It is phenylalanine which forms kaempferol, quercetin, myricetin, and syringetin among others [45]. Various therapeutic properties of kaempferol and its compounds and their biosynthesis and mechanism of action are detailed by various authors. Interestingly, under drought, quercetin pathway seems to be shunted off.
Vitexin and its compounds have been shown to attenuate myocardial ischemia, anticonvulsant and anxiolytic, neuroprotective and many other therapeutic properties [46–49]. Derivatives and complexes of Quercetin exhibit strong antioxidant activities [50]. Such metabolites have also been reported as hepatoprotective, antiproliferative, antiallergic, and anti-inflammation [51,52]. Quercetin-3-O-α-l-rhamnopyranoside could be an anti-influenza metabolite [53]. Under drought, the content of vitexin decreased but its rhamnoside derivative increased.
Cyanidin is a natural organic compound. It is a particular type of anthocyanidin. Its derivatives and compounds have shown antioxidant and anti-inflammatory activities [54]. Similarly, delphinidin is also an anthocyanin with antioxidant and many other therapeutic properties. Cyanidin compounds/derivatives were adversely affected by the drought stress (Table 1).
The l-Rhamnose, a deoxy monosaccharide, has been shown to play important roles in the biological functions of plants. Found primarily in cell walls and conjugated to specialized metabolites, rhamnose serves as a building block for pectic polymers and glycosylation of cell wall glycoproteins and other metabolites. In general, UDP-glucose by the action of some enzymes is converted to UDP-rhamnose. Derivatives of both UDP-glucose and UDP-rhamnose and many rhamnose-containing compounds (Table 1) have been identified in the present study.
Fortunellin is a citrus flavonoid that is a potential anti-inflammation agent in inflammatory diseases [55]. Its content was adversely affected by drought stress, which is a common founding with other such metabolites in the present study. Linarin (acacetin-7-O-β-d-rutinoside) is a naturally occurring flavonoid and it has been isolated from several medicinal plants. Various pharmacological studies have demonstrated linarin’s diverse pharmacological activities, for example, its analgesic, antipyretic, anti-inflammatory, and hepatoprotective activities, acetylcholinesterase and aldose reductase inhibitory activities, and sedative, neuroprotective and anti-apoptotic effects [56,57]. Diosmin is a nutrient that is widely contained in citrus and has been indicated to improve glucose metabolism in diabetic disorders [58]. It was interesting to note that linarin was induced by the drought which indicates its protective role against stress.
Vitamin E is a generic term for tocopherols and tocotrienols. Tocotrienols differ from tocopherols by possessing a farnesyl (isoprenoid) rather than a saturated phytyl side chain. The unsaturated side chain of tocotrienol allows for more efficient penetration into tissues that have saturated fatty layers such as the brain and liver, and other diseases [59]. An increase in this metabolite by drought proves its protective role against stress.
In general, it is evident from the results that N. sativa adjusts its metabolic pathways to drought stress. Many of the leaf metabolites were either declined or shunted off, and vice versa. Modulation of metabolic pathways is thus quite evident.
4.2 Types of Leaves, and Leaf Growth and Morphology
4.2.1 Oxidative Stress and Osmolytes
Drought has been well-known to induce an excessive amount of oxyradicals termed oxidative stress in the plant system. Generation of oxyradicals takes place through altered physiochemical metabolism and stomatal activity when the availability of CO2 for reduction, in the process of photosynthesis, falls and photorespiration operated at an elevated level [60]. Under drought stress, a fall in water potential forces the cells to accumulate compatible osmolytes including free proline and free cysteine.
Proline (Pro) plays an important role in the stress survival of plants during osmotic stress. The function assigned to free proline is as a component of protein synthesis, osmolyte, and cell signalling [61]. The sulfur amino acid cysteine (Cys) functions in many basic and essential processes of life and contributes to the structural, catalytic, regulatory, and metabolic functions [62]. Cys is also required for GSH and phytochelatin biosynthesis, stress tolerance and signalling [63].
Plant carbon metabolism including photosynthesis is extremely sensitive to drought and climate change [64]. Such an impact of drought has been attributed to several physiological and molecular alterations taking place due to adverse impacts, such as oxidative damage to chloroplasts [65]. Similarly, modulation of Gs under drought is directly associated with the rate of photosynthesis [66], as also evident from the present study.
4.2.2 Upregulation of Cellular Antioxidant Activity under Drought
Several studies show that plants increase the activity of antioxidant enzymes under drought to counter oxidative stress [66]. In this study, a rise in the activity of antioxidant enzymes approved that N. sativa upregulates antioxidant enzyme activities viz. SOD, APX, and GR. A similar response of cellular antioxidants in drought-stressed N. sativa has been reported [67]. The activity of GST was sensitive at the lower water potential which might be due to the reason that drought is a different kind of stress, moisture stress, and not inducing response as induced by toxins and metals. ATP sulfurylase (ATPS) catalyzes the first step of sulfur assimilation in photosynthetic organisms [68]. Since plants have to compensate for the impact of drought stress on proteins, thylakoids, and Fe-S clusters, N. sativa might have upregulated the activity of ATPS [69].
When it comes to the Nigella sativa research, often seed oil and metabolites remain in the focus. The research on N. sativa and diseases has not yet proven the worth of the mention of this plant in the historical documents and ancient systems of medicine. The present study considered an out-of-the-box strategy and targeted leaves of N. sativa for metabolic profiling. Our results show that N. sativa leaves bear important metabolic pathways including Cinnamic acid pathway, Chlorophylls, Kaempferol and Vitexin pathways, etc. This research has opened the vistas of future research to consider N. sativa in an array of medical and clinical studies to assess the potential of leaf metabolites in disease control. Furthermore, N. sativa seems to efficiently resist the moderate level of drought stress. However, a high level of drought causes great harm to the metabolic processes through perhaps the generation of a high concentration of oxyradicals.
Acknowledgement: The Project was funded by the Deanship of Scientific Research (DSR) at King Abdulaziz University, Jeddah, under Grant No. G:243-130-1439. The authors, therefore, acknowledge with thanks to DSR for technical and financial support.
Funding Statement: The Project was funded by the Deanship of Scientific Research (DSR) at King Abdulaziz University, Jeddah, under Grant No. G:243-130-1439.
Author Contributions: The authors confirm contribution to the paper as follows: Khalid Rehman Hakeem and M. Irfan Qureshi have generated the research idea, conducted the research, analysed the data and written the manuscript. Hesham F. Alharby has helped in the analysis of the data and edited the manuscript.
Availability of Data and Materials: This data shall be available upon request.
Ethics Approval: Not applicable.
Conflicts of Interest: The authors declare that they have no conflicts of interest to report regarding the present study.
Supplementary Materials: The supplementary material is available online at https://doi.org/10.32604/phyton.2023.030212.
References
1. Yimer, E. M., Tuem, K. B., Karim, A., Ur-Rehman, N., Anwar, F. (2019). Nigella sativa L.(black cuminA promising natural remedy for wide range of illnesses. Evidence-Based Complementary and Alternative Medicine, 2019(6), 1–16. https://doi.org/10.1155/2019/1528635 [Google Scholar] [PubMed] [CrossRef]
2. Islam, M. T., Guha, B., Hosen, S., Riaz, T. A., Shahadat, S. et al. (2017). Nigellalogy: A review on Nigella sativa. MOJ Bioequivalence & Bioavailability, 3(6). https://doi.org/10.15406/mojbb.2017.03.00056 [Google Scholar] [CrossRef]
3. Ijaz, H., Tulain, U. R., Qureshi, J., Danish, Z., Musayab, S. et al. (2017). Nigella sativa (prophetic medicineA review. Pakistan Journal of Pharmaceutical Sciences, 30(1), 229–234. [Google Scholar] [PubMed]
4. Atasever, M., Bakacak, Z. (2017). Nigella Sativa oil protects the rat ovary from oxidative injury due to ischemia-reperfusion. Medical Science Monitor, 23, 5027–5033. https://doi.org/10.12659/MSM.905356 [Google Scholar] [PubMed] [CrossRef]
5. Randhawa, M. A., Al-Ghamdi, M. S. (2002). A review of the pharmaco-therapeutic effects of Nigella sativa. Pakistan Journal of Medical Research, 41(2), 77–83. [Google Scholar]
6. Dajani, E. Z., Shahwan, T. G., Dajani, N. E. (2018). Overview of the human investigations of Nigella sativa (black seedsA complementary drug with historical and clinical significance. General Internal Medicine and Clinical Innovations, 4, 2397–5237. [Google Scholar]
7. Cascella, M., Palma, G., Barbieri, A., Bimonte, S., Amruthraj, N. J. et al. (2017). Role of Nigella sativa and its constituent thymoquinone on chemotherapy-induced nephrotoxicity: Evidences from experimental animal studies. Nutrients, 9(6), 625. [Google Scholar] [PubMed]
8. Tiruppur Venkatachallam, S. K., Pattekhan, H., Divakar, S., Kadimi, U. S. (2010). Chemical composition of Nigella sativa L. seed extracts obtained by supercritical carbon dioxide. Journal of Food Science and Technology, 47(6), 598–605. https://doi.org/10.1007/s13197-010-0109-y [Google Scholar] [PubMed] [CrossRef]
9. Farag, M. A., Gad, H. A., Heiss, A. G., Wessjohann, L. A. (2014). Metabolomics driven analysis of six Nigella species seeds via UPLC-qTOF-MS and GC-MS coupled to chemometrics. Food Chemistry, 151(2), 333–342. [Google Scholar] [PubMed]
10. Zhang, Q., Li, Y., Sun, L., Chu, S., Xu, H. et al. (2022). Integration of transcriptomic and proteomic analyses of Rhododendron chrysanthum Pall. in response to cold stress in the Changbai Mountains. Molecular Biology Reports, 50, 3607–3616. https://doi.org/10.1038/s41576-021-00413-0 [Google Scholar] [CrossRef]
11. Qureshi, M. I., Israr, M., Abdin, M. Z., Iqbal, M. (2005). Responses of Artemisia annua L. to lead and salt-induced oxidative stress. Environmental and Experimental Botany, 53(2), 185–193. [Google Scholar]
12. Qureshi, M. I., Abdin, M. Z., Qadir, S., Iqbal, M. (2007). Lead-induced oxidative stress and metabolic alterations in Cassia angustifolia Vahl. Biologia Plantarum, 51(1), 121–128. [Google Scholar]
13. Imadi, S. R., Kazi, A. G., Hashem, A., Abd-Allah, E. F., Alqarawi, A. A. et al. (2016). Medicinal plants under abiotic stress: An overview. In: Plant-environment interaction: Responses and approaches to mitigate stress, pp. 300–310. [Google Scholar]
14. Ahmad, J., Bashir, H., Bagheri, R., Baig, A., Al-Huqail, A. et al. (2017). Drought and salinity induced changes in ecophysiology and proteomic profile of Parthenium hysterophorus. PLoS One, 12(9), e0185118. [Google Scholar] [PubMed]
15. Fang, Y., Xiong, L. (2015). General mechanisms of drought response and their application in drought resistance improvement in plants. Cellular and Molecular Life Sciences, 72(4), 673–689. https://doi.org/10.1007/s00018-014-1767-0 [Google Scholar] [PubMed] [CrossRef]
16. Qureshi, M. I., Abdin, M. Z., Ahmad, J., Iqbal, M. (2013). Effect of long-term salinity on cellular antioxidants, compatible solute and fatty acid profile of Sweet Annie (Artemisia annua L.). Phytochemistry, 95(167), 215–223. [Google Scholar] [PubMed]
17. Aljabre, S. H. M., Randhawa, M. A., Akhtar, N., Alakloby, O. M., Alqurashi, A. M. et al. (2005). Antidermatophyte activity of ether extract of Nigella sativa and its active principle, thymoquinone. Journal of Ethnopharmacology, 101(1–3), 116–119. [Google Scholar] [PubMed]
18. Houghton, P. J., Zarka, R., de las Heras, B., Hoult, J. R. S. (1995). Fixed oil of Nigella sativa and derived thymoquinone inhibit eicosanoid generation in leukocytes and membrane lipid peroxidation. Planta Medica, 61(1), 33–36. [Google Scholar] [PubMed]
19. Hashem, M. A., Mohamed, W. A. M., Attia, E. S. M. (2018). Assessment of protective potential of Nigella sativa oil against carbendazim- and/or mancozeb-induced hematotoxicity, hepatotoxicity, and genotoxicity. Environmental Science and Pollution Research International, 25(2), 1270–1282. https://doi.org/10.1007/s11356-017-0542-9 [Google Scholar] [PubMed] [CrossRef]
20. Xiao, J. F., Zhou, B., Ressom, H. W. (2012). Metabolite identification and quantitation in LC-MS/MS-based metabolomics. TrAC Trends in Analytical Chemistry, 32, 1–14. https://doi.org/10.1016/j.trac.2011.08.009 [Google Scholar] [PubMed] [CrossRef]
21. Hu, Y., Cai, B., Huan, T. (2019). Enhancing metabolome coverage in data-dependent LC-MS/MS analysis through an integrated feature extraction strategy. Analytical Chemistry, 91(22), 14433–14441. [Google Scholar] [PubMed]
22. Bian, X., Qian, Y., Tan, B., Li, K., Hong, X. et al. (2020). In-depth mapping carboxylic acid metabolome reveals the potential biomarkers in colorectal cancer through characteristic fragment ions and metabolic flux. Analytica Chimica Acta, 1128, 62–71. [Google Scholar] [PubMed]
23. Bradford, M. M. (1976). A rapid and sensitive method for the quantitation of microgram quantities of protein utilizing the principle of protein-dye binding. Analytical Biochemistry, 72(1-2), 248–254. https://doi.org/10.1016/0003-2697(76)90527-3 [Google Scholar] [CrossRef]
24. Heath, R. L., Packer, L. (1968). Photoperoxidation in isolated chloroplasts. I. Kinetics and stoichiometry of fatty acid peroxidation. Archives of Biochemistry and Biophysics, 125(1), 189–198. https://doi.org/10.1016/0003-9861(68)90654-1 [Google Scholar] [PubMed] [CrossRef]
25. Bates, L. S., Waldren, R. P., Teare, I. D. (1973). Rapid determination of free proline for water-stress studies. Plant and Soil, 39(1), 205–207. https://doi.org/10.1007/BF00018060 [Google Scholar] [CrossRef]
26. Gaitonde, M. K. (1967). A spectrophotometric method for the direct determination of cysteine in the presence of other naturally occurring amino acids. The Biochemical Journal, 104(2), 627–633. https://doi.org/10.1042/bj1040627 [Google Scholar] [PubMed] [CrossRef]
27. Scarpeci, T. E., Zanor, M. I., Carrillo, N., Mueller-Roeber, B., Valle, E. M. (2008). Generation of superoxide anion in chloroplasts of Arabidopsis thaliana during active photosynthesis: A focus on rapidly induced genes. Plant Molecular Biology, 66(4), 361–378. [Google Scholar] [PubMed]
28. Bashir, H., Ibrahim, M. M., Bagheri, R., Ahmad, J., Arif, I. A. et al. (2015). Influence of sulfur and cadmium on antioxidants, phytochelatins and growth in Indian mustard. AoB Plants, 7, plv001. https://doi.org/10.1093/aobpla/plv001 [Google Scholar] [PubMed] [CrossRef]
29. Dhindsa, R. S., Plumb-Dhindsa, P. L., Thorpe, T. A. (1981). Leaf senescence: Correlated with increased levels of membrane permeability and lipid peroxidation, and decreased levels of superoxide dismutase and catalase. Journal of Experimental Botany, 32(1), 93–101. [Google Scholar]
30. Nakano, Y., Asada, K. (1981). Hydrogen peroxide is scavenged by ascorbate-specific peroxidase in spinach chloroplasts. Plant and Cell Physiology, 22, 867–880. [Google Scholar]
31. Connell, J. P., Mullet, J. E. (1986). Pea chloroplast glutathione reductase: Purification and characterization. Plant physiology, 82(2), 351–356. https://doi.org/10.1104/pp.82.2.351 [Google Scholar] [PubMed] [CrossRef]
32. Aebi, H. (1984). Catalase in vitro. Methods in Enzymology, 105, 121–126. https://doi.org/10.1016/S0076-6879(84)05016-3 [Google Scholar] [PubMed] [CrossRef]
33. Habig, W. H., Jakoby, W. B. (1981). Assays for differentiation of glutathione S-Transferases. Methods in Enzymology, 77, 398–405. [Google Scholar] [PubMed]
34. Wilson, L. G., Bandurski, R. S. (1958). Enzymatic reactions involving sulfate, sulfite, selenate, and molybdate. Journal of Biological Chemistry, 233(4), 975–981. [Google Scholar] [PubMed]
35. Apak, R., Güçlü, K., Ozyürek, M., Karademir, S. E. (2004). Novel total antioxidant capacity index for dietary polyphenols and vitamins C and E, using their cupric ion reducing capability in the presence of neocuproine: CUPRAC method. Journal of Agricultural and Food Chemistry, 52(26), 7970–7981. https://doi.org/10.1021/jf048741x [Google Scholar] [PubMed] [CrossRef]
36. Botnick, I., Xue, W., Bar, E., Ibdah, M., Schwartz, A. et al. (2012). Distribution of primary and specialized metabolites in Nigella sativa seeds, a spice with vast traditional and historical uses. Molecules, 17(9), 10159–10177. https://doi.org/10.3390/molecules170910159 [Google Scholar] [PubMed] [CrossRef]
37. Farag, M. A., Saad, H. H., Hegazi, N. M. (2021). Rediscovering Nigella seeds bioactives chemical composition using metabolomics technologies. In: Fawzy Ramadan, M. (Ed.Black cumin (Nigella sativa) seeds: Chemistry, technology, functionality, and applications, pp. 131–151. Cham: Springer. https://doi.org/10.1007/978-3-030-48798-0_10 [Google Scholar] [CrossRef]
38. Garde-Cerdán, T., Gonzalo-Diago, A. (2016). Kaempferol: Biosynthesis, food sources and therapeutic uses. UK: Nova Science Publishers Inc. [Google Scholar]
39. Ren, J., Lu, Y., Qian, Y., Chen, B., Wu, T. et al. (2019). Recent progress regarding kaempferol for the treatment of various diseases. Experimental and Therapeutic Medicine, 18(4), 2759–2776. https://doi.org/10.3892/etm.2019.7886 [Google Scholar] [PubMed] [CrossRef]
40. Li, R., Zhou, Y., Zhang, S., Li, J., Zheng, Y. et al. (2022). The natural (poly) phenols as modulators of microglia polarization via TLR4/NF-κB pathway exert anti-inflammatory activity in ischemic stroke. European Journal of Pharmacology, 914, 174660. [Google Scholar] [PubMed]
41. Teixeira, J., Gaspar, A., Garrido, E. M., Garrido, J., Borges, F. (2013). Hydroxycinnamic acid antioxidants: An electrochemical overview. BioMed Research International, 2013, 251754. https://doi.org/10.1155/2013/251754 [Google Scholar] [PubMed] [CrossRef]
42. Sharma, P., Singh, R. (2012). Efficacy of trans-2-hydroxycinnamic acid against trichlorfon-induced oxidative stress in wistar rats. Toxicology International, 19(3), 295–300. https://doi.org/10.4103/0971-6580.103671 [Google Scholar] [PubMed] [CrossRef]
43. Patil, M., Choudhari, A. S., Pandita, S., Islam, M. A., Raina, P. et al. (2017). Cinnamaldehyde, cinnamic acid, and cinnamyl alcohol, the bioactives of cinnamomum cassia exhibit HDAC8 inhibitory activity: An in vitro and in silico study. Pharmacognosy Magazine, 13(Suppl 3), S645–S651. https://doi.org/10.4103/pm.pm_389_16 [Google Scholar] [PubMed] [CrossRef]
44. Flamini, R., Mattivi, F., Rosso, M. D., Arapitsas, P., Bavaresco, L. (2013). Advanced knowledge of three important classes of grape phenolics: Anthocyanins, stilbenes and flavonols. International Journal of Molecular Sciences, 14(10), 19651–19669. [Google Scholar] [PubMed]
45. Flamini, R., De Rosso, M., Panighel, A. (2016). Kaempferol in grape and wine. In: Garde-Cerdán, T., Diago, A. G. (Eds.Kaempferol: Biosynthesis, food sources and therapeutic uses. UK: Nova Science Publishers Inc. [Google Scholar]
46. Xue, W., Wang, X., Tang, H., Sun, F., Zhu, H. et al. (2020). Vitexin attenuates myocardial ischemia/reperfusion injury in rats by regulating mitochondrial dysfunction induced by mitochondrial dynamics imbalance. Biomedicine & Pharmacotherapy, 124(1), 109849. https://doi.org/10.1016/j.biopha.2020.109849 [Google Scholar] [PubMed] [CrossRef]
47. de Oliveira, D. D., da Silva, C. P., Iglesias, B. B., Beleboni, R. O. (2020). Vitexin possesses anticonvulsant and anxiolytic-like effects in murine animal models. Frontiers in Pharmacology, 11, 1181. https://doi.org/10.3389/fphar.2020.01181 [Google Scholar] [PubMed] [CrossRef]
48. Abbasi, E., Nassiri-Asl, M., Shafeei, M., Sheikhi, M. (2012). Neuroprotective effects of vitexin, a flavonoid, on pentylenetetrazole-induced seizure in rats. Chemical Biology & Drug Design, 80(2), 274–278. https://doi.org/10.1111/j.1747-0285.2012.01400.x [Google Scholar] [PubMed] [CrossRef]
49. He, M., Min, J. W., Kong, W. L., He, X. H., Li, J. X. et al. (2016). A review on the pharmacological effects of vitexin and isovitexin. Fitoterapia, 115(4), 74–85. https://doi.org/10.1016/j.fitote.2016.09.011 [Google Scholar] [PubMed] [CrossRef]
50. Xu, D., Hu, M. J., Wang, Y. Q., Cui, Y. L. (2019). Antioxidant activities of quercetin and its complexes for medicinal application. Molecules, 24(6), 1123. https://doi.org/10.3390/molecules24061123 [Google Scholar] [PubMed] [CrossRef]
51. Shaik, Y. B., Castellani, M. L., Perrella, A., Conti, F., Salini, V. et al. (2006). Role of quercetin (a natural herbal compound) in allergy and inflammation. Journal of Biological Regulators and Homeostatic Agents, 20(3–4), 47–52. [Google Scholar] [PubMed]
52. Ali, F. T., Hassan, N. S., Abdrabou, R. R. (2016). Hepatoprotective and antiproliferative activity of moringinine, chlorogenic acid and quercetin. International Journal of Research in Medical Sciences, 4(4), 1147–1153. https://doi.org/10.18203/2320-6012.ijrms20160799 [Google Scholar] [CrossRef]
53. Mehrbod, P., Ebrahimi, S. N., Fotouhi, F., Eskandari, F., Eloff, J. N. et al. (2019). Experimental validation and computational modeling of anti-influenza effects of quercetin-3-O-α-L-rhamnopyranoside from indigenous South African medicinal plant Rapanea melanophloeos. BMC Complementary and Alternative Medicine, 19(1), 346. https://doi.org/10.1186/s12906-019-2774-3 [Google Scholar] [PubMed] [CrossRef]
54. Sun, Y., Li, L. (2018). Cyanidin-3-glucoside inhibits inflammatory activities in human fibroblast-like synoviocytes and in mice with collagen-induced arthritis. Clinical and Experimental Pharmacology & Physiology, 45(10), 1038–1045. https://doi.org/10.1111/1440-1681.12970 [Google Scholar] [PubMed] [CrossRef]
55. Xiong, Y., Qiu, J., Li, C., Qiu, Y., Guo, L. et al. (2018). Fortunellin-induced modulation of phosphatase and tensin homolog by MicroRNA-374a decreases inflammation and maintains intestinal barrier function in colitis. Frontiers in Immunology, 9, 83. https://doi.org/10.3389/fimmu.2018.00083 [Google Scholar] [PubMed] [CrossRef]
56. Feng, Y., Dunshea, F. R., Suleria, H. A. R. (2020). LC-ESI-QTOF/MS characterization of bioactive compounds from black spices and their potential antioxidant activities. Journal of Food Science and Technology, 57(12), 4671–4687. [Google Scholar] [PubMed]
57. Feng, X., Li, Y., Guang, C., Qiao, M., Wang, T. et al. (2018). Characterization of the in vivo and in vitro metabolites of linarin in rat biosamples and intestinal flora using ultra-high performance liquid chromatography coupled with quadrupole time-of-flight tandem mass spectrometry. Molecules, 23(9), 2140. https://doi.org/10.3390/molecules23092140 [Google Scholar] [PubMed] [CrossRef]
58. Hsu, C. C., Lin, M. H., Cheng, J. T., Wu, M. C. (2017). Diosmin, a citrus nutrient, activates imidazoline receptors to alleviate blood glucose and lipids in type 1-like diabetic rats. Nutrients, 9(7), 684. https://doi.org/10.3390/nu9070684 [Google Scholar] [PubMed] [CrossRef]
59. Khanna, S., Roy, S., Slivka, A., Craft, T. K., Chaki, S. et al. (2005). Neuroprotective properties of the natural vitamin E α-tocotrienol. Stroke, 36(10), 2258–2264. https://doi.org/10.1161/01.STR.0000181082.70763.22 [Google Scholar] [PubMed] [CrossRef]
60. Hasan, M. M., Alharby, H. F., Hajar, A., Hakeem, K. R. (2017). Leaf gas exchange, Fv/Fm ratio, ion content and growth conditions of the two moringa species under magnetic water treatment. Pakistan Journal of Botany, 49(3), 921–928. [Google Scholar]
61. Fu, Y., Ma, H., Chen, S., Gu, T., Gong, J. (2018). Control of proline accumulation under drought via a novel pathway comprising the histone methylase CAU1 and the transcription factor ANAC055. Journal of Experimental Botany, 69(3), 579–588. https://doi.org/10.1093/jxb/erx419 [Google Scholar] [PubMed] [CrossRef]
62. Hell, R., Wirtz, M. (2008). Metabolism of cysteine in plants and phototrophic bacteria. In: Hell, R., Dahl, C., Knaff, D., Leustek, T. (Eds.Sulfur metabolism in phototrophic organisms, pp. 59–91. Dordrecht: Springer. https://doi.org/10.1007/978-1-4020-6863-8_4 [Google Scholar] [CrossRef]
63. Lallement, P. A., Brouwer, B., Keech, O., Hecker, A., andRouhier, N. (2014). The still mysterious roles of cysteine-containing glutathione transferases in plants. Frontiers in Pharmacology, 5, 192. https://doi.org/10.3389/fphar.2014.00192 [Google Scholar] [PubMed] [CrossRef]
64. Cruz de Carvalho, M. H. (2008). Drought stress and reactive oxygen species: Production, scavenging and signaling. Plant Signaling & Behavior, 3(3), 156–65. https://doi.org/10.4161/psb.3.3.5536 [Google Scholar] [PubMed] [CrossRef]
65. Dusenge, M. E., Duarte, A. G., Way, D. A. (2019). Plant carbon metabolism and climate change: Elevated CO2 and temperature impacts on photosynthesis, photorespiration and respiration. The New Phytologist, 221(1), 32–49. https://doi.org/10.1111/nph.15283 [Google Scholar] [PubMed] [CrossRef]
66. Baslam, M., Mitsui, T., Hodges, M., Priesack, E., Herritt, M. T. et al. (2020). Photosynthesis in a changing global climate: Scaling up and scaling down in crops. Frontiers in Plant Science, 11, 882. https://doi.org/10.3389/fpls.2020.00882 [Google Scholar] [PubMed] [CrossRef]
67. Ansari, W. A., Atri, N., Ahmad, J., Qureshi, M. I., Singh, B. et al. (2019). Drought mediated physiological and molecular changes in muskmelon (Cucumis melo L.). PLoS One, 14(9), e0222647. https://doi.org/10.1371/journal.pone.0222647 [Google Scholar] [PubMed] [CrossRef]
68. Kabiri, R., Farahbakhsh, H., Nasibi, F. (2012). Salicylic acid ameliorates the effects of oxidative stress induced by water deficit in hydroponic culture of Nigella sativa. Journal of Stress Physiology & Biochemistry, 3, 13–22. https://core.ac.uk/download/pdf/26988608.pdf [Google Scholar]
69. Bashir, H., Ahmad, J., Bagheri, R., Nauman, M., Qureshi, M. I. (2013). Limited sulfur resource forces Arabidopsis thaliana to shift towards non-sulfur tolerance under cadmium stress. Environmental and Experimental Botany, 94, 19–32. https://doi.org/10.1016/j.envexpbot.2012.05.004 [Google Scholar] [CrossRef]
Cite This Article
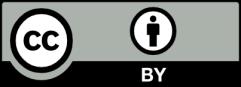
This work is licensed under a Creative Commons Attribution 4.0 International License , which permits unrestricted use, distribution, and reproduction in any medium, provided the original work is properly cited.