Open Access
ARTICLE
Genotypic Diversity Affects Age Structure of Tiller and Rhizome of Leymus chinensis Population, But Not Age Structure of Bud
1 School of Life Science, Liaoning University, Shenyang, 110036, China
2 School of Life Science and Bioengineering, Shenyang University, Shenyang, 110044, China
3 Research Institute of Sand Control and Utilization, Fuxin, 123000, China
4 State Key Laboratory of Forest and Soil Ecology, Institute of Applied Ecology, Chinese Academy of Sciences, Shenyang, 110164, China
* Corresponding Author: Chan Zhou. Email:
(This article belongs to the Special Issue: Symbiotic Associations for Nutrients Management and Complexes Formation for Better Agricultural Crops Productivity under Biotic and Abiotic Stresses)
Phyton-International Journal of Experimental Botany 2023, 92(11), 3163-3176. https://doi.org/10.32604/phyton.2023.030245
Received 28 March 2023; Accepted 08 June 2023; Issue published 24 October 2023
Abstract
The effect of genotypic diversity on the age structure and bud bank of the Leymus chinensis population has not yet been demonstrated. This experiment was designed to study the age structure of the tiller, rhizome, and bud of L. chinensis at genotypic diversity levels of 1, 2, 4, 8, and 12. A total of 64 communities were established in this experiment, following the principle of randomized block experimental design. The results indicated that genotypic diversity had a significant or obviously significant effect on the cumulative length of rhizomes of 2a and 4a, dry matter accumulation of rhizomes of 1a and 2a, tiller number of 1a, tiller productivity of 3a, but had no significant effect age structure of buds. As the levels of genotypic diversity increased, the proportion of the cumulative length of rhizomes with 4a decreased and then increased, and dry matter accumulation of rhizomes with 1a and 2a gradually increased while the number of tillers with 1a gradually decreased, when the gradient of genotypic diversity increased. At the 1, 2, 4, 8, and 12 genotype diversity levels, the number of tillers, the cumulative length of rhizomes, and dry matter accumulation in rhizomes and buds were all age structures of expanding type. However, tiller productivity was an expanding or stable age structure at levels of 1, 2, 4, 8 genotypic diversity, while it was a declining age structure at the 12 genotype diversity gradient. An appropriate genotypic diversity is conducive to maintaining the growth and stability of the age structure of L. chinensis population, but too high a gradient of genotypic diversity can have a negative impact on the population age structure. This study provided that an appropriate number of genotypic diversity contributes to the stability of the population.Keywords
In recent years, the ecological importance of genotypic diversity has aroused extensive concerns of ecologists [1–4]. There are abundant experiments demonstrating that the researches concerning genotypic diversity mainly focuses on its effects on population stability and productivity [5–9]. Gebregerges et al. found that the construction of a seagrass bed by Zostera marina with a low genotypic diversity gradient was difficult to succeed, with low fitness of individuals. The time required for recovery of decreased with increasing genotypic diversity, and the community would pick up stronger anti-interference capacity [10]. Kotowska et al. directly demonstrated that whether in the presence of herbivores or not, higher genotypic diversity of Arabidopsis thaliana led to higher plant productivity [11]. Yang et al. also showed Stipa grandis population of multi-genotypes had higher productivity than that of uni-genotype. The above-ground biomass of Stipa grandis population in high genotypic diversity was significantly higher than the average biomass in monocultures genotypic diversity. In consequence, genotypic diversity played an important role in influencing above ground biomass [12]. Therefore, genotypic diversity can have an important impact on population production and population development. Despite the great progress in exploring the role of genotypic diversity in the population, further experimental research is still badly needed.
The age structure of the plant population can reflect the combination of individuals of different ages, demography, and developing trends [13,14]. During the past three decades, the research into the age structure of plant populations has focused on the methods of age structure discrimination [15–17] and the investigation into the age structure of different species in the natural environment [18–20]. Generally, age structure is used to predict the population dynamics of dominant and endangered species and to predict the population developing trend [21–23]. The age structure of the population is mainly characterized by an increasing, decreasing, or stable structure. However, whether and how genotypic diversity of the population affects age structure has never been reported. The efficiency of resource utilization of individuals with different genotypes in populations is various, which leads to the diversity of nutrient utilization and accumulation in populations. Under the condition of limited resources, the younger or older plants have different abilities of vitality to take up nutrients. The younger or older plants have different numbers and accumulation biomass. Consequently, the changes in numbers and biomass in younger or older plants formed the variations population age structure [24,25]. Therefore, studying the effects of genotypic diversity on the age structure of population can predict population dynamics and development by examining the proportion and allocation of individuals with different ages in the population, and then exploring population response to genotypic diversity.
The bud bank is the collection of all potential buds with a capacity for vegetative propagation [26]. The propagation system of plants consists of a bud bank and seed bank and together play a crucial role in the maintenance and regeneration of vegetation. The influence of environmental factors on the establishment and dynamics of bud bank becomes a hot research issue, including fire, moisture, soil conditions, light, and so on [27–30]. Benson et al. studied the effects of two different fire frequencies on the bud banks in North American tallgrass prairie. Their results demonstrated that the bud bank size of grass was significantly greater in the grassland burned once a year than in the grassland burned once every 20 years, which was compared with the forb bud banks, contributing significantly to different vegetation responses to fire [31]. Bravo et al. found that rhizome buds were more susceptible to soil moisture than tiller buds, which also further demonstrated that plants could survive the harsh environment by regulating the pattern of bud banks [32]. Despite that, some researchers suggested that genotypic diversity can enhance the anti-interference ability and adaptability of plants to environmental factors, yet the effect of genotypic diversity on bud banks has not been studied in detail [33–35].
L. chinensis is a typical rhizomatous, perennial grass with such ecological characteristics as cold tolerance, drought tolerance, and salinity tolerance [15,19], and is an excellent forage grass widely distributed in eastern Inner Mongolia and Western Northeast China [36,37]. There are different levels of genotypic diversity in L. chinensis population in different regions, and the number of genotypes varies among populations in different habitats. There are differences in traits and nutrient acquisition capacity among different genotypes of L. chinensis. A variety of different genotypes of L. chinensis constitute the population genotypic diversity. Genotypic diversity is believed to contribute to the resource use efficiency of populations because of complementary effects among individual plants of different genotypes due to their different functional traits and resource-acquiring capabilities. As genotypic diversity increases, population fitness, anti-interference ability and the success rate of population expansion increase [38–40]. It has shown that genotypic diversity could not only improve the performance of L. chinensis population, but also enhance their anti-interference capabilities [34,41,42]. However, the effect of genotypic diversity on the age structure of L. chinensis population is not undertaken.
To investigate the impact of genotypic diversity on the age structure and bud bank of L. chinensis population, we conducted a manipulative experiment in which five levels of genotypic diversity of L. chinensis population were artificially constructed. This experiment aims to test the following two scientific issues: 1) What is the impact of genotypic diversity on the number and biomass of tillers, rhizome and bud of L. chinensis; 2) What is the impact of genotypic diversity on of tillers, rhizome and bud of age structure of L. chinensis. This study provides theoretical support for the scientific management of L. chinensis grassland.
The experimental site is located in the Institute of Liaoning Aeolian Sandy Land Improvement and Utilization (42.42′N, 122.31′E). It is located in the northern temperate zone, with a temperate continental monsoon climate, characterized by cold, dry winters and hot, humid summers. The minimum temperature in winter is −29.5°C and the maximum temperature in summer is 35.2°C. The average annual temperature is about 5.7°C and the frost-free period is about 156 days. The precipitation is the least in winter and the most in summer. The annual average precipitation is about 480 mm, while the annual evaporation is as high as about 1600 mm [43]. Aeolian sandy soils are widespread, with uniformly sized sand particles and low soil nutrient content. The annual average wind speed is about 4.5 m/s, with dusty and sandy weather in spring.
We established twelve 3 × 3 m L. chinensis genotypic cultivation areas at experimental site in 2012. Tiller in each cultivation is propagated with rhizome in order to obtain a large number of ramets of the same genotype. Twelve genotypes of L. chinensis was obtained from twelve different sites in the mainly distribution area of L. chinensis in China, and then one tiller of each genotype was transplanted to the twelve cultivation areas respectively. They were managed and raised under the same conditions. Twelve genotypes of L. chinensis were identified and labelled by Simple Sequence Repeat [44].
In the experiment, L. chinensis population composed of 1, 2, 4, 8 and 12 genotypic was constructed [45], and were replicated by 24, 12, 9, 9 and 10 times, respectively, gave rise to altogether 64 populations, which were assigned to 64 2 m × 2 m experimental plots. Ramet number in each plot was 12 and different genotypes had the same abundance in multi-genotype plots. In a mono-genotype, there are two replicates in each plot, with 12 replicates in each plot, resulting in a total of 24 mono-genotype replicates. Among the 12 genotypic diversities, each plot has 12 ramet combinations, with 10 plots are repeated, resulting in a total of 10 genotypic diversity replicates. The assignment of the 64 populations followed the principles of a randomized block experimental design, and the genotypes for multi-genotypic treatments were randomly selected by reference to Excel random number [45]. To eliminate the interference of genotypic differences in L. chinensis, the experiment was designed to has consistent number of occurrences for each genotype at the same gradient of genotypic diversity and even in the 64 experimental plots. In addition, ramet with each genotype was arranged in different locations in different experimental plots of the same genotypic diversity gradient, and the chances of being adjacent to other genotypes are the same [45].
In May 2014, 1st age class ramets of 12 different genotypes with uniform height and size were selected and were separated from the rhizomes, and then transplanted into each experimental plot according to experimental design. 12 ramets in the same plot were transplanted with grid like equidistant spacing method. Replant any dead ramet within a week after transplanting until they are fully survival. After five consecutive years of natural recovery and growth, the population had reached a relatively stable status. In September 2019, one small quadrat with 25 cm × 25 cm × 30 cm size in all plots were sampled, keeping the natural connection between the rhizomes and the plants and the integrity of all shoots.
All samples collected were taken back to the laboratory to classify the age of the tillers, rhizomes and buds. According to the growth years of tiller node and rhizome, tillers, rhizome and bud were divided into different age classes. A tiller growing from the top of the rhizome or from the rhizome internode upwards is the age one (1a) tiller, a tiller growing from a 1a tiller node is a 2a, a tiller from a 2a tiller node is a 3a, and so on. All new rhizomes formed during the current season are referred to as 1a rhizomes, the rhizomes grown from 1a tillers as 2a rhizomes, and so on. Rhizome terminal buds at each age of tiller node apical buds and rhizome node buds are 0a, upward growing buds from 1a tiller nodes are 1a buds, upward growing buds from 2a tiller nodes are 2a buds, and so on [17]. The tillers and rhizome were placed into perforated paper bags and oven-dried at 80°C to constant weight and biomass was weighed. Dry matter accumulation of rhizome was measured as the cumulative length 100 cm of rhizome biomass. Percentages of age structure were calculated according to the number and biomass of tillers, rhizomes and buds at different age classes.
The means and standard deviation of each index were analyzed using SPSS 25.0 One-way ANOVA analysis was also used to test the significance of genotypic diversity on the number and productivity of tillers, the cumulative length of rhizomes and dry matter accumulation in rhizome, and the number of buds of L. chinensis. The means and standard deviation of each index was plotted as bar charts using Origin 2021.
3.1 Age Structure of the Number of Tillers
Irrespective of genotypic diversity level, tillers of 1a accounted for the largest proportion, followed by tillers of 2a, and then those of 3a and 4a (Table 1). This indicated that the newly emerged tillers dominated the populations of all the genotypic diversity levels and therefore the age structure was generally consistent, showing an expanding age structure. Increasing genotypic diversity resulted in a gradual decrease in the tiller number of 1a, 2a and 3a and an increasing trend in 4a (Fig. 1A). The effect of genotypic diversity for L. chinensis on the number of tillers varied across age classes, with a highly significant effect on the tiller number of 1a (p < 0.05) (Table 2).
Figure 1: Effects of different genotypic diversity gradient on (A) number of tillers of L. chinensis; (B) tiller productivity; (C) the cumulative length of rhizomes; (D) dry matter accumulation in rhizome
3.2 Age Structure of Tiller Productivity
The populations composed of 1 and 2 genotypes were dominated by 1a and 2a tiller productivity, showing expanding age structure. However, for the populations composed of 12 genotypes, the productivity of 1a, 2a and 3a tillers was lower than that of 4a tillers, showing a declining age structure. Tiller productivity at 12 genotypic diversity levels showed a declining age structure. For the populations composed of 4 and 8 genotypes, a relatively balanced production among the four age classes suggested a stable age structure (Fig. 1B). As the levels of genotypic diversity increased, the productivity of 1a and 4a tillers tended to increase, while the productivity of 2a and 3a tillers increased first and then decreased. Irrespective of age classes, the productivity of tillers in the treatment of 4 genotypes is higher than any other genotypic diversity levels (Table 1). One-way ANOVA showed a significant effect of genotypic diversity on 3a tiller productivity (p < 0.01) (Table 2).
3.3 Age Structure of the Cumulative Length of Rhizomes
For the populations composed of 1, 2, 4 and 8 genotypes, the length of 1a rhizomes was accounted for larger proportion than that of 2a rhizomes, and that of 3a and 4a rhizomes was much lower than that of 2a rhizomes (Table 1). Although the length of 4a rhizomes accounted for larger proportion than that of 1a, 2a and 3a rhizomes for the treatment of 12 genotypes, yet the length of young rhizomes generally accounted for significantly larger proportion than old rhizomes, therefore the age structure of their rhizomes was also expanding type. Our results demonstrated that L. chinensis had a high capacity to reproduce via rhizomes and that the rhizome length of all the age classes showed expanding age structure. With the increasing genotypic diversity, the proportion of 1a and 2a rhizome length increased first and then decreased, while the proportion of 3a and 4a rhizome length decreased first and then increased (Fig. 1C). One-way ANOVA indicated that genotypic diversity played a significant effect on the cumulative length of rhizomes with 2a (p < 0.05) and an extremely significant effect on 4a (p < 0.01) (Table 2).
3.4 Age Structure of Dry Matter Accumulation in Rhizome
Irrespective of genotypic diversity level, rhizome dry matter decreased with the rhizome age class, and thus rhizome dry matter accumulated in the current year dominate, suggestive of an expanding age structure (Table 1). The results revealed that dry matter accumulation of rhizome in younger rhizome was consistently dominant among all age classes. Age structure of dry matter accumulation in rhizomes showed uniformly expanding age structure at any genotypic diversity gradient. With the increasing genotypic diversity, the dry matter accumulation of 1a and 2a rhizomes gradually increased, while those of 3a and 4a rhizomes increased first and then decreased. But in general, genotypic diversity significantly promoted average rhizome dry matter accumulation (Fig. 1D). Analysis of variance (ANOVA) revealed that genotypic diversity showed extremely significant effects on dry matter accumulation of 1a and 2a rhizomes (p < 0.01) (Table 2).
Irrespective of genotypic diversity, 0a and 1a buds accounted for greater proportion than the older buds, including those of 2a, 3a and 4a, therefore, the age structure of the buds was consistently expanding type across all the five genotypic diversity levels (Fig. 2). With the increasing genotypic diversity except for that of 12 genotypes, the proportion of 0a buds decreased, while that of 1a and 2a buds increased, and the proportion of 3a buds decreased first and then increased. The proportion of 4a buds increased and then decreased, and there were no 4a buds in the 12 genotype diversity levels (Table 3). One-way ANOVA declared that genotype diversity had little effect on bud bank size of the populations (Table 4).
Figure 2: Effects of different genotypic diversity gradient on number of buds at different ages
4.1 Effects of Genotypic Diversity on Age Structure of L. chinensis Tillers in Terms of Number and Productivity
Age structure of the population for each of the five genotypic diversity levels consisted of 1a, 2a, 3a and 4a tillers, and the number of tillers tended to decrease with their age class. Firstly, L. chinensis is a perennial grass that relies mainly on rhizome for reproduction. Younger tiller nodes and rhizome nodes are the most capable of producing buds and seedlings. Therefore L. chinensis population produced the highest proportion of younger tillers [46,47]. Secondly, the reproductive capacity of tiller nodes was weaker than that of rhizomes, and the reproduction ability of tiller nodes decreased with the increase of surviving generations, so the number of young tillers was more than that of older tillers [46,48,49]. Except for the 4a tillers, 1a, 2a and 3a tillers decreased gradually as genotypic diversity increased. This is because, given the limited nutrient material and growing space, too high genotypic diversity increases competition among the L. chinensis population and limits the growth of the dominant genes in the population, when the negative effect of selection plays a major role in the increase of genotypic diversity [42,50,51]. Among the 4 and 8 levels of genotypic diversity, the population can grow and reproduce stably.
Productivity in the 1 and 2 genotypic diversity levels appears an expanding age structure. In other words, L. chinensis had a greater capacity for growth and reproduction, with more space for population development and a faster growth rate at the 1 and 2 genotypic diversity levels. It is explained by the fact that 1a and 2a tillers have a higher capacity to obtain nutrients and can accumulate more nutrients for the population to grow and reproduce. The age structure of tiller productivity was stable in 4 and 8 genotypic diversity levels, and the productivity of 2a and 3a tillers was relatively larger than those in other age classes. It indicated that the population can grow and reproduce relatively stable in the two genotypic diversity levels. This is due to the nutrient accumulation by tiller buds in the previous year, enabling 2a and 3a tillers relatively high productivity. However, the age structure of tiller productivity in 12 genotypic diversity levels showed a declining age structure, due to the negative effect of selection effect on genotypic diversity [52–54]. The increase in genotypic diversity led to an increasing trend in the productivity of 1a and 4a tillers, while the productivity of 2a and 3a tillers tended to increase first and then decrease. It indicated that the selection effect had a positive effect on the productivity of 1a tillers in order to make them have more capable of growth and reproduction gradually [2,55]. Even though the 4a tillers also showed an increasing trend in biomass, 4a tiller productivity was relatively lower and the population was less competitive. It suggested that appropriate genotypic diversity could promote population productivity, but too high genotypic diversity might have a negative impact on population productivity.
4.2 Effect of Genotypic Diversity on the Age Structure of Length and Dry Matter Accumulation in Rhizome
The cumulative length of rhizomes in the 1, 2, 4, 8 and 12 genotypic diversity levels all consisted of that from all the four age classes. The cumulative length of rhizomes with 1a accounted for the highest proportion, leading to the expanding age structure for all the five genotypic diversity levels. This is because 1a rhizomes, as newborn rhizomes in the current year, are more capable of growth and reproduction and dominated the population in terms of age structure in the cumulative length of rhizomes. With the increase of genotypic diversity, the proportion of length of 1a and 2a rhizomes increased first and then decreased, while that of 3a and 4a rhizomes decreased first and then increased. It reflected the expansion strategy of horizontally extended rhizome in L. chinensis population [56]. This may be due to the fact that a moderate increase in genotypic diversity promotes the growth and reproduction of younger rhizomes and the population shows an expansion strategy, which helped improve the asexual reproduction ability of the population [49,54,57]. However, too high genotypic diversity can inhibit horizontal extension of the young rhizomes and increase extension of the old rhizomes. The population showed signs of decline, which was not conducive to further population expansion. There were significant differences in the length of 2a rhizomes among genotypic diversity levels and extremely significant differences in the length of 4a rhizomes. This can be explained by the fact that 2a rhizomes are more vigorous in the current year after the previous year’s growth and nutrient accumulation, and therefore length of 2a rhizomes dominate in all the genotypic diversity levels except for the 12 genotypic diversity. The length of 4a rhizomes showed an increasing trend in the level of 12 genotypic diversity. It meant that rhizome may adopt a growth and reproduction strategy that biased towards maintaining population stability when genotypic diversity was too high, because L. chinensis population are highly resistant to external disturbances.
The age structure of dry matter accumulation in the rhizomes of all five genotypic diversity was increasing, and the dry matter accumulation of 1a and 2a rhizomes accounted for a larger proportion of the total compared to that of 3a and 4a rhizomes. The age structure of the buds shows an increasing type, which reflects the strong growth and reproduction ability of the five genotypes of L. chinensis and the fast population renewal speed. However, there were no 4a buds in all 12 genotypes, indicating that the population reflects more of the utilization of nutrients in younger buds, ensuring their growth and maintaining population development. The L. chinensis clones always transport more nutrients to the 1a and 2a rhizomes, promoting the accumulation of dry matter in the younger rhizomes and facilitating the lateral spatial expansion of the population’s rhizomes. Elevated genotypic diversity resulted in a significant proportional increase in dry matter accumulation of younger rhizomes and accordingly a proportional decrease in older rhizomes [56]. Because increased genotypic diversity could improve resource utilization and promote diversity of nutrient accumulation types for the younger rhizomes. But the old rhizomes are less vigorous and unable to take up more nutrients, and so dry matter accumulation was very limited [49,58]. There was an extremely significant difference on dry matter accumulation of 1a and 2a rhizomes among genotypic diversity levels, while there was no significant effect on those of 3a and 4a rhizomes. The obvious differences in the uptake capacity of rhizomes for various nutrients depended on different genotypic diversity levels. It was helpful to the accumulation of different nutrients by L. chinensis population. The positive effect of the complementarity effect obviously promoted genotype diversity to play an effect on the dry matter accumulation of rhizomes. Appropriate genotype diversity can promote the development of L. chinensis population, and is conducive to population growth and spatial expansion.
4.3 Effect of Genotypic Diversity on Bud Age Structure
The age structure of buds in the population represents their vegetative reproduction ability. In all five genotypic diversity levels, 0a and 1a buds were more predominant, and the bud age structure showed a growth pattern. This reflects that L. chinensis has a high reproductive capacity and a high rate of population renewal in the five genotypic diversity levels. The level of genotype diversity did not have a substantial impact on the structure of bud age. Although the bud age structure was growing in all five genotype diversity levels, the absence of 4a buds in the 12 genotypic diversity. In genotype rich communities, the old roots are chosen to give up participating in reproduction. The population tends to use new and more active roots for vegetative reproduction. At the same time, buds located on young roots can give the population a leading advantage in the growth of the next year [59]. However, the increase in genotypic diversity led to a decrease in the proportion of 0a and 4a buds, and an increase in the proportion of 1a and 2a buds. With the increase of genotype diversity, in addition to abandoning aging buds (4a), the population’s selection of buds does not seem to be inclined towards 0a. From the observation of actual root color at different ages, it can be inferred that the water content is the main component of the roots of 0a buds, while the roots of 1a and 2a buds are mainly composed of nutrients. In future research, we will conduct further research on root nutrients at different levels of genotype diversity in order to understand their underlying causes.
Experimental studies have found that L. chinensis maintained a relatively stable population gradient by regulating the number and age structure of tillers, rhizomes, and buds. A moderate increase in genotypic diversity positively affected the age of tillers number, tillers productivity, the cumulative length of rhizomes, rhizome dry matter accumulation, and buds in L. chinensis population. Among the five genotypic diversity levels, the age of tillers number, the cumulative length of rhizomes, dry matter accumulation, and buds in L. chinensis population were growing or stable age structures. However, the productivity of tillers at the 12 genotypic diversity gradient showed a declining age structure and the population showed a signal of decline. As a result, appropriate genotypic diversity facilitates the expansion of L. chinensis population, while increased genotypic diversity is more beneficial for the development of the population’s hibernation components. In the future, the study of genotypic diversity from multiple perspectives and dimensions will promote the understanding of the relationship between genotypic diversity and ecosystem function.
Acknowledgement: We thank Zoe Zhang and Sarayang for their critical proof check of the manuscript.
Funding Statement: This work was supported by the National Natural Science Foundation of China (32071860).
Author Contributions: The authors confirm their contribution to the paper as follows: study conception and design: C.Z., Z.Z., and Z.W.W.; data collection: W.J.R., and L.Y.L.; analysis and interpretation of results: Z.Z., N.N.X., W.J.R., and L.Y.L.; draft manuscript preparation: C.Z., N.N.X., and Z.W.W. All authors reviewed the results and approved the final version of the manuscript.
Availability of Data and Materials: All related information is available in this manuscript.
Ethics Approval: This manuscript does not contain experiments using animals. The manuscript does not contain human studies. Leymus chinensis is native to China and is currently widely grown in Northeast, Northwest and Inner Mongolia of China and the material in this study is in absolute compliance with relevant institutional, national and international guidelines and regulations. We recommend compliance with the IUCN Policy Statement on Research Involving Endangered Species and the Convention on Trade in Endangered Species of Wild Fauna and Flora.
Conflicts of Interest: The authors declare that they have no conflicts of interest to report regarding the present study.
References
1. Ehlers, A., Worm, B., Reusch, T. B. (2008). Importance of genetic diversity in eelgrass Zostera marina for its resilience to global warming. Marine Ecology Progress Series, 355, 1–7. https://doi.org/10.3354/meps07369 [Google Scholar] [CrossRef]
2. Hughes, A. R., Inouye, B. D., Johnson, M. T., Underwood, N., Vellend, M. (2008). Ecological consequences of genetic diversity. Ecology Letters, 11(6), 609–623. https://doi.org/10.1111/j.1461-0248.2008.01179.x [Google Scholar] [PubMed] [CrossRef]
3. Kettenring, K. M., Mercer, K. L., Reinhardt, A. C., Hines, J. (2014). EDITOR’S CHOICE: Application of genetic diversity-ecosystem function research to ecological restoration. Journal of Applied Ecology, 51(2), 339–348. https://doi.org/10.1111/1365-2664.12202 [Google Scholar] [CrossRef]
4. Abdala-Roberts, L., Mooney, K. A. (2014). Ecological and evolutionary consequences of plant genotype diversity in a tri-trophic system. Ecology, 95(10), 2879–2893. https://doi.org/10.1890/13-2029.1 [Google Scholar] [CrossRef]
5. Gebregerges, T., Tessema, Z. K., Birhane, E. (2018). Effect of exclosure ages on woody plant structure, diversity and regeneration potential in the Western Tigray region of Ethiopia. Journal of Forestry Research, 29(3), 697–707. https://doi.org/10.1007/s11676-017-0512-6 [Google Scholar] [CrossRef]
6. Puy, J., Carmona, C. P., Dvořáková, H., Latzel, V., de Bello, F. (2021). Diversity of parental environments increases phenotypic variation in Arabidopsis populations more than genetic diversity but similarly affects productivity. Annals of Botany, 127(4), 425–436. https://doi.org/10.1093/aob/mcaa100/5848278 [Google Scholar] [PubMed] [CrossRef]
7. Roger, F., Godhe, A., Gamfeldt, L. (2012). Genetic diversity and ecosystem functioning in the face of multiple stressors. PLoS One, 7(9), 1–10. https://doi.org/10.1371/journal.pone.0045007 [Google Scholar] [PubMed] [CrossRef]
8. Semchenko, M., Nettan, S., Sepp, A., Zhang, Q. Y., Abakumova, M. et al. (2019). Soil biota and chemical interactions promote co-existence in co-evolved grassland communities. Journal of Ecology, 107(6), 2611– 2622. https://doi.org/10.1007/s11104-021-04967-0 [Google Scholar] [CrossRef]
9. Vellend, M., Drummond, E. B., Muir, J. L. (2009). Ecological differentiation among genotypes of dandelions (Taraxacum officinale). Weed Science, 57(4), 410–416. https://doi.org/10.1614/WS-09-004.1 [Google Scholar] [CrossRef]
10. Hughes, A. R., Stachowicz, J. J. (2004). Genetic diversity enhances the resistance of a seagrass ecosystem to disturbance. Proceedings of the National Academy of Sciences of the United States of America, 101(24), 8998– 9002. [Google Scholar] [PubMed]
11. Kotowska, A. M., Cahill Jr, J. F., Keddie, B. A. (2010). Plant genetic diversity yields increased plant productivity and herbivore performance. Journal of Ecology, 98(1), 237–245. https://doi.org/10.1111/j.1365-2745.2009.01606.x [Google Scholar] [CrossRef]
12. Yang, X., Wang, X. P., Qu, Y. B., Wu, M., Wang, J. L. et al. (2019). Comparing the effects of companion species diversity and the dominant species (Stipa grandis) genotypic diversity on the biomass explained by plant functional trait. Ecological Engineering, 136, 17–22. https://doi.org/10.1016/j.ecoleng.2019.05.018 [Google Scholar] [CrossRef]
13. Anthony, N. M., Johnson-Bawe, M., Jeffery, K., Clifford, S. L., Abernethy, K. A. et al. (2007). The role of Pleistocene refugia and rivers in shaping gorilla genetic diversity in Central Africa. Proceedings of the National Academy of Sciences, 104(51), 20432–20436. https://doi.org/10.1073/PNAS.0704816105 [Google Scholar] [PubMed] [CrossRef]
14. Lindh, T., Malmberg, B. (1999). Age structure effects and growth in the OECD, 1950–1990. Journal of Population Economics, 12(3), 431–449. https://doi.org/10.1007/s001480050107 [Google Scholar] [CrossRef]
15. Meng, L. Y., Li, H. Y., Yang, Y. F. (2011). Characteristics of age structure of modules on Leymus chinensis populations in several succession series in degradation L. chinensis community. Pratacultural Science, 28, 807–812. https://doi.org/10.1093/mp/ssq070 [Google Scholar] [CrossRef]
16. Therrien, K. L. (2010). Age and age structure of an invasive plant, Lythrum salicaria. University of New Hampshire, USA. http://www.caj11.com/e/action/ShowInfo.php?classid=113&id=1423 [Google Scholar]
17. Yang, Y. F., Zheng, H. Y. (1998). Methods of study on age structures of clonal populations in rhizome type grass. Journal of Northeast Normal University, 1, 49–53. [Google Scholar]
18. Pan, Y., Chen, J., Birdsey, R., McCullough, K., He, L. (2011). Age structure and disturbance legacy of North American forests. Biogeosciences, 8(3), 715–732. https://doi.org/10.5194/bg-8-715-2011 [Google Scholar] [CrossRef]
19. Zong, X., Wei, C., Peng, L. U., Dong, H. U. (1999). The demography and age structure of the endangered plant population of Cathaya argyrophylla. Acta Ecologica Sinica, 19, 523–528. https://doi.org/10.3321/j.issn:1000-0933.1999.06.002 [Google Scholar] [CrossRef]
20. Karuppannan, K., Mohamed, K., Abdul-Razak, M., Ahmad-Tahir, N., Mohd-Naim, N. et al. (2020). Sex ratio and age structure patterns of Asian elephants from Peninsular Malaysia revealed by non-invasive surveys. The Journal of Animal & Plant Sciences, 30(6), 1415–1423. https://doi.org/10.36899/JAPS.2020.6.0163 [Google Scholar] [CrossRef]
21. Cao, X., Liu, Y., Liu, Q., Cui, X., Chen, X. et al. (2018). Estimating the age and population structure of encroaching shrubs in arid/semiarid grasslands using high spatial resolution remote sensing imagery. Remote Sensing of Environment, 216, 572–585. https://doi.org/10.1016/j.rse.2018.07.025 [Google Scholar] [CrossRef]
22. Edelfeldt, S., Bengtsson, K., Dahlgren, J. P. (2019). Demographic senescence and effects on population dynamics of a perennial plant. Ecology, 100(8), 1–9. https://doi.org/10.1002/ecy.2742 [Google Scholar] [PubMed] [CrossRef]
23. Yuan, C., Meng, G., Fang, X., Chai, Y., Li, G. X. et al. (2012). Age structure and spatial distribution of the rare and endangered plant Alcimandra cathcartii. Acta Ecologica Sinica, 32, 3866–3872. [Google Scholar]
24. Jiao, D. Z., Yang, Y. F. (2012). Study on age structure of the rhizomes of Phragmites communis population in Zhalong wetlands. Heilongjiang Animal Science and Veterinary Medicine, 21, 74–76. [Google Scholar]
25. Yang, Y., Zheng, H., Li, J. (1998). Comparison of age structures of the tillers in the Leymus chinensis population, a clonal grass species under different ecological conditions. Acta Ecologica Sinica, 28, 567–575. [Google Scholar]
26. Mooney, H. A. (1978). Population biology of plants. Science, 199(4329), 675–676. [Google Scholar]
27. Fidelis, A., Appezzato-da-Glória, B., Pillar, V. D., Pfadenhauer, J. (2014). Does disturbance affect bud bank size and belowground structures diversity in Brazilian subtropical grasslands? Flora-Morphology. Distribution Functional Ecology of Plants, 209(2), 110–116. https://doi.org/10.1016/J.FLORA.2013.12.003 [Google Scholar] [CrossRef]
28. Gai, Z., Li, X., Zhai, J., Chen, X., Li, Z. (2020). Effects of river bank soil physical and chemical factors on populus pruinosa schrenk clonal growth. Applied Ecology and Environmental Research, 18(3), 4791–4806. https://doi.org/10.15666/aeer/1803_47914806 [Google Scholar] [CrossRef]
29. Zhang, D. M., Zhao, W. Z., Luo, W. C. (2019). Effect of the population density on belowground bud bank of a rhizomatous clonal plant Leymus secalinus in Mu Us sandy land. Journal of Plant Research, 132(1), 69–80. https://doi.org/10.1007/s10265-018-01080-9 [Google Scholar] [PubMed] [CrossRef]
30. Bombo, A., Siebert, F., Fidelis, A. (2022). Fire and herbivory shape belowground bud banks in a semi-arid African savanna. African Journal of Range & Forage Science, 39(1), 16–26. https://doi.org/10.2989/10220119.2021.1982004 [Google Scholar] [CrossRef]
31. Benson, E. J., Hartnett, D. C., Mann, K. H. (2004). Belowground bud banks and meristem limitation in tallgrass prairieplant populations. American Journal of Botany, 91(3), 416–421. https://doi.org/10.3732/ajb.91.3.416 [Google Scholar] [PubMed] [CrossRef]
32. Bravo, S., Basualdo, M., Kunst, C., del Corro, F. (2019). Aerial bud bank and structural changes of woody species from Argentine Chaco in response to disturbances. Journal of Environmental Science and Engineering, 8, 58–69. https://doi.org/10.17265/2162-5298/2019.02.002 [Google Scholar] [CrossRef]
33. Semchenko, M., Saar, S., Lepik, A. (2017). Intraspecific genetic diversity modulates plant-soil feedback and nutrient cycling. New Phytologist, 216(1), 90–98. https://doi.org/10.1111/nph.14653 [Google Scholar] [PubMed] [CrossRef]
34. Zhang, L. W., Han, G. X. (2018). A review on the relationships between plant genetic diversity and ecosystem functioning. Chinese Journal of Plant Ecology, 42(10), 977–989. https://doi.org/10.17521/cjpe.2018.0013 [Google Scholar] [CrossRef]
35. Wernberg, T., Coleman, M. A., Bennett, S., Thomsen, M. S., Tuya, F. et al. (2018). Genetic diversity and kelp forest vulnerability to climatic stress. Scientific Reports, 8(1), 1–8. https://doi.org/10.1038/s41598-018-20009-9 [Google Scholar] [PubMed] [CrossRef]
36. Yang, Y., Zhang, B. T. (1998). Quantitative characteristics of vegetative propagation of calamagrostis clonal populations in Songnen Plain of China. Acta Pratacultural Sinica, 7(4), 7–12. [Google Scholar]
37. Yang, Y. F., Liu, G. C., Zhang, B. T. (1995). An analysis of age structure and the strategy for asexual propagation of Aneurolepidium chinense population. Acta Botanica Sinica, 37, 147–153. https://doi.org/10.1007/BF02007173 [Google Scholar] [CrossRef]
38. Forsman, A. (2014). Effects of genotypic and phenotypic variation on establishment are important for conservation, invasion, and infection biology. Proceedings of the National Academy of Sciences of the United States of America, 111(1), 302–307. https://doi.org/10.1073/pnas.1317745111 [Google Scholar] [PubMed] [CrossRef]
39. Lucardi, R. D., Wallace, L. E., Ervin, G. N. (2020). Patterns of genetic diversity in highly invasive species: Cogongrass (Imperata cylindrica) expansion in the invaded range of the Southern United States (US). Plants, 9(4), 1–13. https://doi.org/10.3390/plants9040423 [Google Scholar] [PubMed] [CrossRef]
40. Reed, D. H., Frankham, R. (2003). Correlation between fitness and genetic diversity. Conservation Biology, 17(1), 230–237. https://doi.org/10.1046/j.1523-1739.2003.01236.x [Google Scholar] [CrossRef]
41. Fan, D., Rong, X. J., Ming, Y. Y., Hui, Z. M., Jing, W. (2000). Study on flowering phenomenon and its type of bamboo in Yunnan in past fifteen years. Scientia Silvae Sinicae, 36(6), 57–68. https://doi.org/10.3321/j.issn:1001-7488.2000.06.009 [Google Scholar] [PubMed] [CrossRef]
42. Wang, Y., Ding, X., Wang, X., Wu, M., Gao, S. et al. (2019). Genotypic diversity of a dominant species Leymus chinensis inhibited ecological function of species diversity in the Inner Mongolia Steppe. Acta Ecologica Sinica, 39, 1507–1516. https://doi.org/10.5846/stxb201711021964 [Google Scholar] [CrossRef]
43. Yu, D. W., Lei, Z. Y., Zhao, G. J., Zhang, Y. S., Yu, D. L. et al. (2020). Response of soil physiochemical properties under sand-fixation forest of Pinus sylvestris var. mongolica to stand density. Arid Zone Research, 1, 134–141. https://doi.org/10.13866/j.azr.2020.01.15 [Google Scholar] [CrossRef]
44. Liu, J., Liu, G. S., Qi, D. M., Li, F. F. (2000). Construction of genetic fingerprints of Aneurolepidium chinensis using microsatellite sequences. Acta Botanica Sinica, 42(9), 985–987. [Google Scholar]
45. Xu, S. N., Wang, X. Q., Zhang, X. L., Xia, Y. T., Qiu, X. et al. (2021). Effects of genotypic diversity on age structure in Leymus chinensis population. Acta Botanica Sinica, 41(19), 7848–7857. https://doi.org/10.5846/stxb201910212207 [Google Scholar] [CrossRef]
46. Dong, Y. B. (2007). Study on quantity characteristic of Leymus chinensis population tillering plants in zhalong natural reserve. Journal of Anhui Agricultural Sciences, 35(19), 5732–5733. https://doi.org/10.13989/j.cnki.0517-6611.2007.19.039 [Google Scholar] [CrossRef]
47. Jin, X. M. (2011). The dynamics of age structures on Agropyron michnoi and Leymus chinensis in community with two dominant species populations. African Journal of Biotechnology, 10(76), 17451–17457. https://doi.org/10.5897/AJB11.838 [Google Scholar] [CrossRef]
48. Sun, X. Y., Lu, Z. H., Yu, X. J., Sang, W. G. (2005). Age structure dynamics of populations and its implications for control. Acta Phytoecological Sinica, 29(3), 373–379. https://doi.org/10.17521/cjpe.2005.0049 [Google Scholar] [CrossRef]
49. Yan, L. H., Fei, Y. Y. (2004). Age structures of modules on Leymus chinensis clonal populations in the process of restoration succession after the flooded meadow in the Songnen Plains. China Acta Ecologica Sinica, 24(10), 2171–2177. https://doi.org/10.3321/j.issn:1000-0933.2004.10.012 [Google Scholar] [CrossRef]
50. Bai, W., Sun, X., Wang, Z., Li, L. (2009). Nitrogen addition and rhizome severing modify clonal growth and reproductive modes of Leymus chinensis population. Plant Ecology, 205(1), 13–21. https://doi.org/10.1108/IJCCSM-06-2020-0066 [Google Scholar] [CrossRef]
51. Crawford, K. M., Rudgers, J. A. (2013). Genetic diversity within a dominant plant outweighs plant species diversity in structuring an arthropod community. Ecology, 94(5), 1025–1035. https://doi.org/10.1890/12-1468.1 [Google Scholar] [PubMed] [CrossRef]
52. Shen, J. F., Ren, H. Q., Xin, X. J., Xu, B., Gao, Y. B. (2015). Leymus chinensis genotypic diversity increases the response of populations to disturbance. Acta Ecologica Sinica, 35(23), 7682–7689. https://doi.org/10.5846/stxb201405130979 [Google Scholar] [CrossRef]
53. Yang, X., Shen, J. F., Zhao, N. X., Gao, Y. B. (2017). Phenotypic plasticity and genetic differentiation of quantitative traits in genotypes of Leymus chinensis. Chinese Journal of Plant Ecology, 41(3), 359–368. https://doi.org/10.17521/cjpe.2015.0257 [Google Scholar] [CrossRef]
54. Xu, Y. T., He, M. T., Yang, J. Y., Li, B. Z., Wang, Y. K. et al. (2017). Effect of genotypic diversity on the growth of Koeleria cristata. Chinese Journal of Ecology, 36(9), 2489–2493. https://doi.org/10.13292/j.1000-4890.201709.025 [Google Scholar] [CrossRef]
55. Chang, C. C., Smith, M. D. (2014). Direct and indirect relationships between genetic diversity of a dominant grass, community diversity and above-ground productivity in tallgrass prairie. Journal of Vegetation Science, 25(2), 470–480. https://doi.org/10.1111/jvs.12108 [Google Scholar] [CrossRef]
56. Zhang, J., Li, X., Tian, S., Chen, G. (2015). The spiral elongation rule in clonal population of Leymus chinensis. Acta Ecologica Sinica, 35(8), 2509–2515. https://doi.org/10.5846/stxb201307161901 [Google Scholar] [CrossRef]
57. Yang, Y. F., Zheng, H. Y., Li, J. D. (2001). The effects of grazing on age structure in clonal populations of agropyron michnoi. Acta Phytoecologica Sinica, 25(1), 71–75. [Google Scholar]
58. Wang, Y. S., Gai, X. C. (1995). Foraging growth patterns and resource allocations of clonal Leymus chinensis population in the Songnen steppe. Acta Phytoecologica Sinica, 19(4), 293–301. [Google Scholar]
59. Chu, L. S., Li, H. Y., Yang, Y. F. (2020). Vegetative reproduction characteristics of Leymus chinensis in heterogeneous habitats in Songnen Plain, China. The Journal of Applied Ecology, 31(1), 83–88. https://doi.org/10.13287/j.1001-9332.202001.001 [Google Scholar] [PubMed] [CrossRef]
Cite This Article
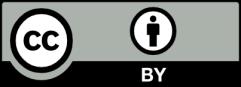
This work is licensed under a Creative Commons Attribution 4.0 International License , which permits unrestricted use, distribution, and reproduction in any medium, provided the original work is properly cited.