Open Access
ARTICLE
Appraisal of Improvement in Physiological and Metabolic Processes by Exogenously Applied Natural and Synthetic Ascorbic Acid in Okra (Abelmoschus esculentus L.) Fruit Subjected to Water Deficit Stress
1
Department of Botany, Government College University, Faisalabad, 38000, Pakistan
2
Department of Botany and Microbiology, Faculty of Science, South Valley University, Qena, 83523, Egypt
3
Department of Botany, University of Lahore, Lahore, Pakistan
* Corresponding Authors: Nudrat Aisha Akram. Email: ; Arafat Abdel Hamed Abdel Latef. Email:
Phyton-International Journal of Experimental Botany 2023, 92(10), 2761-2784. https://doi.org/10.32604/phyton.2023.028801
Received 08 January 2023; Accepted 16 June 2023; Issue published 15 September 2023
Abstract
To counteract the effects of drought stress, scientists have adopted several approaches including the use of different chemicals both inorganic and organic, which is contemplated as a highly efficient and cost-effective shot-gun approach. Ascorbic acid (AsA) is a potential organic substance, which widely occurs in plants, and is considered to be an effective antioxidant to counteract reactive oxygen species (ROS). Thus, a pot experiment was performed to assess the relative mitigating impacts of synthetic AsA and naturally occurring AsA in the form of lemon juice (LJ) and orange juice (OJ) on two cultivars of okra (Abelmoschus esculentus L.) namely Sabz Pari and Bhindi Sanwali under varying water deficit conditions. After 30 days of seed germination, okra seedlings were subjected to different irrigation regimes, i.e., water deficit stress [(65% and 50% F.C.) and control conditions (100% F.C.)]. Different levels of AsA [control (no spray), 14 mg L−1 LJ, 24 mg L−1 OJ and 150 mg L−1 AsA] obtained from different sources were applied as a foliar spray to control and water-stressed plants. Drought stress prominently reduced plant growth and yield attributes of the okra cultivars. Water-deficit conditions (65% and 50% F.C.) substantially decreased the fruit chlorophyll (a, b) pigments and the activity of superoxide dismutase (SOD) enzyme, while an increase was observed in the contents of fruit’s hydrogen peroxide (H2O2), malondialdehyde (MDA), total phenolics, total soluble sugars, AsA, and total soluble proteins. Drought stress also increased the activities of antioxidant enzymes like peroxidase (POD) and catalase (CAT). However, plant growth and yield attributes, fruit chlorophyll pigments, total phenolics, total soluble sugars, total free amino acids, total soluble proteins, AsA, GB, H2O2, and the activities of antioxidant enzymes (POD and CAT) were increased by the AsA exogenous treatment in both okra cultivars under water deficit and control conditions. Overall, LJ and OJ were more effective than the synthetic AsA in upregulating the physiological and metabolic processes of okra plants. So, cost-effective as well as multi-nutrient natural sources of AsA could be suggested for alleviating the harmful effects of water deficit stress on plants.Keywords
Plants naturally face several environmental stresses and such constraints cause a reduction in plant growth and development, which ultimately results in yield reduction [1,2]. Water deficiency is considered as the most dreadful environmental factor, that causes a reduction in crop yield more effectively as compared to that by other environmental constraints [3,4]. According to Manivannan et al. [5], agricultural drought is the lack of sufficient quantity of irrigation water required for most crops to complete their life span. It is widely reported that the morphological and physio-biochemical characteristics of plants are adversely affected by water shortage during different growth stages [6,7]. Common visible morphological effects of drought observed in plants include a reduction in the number of leaves, plant height, decreased seed number, width and diameter, and fruit and seed weight [8,9]. Furthermore, plants upregulate drought tolerance mechanism against abiotic stress by adopting different physio-biochemical strategies including more uptake of water by a deeper root system, increased stomatal conductance, enhanced accumulation of osmoprotectants, stability of plasma membrane, scavenging of ROS and production of stress-related proteins [10,11].
Vitamin C or AsA acts as an antioxidant, and it plays an important role in the detoxification of ROS [12–15]. Previous studies have reported that AsA scavenge the free oxygen species and protect plants from oxidative damage [15,16]. The use of plant-growth biostimulants has increased in the last few years since it can improve crop quality without causing any damage to the environment [17]. It is believed that the direct use of plant extracts enriched with different biostimulants/bioregulators is more effective and environmentally safe as compared to synthetic chemicals. These biostimulants can reduce environmental pollution by eliminating or reducing the need for mineral fertilizers [18–20]. Moreover, AsA plays a role as an important signaling modulator of different physiological processes occurring in plant’s cell [21,22]. AsA causes detoxification of hydrogen peroxide under drought-stress conditions [23,24]. Ascorbic acid acts as an enzyme co-factor and regulates the signaling mechanism mediated by phytohormones as well as the physiological processes of plants [15,25]. The stress tolerance mechanism of the plant can be enhanced by increasing the level of AsA, so the harmful effects of drought can be eliminated [26–28].
Moreover, the exogenous application of AsA improves plant growth and development efficiently by maintaining different processes like phytohormones’ signaling, ion transport mechanism, cell expansion, and oxidative defense mechanism under both stress and non-stress environments [15,22,29–32]. In addition, exogenously applied AsA eliminates the negative role of water deficit stress on plants [33,34]. Previous research performed by different investigators on different crops, i.e., wheat [35], corn [36], soybean [37], rapeseed [38], grapes [39], canola [16] and cauliflower [7] showed that exogenously applied AsA had beneficial effect on morphological and physio-biochemical processes under drought stress, while the effects of AsA on the biochemistry as well as the quality of fruits and vegetables have been rarely investigated.
Okra (Abelmoschus esculentus L.) is one of the potential heat-tolerant vegetable crops and is widely grown for tender fruits and young leaves during hot seasons in worldwide tropical areas [40–44]. Calisir et al. [45] proposed that okra crop can grow well in both tropical and temperate zones and is very easy to cultivate. The fruit of okra is rich in nutritional contents like dietary fiber, amino acids, riboflavin, folic acid, thiamin, vitamins, and minerals [42,46]. Many researchers prefer to adopt an easy and cost-effective approach to mitigate the harmful effects of stressful environmental conditions including water deficiency. Many studies in the literature revealed that some potential non-enzymatic antioxidants applied externally proved better for promoting plant growth, and AsA is one of them [15,16]. The presence of AsA was mainly noticed in plant cytosol, apoplast and in actively growing parts [15,47,48]. The present study was conducted based on a potential hypothesis that natural AsA taken from the juices of fresh lemon and orange is one of the effective strategies to enhance growth and key physio-biochemical processes of okra plants and fruits as compared to that by the synthetic AsA under water deficit environment. Thus, the experiment was conducted to evaluate the influence of externally applied natural (fresh lemon and orange juices) vitamin C (AsA) in comparison with synthetic AsA (purchased from the market) on plant growth, yield production and physio-biochemical attributes of okra fruits under drought-stress and control (non-stress) conditions. Moreover, the best source of AsA can be suggested based on improvement in growth and yield production for the crops subjected to water-deficit stress during their life cycle.
Influence of foliar applied synthetic AsA and natural sources of AsA such as phytoextracts of two plants [lemon (Citrus limon L.) and sweet orange (Citrus sinensis L.)] on okra (Abelmoschus esculentus L.) fruits were examined under water deficit conditions. For this purpose, an experiment was conducted in the village Mahar Sharif near Chishtian, Punjab Pakistan in 2019. During experimentation, the average day and night temperature 34.4°C and 19.8°C, relative humidity 58%, rainfall 22.4 mm, and sunshine duration 11.4 h, were recorded. The seeds of Cv. Sabz Pari were collected from the Vegetable Section of Ayub Agricultural Research Institute (AARI), Faisalabad, Pakistan, while those of Cv. Bhindi Sanwali were purchased from the local market of Faisalabad, Pakistan. Each pot was filled with 7.0 kg soil having the following composition: 64% sand, 28.4% silt and 7.6% clay, pH 7.9, saturation percentage 29% and ECe 1.87 dS m−1. There were a total of 96 experimental units (pots) including cultivars, drought stress levels, AsA levels and four replicates of each treatment (2 × 3 × 4 × 4 = 96). Each pot was considered as a replicate. In each pot (replicate), six seeds were planted, and thinning was done after 15 days of seed germination to retain four plantlets in every pot or replicate. After thirty days, the seedlings were subjected to 100% (control), 65% and 50% field capacities (drought stress levels). Based on the soil saturation percentage and field capacity, these drought stress levels were maintained; the drought stress levels were attained after a period of 15 days, and maintained for the next thirty days. Drought stress levels were checked daily and added water as per set value. After thirty days of maintenance of drought stress levels, 150 mg L−1 AsA, 14 mg L−1 LJ and 24 mg L−1 OJ besides the control (no spray) were used as foliage spray. For the LJ and OJ extraction, fresh lemons and sweet oranges were collected from the market; the peel was removed from both fruits. The juice was stored after extraction from the pulp by using an electric juicer. Before use, the content of AsA in 50% LJ (14 mg L−1) and 50% OJ (24 mg L−1) were determined [49]. Different solutions were prepared, and 0.1% Tween-20 was also added to each solution. After 30 days of foliar-applied treatments, a plant of uniform size from each pot was collected and air-dried after washing with distilled water, and their dry weights determined. Moreover, the following physio-biochemical traits were recorded after fruit collection.
A fresh fruit sample (0.25 g) was ground in 5 mL of 80% acetone solution; it was homogenized and kept overnight at 4°C following the procedure of Arnon [50]. The absorbance was read at 663 and 645 nm and all the concentrations of all pigments were assessed.
According to Bates et al. [51], a fresh fruit sample (0.25 g) was ground in 5 mL of 3% sulfosalicylic acid. One mL of the extracted material was filtered and mixed with 1.0 mL of acidic ninhydrin and 1.0 mL of glacial acetic acid. The mixture was heated for 1.0 h at 100°C in a water bath. Then 2 mL of toluene were added to the cooled mixture. The absorbance was noted at 520 nm with the help of a spectrophotometer.
Following Mukherjee et al. [49], the fresh fruit sample (0.25 g) was extracted in 5 mL solution of 6% trichloroacetic acid (TCA). Two mL of the extracted solution were filtered and mixed with 1.0 mL of 2, 4 dinitrophenylhydrazine (2%). Then, one drop of 10% thiourea was added and the solution was heated at 100°C for 15 min on a water bath. Each sample mixture was cooled at room temperature, and mixed with 2.5 mL of 80% H2SO4, and the optical density of all samples were recorded at 530 nm using a spectrophotometer.
2.4 Hydrogen Peroxide Contents
Hydrogen peroxide contents were estimated following the procedure of Velikova et al. [52]. Fresh fruit sample (0.25 g) was triturated in 5 mL of 0.1% ice-cold TCA. Then, an aliquot (500 uL) of the supernatant was separated by centrifugation. Then, 1.0 mL of 1 M potassium iodide (KI) and 500 uL of 10 mM potassium phosphate buffer pH (7.0) were added and the optical density of each sample was noted at 390 nm.
2.5 Malondialdehyde (MDA) Contents
Heath et al. [53] protocol was followed to determine the MDA contents. Fresh fruit (0.25 g) was homogenized in 5 mL of TCA (5%). The supernatant (500 μL) was separated and 2 mL of 0.5% thiobarbituric acid were added to it. The solution was heated in a water bath for 50 min at 95°C and the absorbance of the cooled solution was noted at 600 and 532 nm.
A fruit sample (0.25 g) was extracted in 5 mL of 80% acetone. The supernatant (100 uL) was separated in a test tube and to it distilled water (2 mL) was added. Then 1 mL of the Folin-Ciocalteu phenol reagent was added to the mixture, and all test tubes were agitated efficiently. Then, 5 mL of 20% sodium carbonate (Na2CO3) solution were added to each of the test tubes followed by the addition of distilled water to make the final volume up to 10 mL. The absorbance of each treated sample was read at 750 nm following the protocol of Julkenen-Titto [54].
The Grieve et al. [55] protocol was followed to determine the GB content. A fruit sample (0.25 g) was extracted in 5 mL distilled water. Then 1.0 mL of the filtered sample was mixed with 1.0 mL of 2N H2SO4 and 200 μL of potassium tri-iodide (KI3). In the cooled mixtures, 6 mL of 1, 2 dichloroethane and 2.8 mL of distilled water were added. Resultantly, two layers appeared in the reaction mixture. The upper layer was discarded, and the lower layer was used to record absorbance at 365 nm.
2.8 Activities of Antioxidant Enzymes
For measuring antioxidant enzymes activities, 0.25 g fresh fruit sample was crushed and homogenized in cooled potassium phosphate buffer (50 mM; pH, 7.8) using a chilled pestle and mortar. Then, the extracted sample was centrifuged at 12,000 × g at 4°C for 20 min. The supernatant was separated and stored at −20°C to measure the following enzymatic activities and total soluble proteins.
The Van Rossum et al. [56] procedure was used, and the extracted fruit sample (50 μL) was added in a plastic cuvette. The reaction mixture was made by taking 100 μL L-methionine, 100 μL triton-X, 250 μL (50 mM) potassium phosphate buffer (pH 7.8), 50 μL nitroblue tetrazolium (NBT), 50 μL riboflavin and 400 μL distilled water. Then, the quartz cuvettes containing reaction mixture were kept under light for 5 min before recording the absorbance at 560 nm.
Fresh fruit extract (100 μL) was taken in a quartz cuvette and 1.8 mL of 50 mM potassium phosphate buffer (7.8 pH) was added to it. In addition, 100 μL (40 mM) H2O2 and 100 μL (20 mM) guaiacol were also added. The absorbance was noted at 470 nm for 180 s following the protocol documented by Chance et al. [57].
The activity of CAT enzyme was estimated following the procedure outlined by Chance et al. [57]. For this, the reaction mixture was prepared in a quartz cuvette by adding 100 uL of the fruit extract, 1.0 mL of 5.9 mM H2O2 and 1.9 mL of potassium phosphate buffer (50 mM, pH 7.8). The optical density was noted after every 20 s for 2 min at 240 nm.
Total soluble proteins were estimated by mixing 100 μL fruit extract with an aliquot (2 mL) of the Bradford reagent in a test tube following the method of Bradford [58]. After keeping the mixture at room temperature for 20 min the optical density was recorded at 595 nm for the determination of total soluble proteins.
Total free amino acids were estimated following Hamilton et al. [59]. The fruit sample (1.0 mL) was taken in a test tube. The same volume of 10% pyridine and acidic ninhydrin were also added to the test tubes. The reaction mixture was boiled for 30 min at 100°C. Then, the mixture was cooled and distilled water added to make the final volume to 7.5 mL. Then, the absorbance was noted at 570 nm using a spectrophotometer.
The Yemm et al. [60] protocol was followed and 0.25 g of the fresh fruit sample was extracted in 5 mL (80%) ethanol. A sample extract (100 μL) was taken in a test tube and 3.0 mL of the anthrone reagent (prepared in 72% H2SO4) were added to it. The mixture was boiled for 10 min at 100°C. The reaction mixture was maintained at room temperature for 20 min and the absorbance of all treated samples was noted at 625 nm.
A completely randomized experiment with a three-factor factorial arrangement (cultivars, drought stress and different AsA levels) was performed in pots with four replicates. The data of all the parameters were subjected to a three-way analysis of variance (ANOVA) (Table 1) and a one-way ANOVA (Tables 2a–6b). The significant differences among the mean values and the significant effect of AsA on each okra cultivar were determined by the least significant difference test at the probability level of 0.05% and different letters were presented on each bar of all figures.
Shoot and root dry weights declined constantly as water deficit levels increased from 65% to 50% F.C. (Table 1). However, foliar-applied 150 mg L−1 ascorbic acid and the phytoextracts (14 and 24 mg L−1) showed significant promoting effects on the shoot and root dry weights of both okra cultivars. For both cultivars, Sabz Pari and Bhindi Sanwali, 14 mg L−1 natural AsA was the most effective in enhancing the shoot and root dry weights, specifically at 50% F.C. (Tables 2a–3b). However, at 100% F.C. (control), root dry weight was promoted more by 24 mg L−1 OJ application in okra Cv. Sabz Pari (Table 1; Fig. 1). While both okra cultivars showed consistent responses to water stress and exogenous application of AsA in terms of these growth attributes which significantly deacreased in both okra cultivars at 65% and 50% F.C. (Tables 3a and 3b).
Figure 1: Shoot and root dry weights, fruit fresh and dry weights and number of fruits per plant of two cultivars of okra (Abelmoschus esculentus L.) subjected to varying water-deficit conditions and foliarly treated with varying sources of AsA (Mean ± S.E.); AsA, Ascorbic acid; LJ, Lemon juice; OJ, Orange juice. Different letters (a–k) showing significance of difference among different treatments
Different water deficit levels considerably caused a reduction in fruit fresh and dry weights as well as the number of fruits per plant (Fig. 1). While fruit fresh and dry weights were recorded to be improved (p ≤ 0.001) by foliar applied AsA, LJ and OJ under all water deficit regimes. However, the number of fruits per plant showed no significant response to exogenously applied AsA (Table 1). The maximum fruit fresh and dry weights were observed under control conditions by foliar applied LJ in Cv. Sabz Pari and by 150 mg L−1 AsA in Cv. Bhindi Sanwali (Tables 4a–5c).
Water stress regimes (65% and 50% F.C.) adversely (p ≤ 0.001) affected chlorophyll a, b and total chlorophyll contents in both okra cultivars (Tables 1–3b). Moreover, the ratio of chlorophyll a/b was increased under water stress, particularly at 65% F.C. in both okra cultivars (Fig. 2). Exogenously applied varying sources of AsA significantly improved (p ≤ 0.001) chlorophyll a, b and total chlorophyll contents, while the ratio of chlorophyll a/b showed no significant change under both stress and control conditions. No significant difference was observed among all AsA sources under varying water regimes. Similarly, the difference between both okra cultivars was non-significant under water stress and AsA treatments.
Figure 2: Chlorophyll a, b, total chlorophyll and chlorophyll a/b ratio, proline and glycinebetaine (GB) contents of fruits of two cultivars of okra (Abelmoschus esculentus L.) subjected to varying water-deficit conditions and foliarly treated with varying sources of AsA (Mean ± S.E.). Different letters (a–k) showing significance of difference among different treatments
Fruit proline contents remained unaffected, while water deficit conditions significantly increased the GB contents in both okra cultivars. Exogenously applied varying treatments of AsA, LJ and OJ markedly (p ≤ 0.001) increased proline concentrations of okra fruits, while GB content remained unchanged at different water stress levels (Table 1). Furthermore, a considerable difference was noticed in both okra cultivars for fruit proline and GB contents. The Cv. Sabz Pari was better than Cv. Bhindi Sanwali in these parameters under varying drought stress levels (Table 6a).
Accumulation of H2O2 augmented substantially (p ≤ 0.001) in fruits of both okra cultivars under varying water-deficit levels. Moreover, a significant decrease in H2O2 contents was observed in both okra cultivars by different AsA sources at 50% field capacity (Table 1). However, of both okra cultivars, Cv. Bhindi Sanwali was higher in H2O2 accumulation than Cv. Sabz Pari at 50% F.C. (Fig. 3).
Figure 3: Hydrogen peroxide (H2O2), malondialdehyde (MDA), total phenolics, ascorbic acid (AsA), total soluble sugars and total free amino acids of fruits of two cultivars of okra (Abelmoschus esculentus L.) subjected to varying water-deficit conditions and foliarly treated with varying sources of AsA (Mean ± S.E.). Different letters (a–k) showing significance of difference among different treatments
Fruit MDA content increased substantially under drought stress in both cultivars of okra (Table 1). While different sources of AsA significantly (p ≤ 0.05) suppressed the MDA contents in the water-stressed plants of both okra cultivars. The MDA contents decreased more prominently in Cv. Sabz Pari than that inokra Cv. Bhindi Sanwali under varying water stress regimes (Fig. 3).
The contents of ascorbic acid and total phenolics considerably (p ≤ 0.001) increased in fruits of both okra cultivars due to drought stress imposition. However, exogenously applied AsA, LJ and OJ considerably improved the concentrations of ascorbic acid and total phenolics in okra cultivars under both water deficit stress and non-stress conditions (Fig. 3). Of both okra cultivars, Cv. Sabz Pari showed more increase in fruit total phenolics and AsA contents at varying water deficit regimes.
Total soluble sugars increased prominently (p ≤ 0.01) under water stress as well as on application of varying sources of AsA (Fig. 3; Table 6b). Of all sources, 14 mg L−1 LJ and 24 mg L−1 OJ notably raised the total soluble sugars, particularly at 50% F.C. Of both okra cultivars, Cv. Sabz Pari accumulated a maximum increase in the levels of total soluble sugars under water-limited environment particularly at 50% F.C.
Water deficit regimes considerably (p ≤ 0.001) increased total free amino acids in fruits of both okra cultivars (Fig. 3). Foliar application of different ascorbic acid sources improved total free amino acids in fruits of both okra cultivars and 24 mg L−1 OJ was the most effective for increasing this attribute under 50% F.C. Moreover, okra Cv. Sabz Pari was better in total free amino acids accumulation than that by Cv. Bhindi Sanwali at 50% F.C.
Total soluble protein content was considerably increased (p ≤ 0.01) in okra fruits under drought stress in both cultivars (Table 1; Fig. 4). Different sources of ascorbic acid also improved total soluble proteins more prominently with the foliar-applied 14 mg L−1 LJ and 24 mg L−1 OJ at varying water-deficit levels. However, both okra cultivars were similar in response to different AsA and drought stress levels.
Figure 4: Total soluble proteins and activities of catalase (CAT), peroxidase (POD) and superoxide dismutase (SOD) enzymes of fruits of two cultivars of okra (Abelmoschus esculentus L.) subjected to varying water-deficit conditions and foliarly treated with varying sources of AsA (Mean ± S.E.). Different letters (a–j) showing significance of difference among different treatments
The activity of SOD enzyme was decreased (p ≤ 0.001) under drought stress conditions. However, exogenously-applied varying sources of AsA upregulated the activity of SOD enzyme only in okra Cv. Sabz Pari, while it decreased in the case of Cv. Bhindi Sanwali. Drought stress and foliar-applied AsA, LJ and OJ markedly upregulated the activities of POD (p ≤ 0.001) and CAT (p ≤ 0.05) enzymes in the fruits of both okra cultivars (Table 1; Fig. 4). Moreover, a maximum improvement in the enzymatic antioxidant activities of fruit POD and CAT enzymes was observed in Cv. Sabz Pari at 65% F.C. and SOD activity at 100% F.C. in both cultivars of okra. Of all AsA sources, foliar applied 24 mg L−1 OJ showed a maximal improvement in the enzymatic activities of fruit POD and CAT of both okra cultivars under drought stress conditions (Fig. 4). Overall, Cv. Sabz Pari was better in POD activity and Cv. Bhindi Sanwali in the activity of SOD under water-deficit conditions.
The quality and quantity of agricultural products can be enhanced by using economical approaches including the use of biostimulants, extracted from plants or their parts. Such natural chemical substances are not only profitable and effective, but are also safe environmentally and biologically [61,62]. The present experiment compared the influence of different sources of ascorbic acid used exogenously including synthetic AsA (150 mg L−1) and phytoextracts [LJ (14 mg L−1) and OJ (24 mg L−1)] naturally enriched with AsA on modulation of growth, yield and biochemical metabolites of two okra cultivars under drought stress and non-stress environments. The current study showed that the shoot and root dry weights as well as the fruit yield of water stress-induced okra plants decreased consistently. Water deficiency-induced adverse effects are believed to be usually due to the inhibition of enzyme activities, stomatal closure, impairment in photosynthesis, imbalance in nutritional and hormonal metabolism, membrane leakage as well as excessive oxidative stress [11,63–65]. We observed that both shoot and root dry weights and fruit yield of both okra cultivars were enhanced by exogenously applied AsA sources, and of all sources, 14 mg L−1 LJ application greatly enhanced the shoot and root dry weights, particularly at 50% field capacity. Earlier studies have shown that AsA can improve plant growth by promoting photosynthetic pigments, photosynthetic rate, water balance and oxidative defense potential under stress conditions [66,67]. It has also been reported that exogenously applied AsA protects proteins and lipids against oxidative damage [16,67], by performing the role as a substrate for ascorbate peroxidase which scavenges H2O2 under different stresses including water deficit conditions. Previously, Khan et al. [66] revealed the influence of foliar-applied AsA (50 and 100 mg L−1) on wheat plants growing in hydroponic culture under salt stress. They reported that ascorbic acid applied as a foliar spray increased chlorophyll a content. However, in another study with okra plants, AsA application increased proline contents and decreased membrane leakage and lipid peroxidation under water deficit conditions, resulting in improved plant growth [33].
Under stress conditions, decline in chlorophyll contents and rate of photosynthesis occurs due to the inactivation of enzymes involved in the synthesis of chlorophyll pigments, resulting a reduction in crop yield and quality by altering their normal metabolic activities [41,68–70]. In the current study, increasing drought stress from 65% to 50% F.C. significantly decreased the chlorophyll a, b and total chlorophyll contents. Exogenously applied various sources of AsA improved the photosynthetic pigments of okra fruits (Table 1; Fig. 2). Foliar-applied AsA has been reported to improve chlorophyll pigments in different plants such as cucumber [71], and cauliflower [7]. Another study performed by Aziz et al. [28] showed that foliar-applied AsA affected positively chlorophyll a, b and total chlorophyll contents in quinoa plants. However, they suggested that both synthetic AsA (150 mg L−1) and 25% OJ were equally effective in improving chlorophyll pigments under water-limited conditions.
Osmoprotection is one of the potential strategies adopted by plants under environmental stresses [72]. However, a variety of organic substances can play an active role in this vital process. For example, proline and GB widely occur in most plants and their contribution towards osmoprotection is significant [11,39,73]. Our results indicated that fruit proline contents remained unaffected in both okra cultivars, whereas those of GB increased prominently under water deficit conditions. However, fruit proline concentration increased significantly by foliar-applied AsA and LJ. These findings are not in agreement with those already reported by Singh et al. [26], as they observed no considerable AsA-induced changes in the accumulation of proline contents in wheat plants by application of AsA, while GB contents increased under stress conditions. However, in several studies with different plants, an increase in proline contents was observed by exogenously applied AsA either synthetic or natural, e.g., rapeseed [38], soybean [37], and cucumber [71], etc. Both GB and proline are believed to accelerate the oxidative defense system in stressed plants [74]. So, it can be inferred from the results of the present study that exogenously applied AsA sources enhanced GB and proline accumulation, thereby resulting in enhanced osmotic adjustment.
Hydrogen peroxide and MDA contents were raised in the fruits of both okra cultivars under water deficit conditions. These results are in accordance with what has already been observed in quinoa plants by Aziz et al. [28], and in Amaranthus plants by Sarker et al. [75], under varying water-deficit conditions. Accumulation of H2O2 at a low level in plants under stress may act as a signaling molecule that can trigger tolerance to various biotic and abiotic stresses, while in higher concentrations, it is harmful to cellular metabolites [76–78]. Moreover, in the present study, foliar-applied AsA 14 mg L−1 LJ or 24 mg L−1 OJ significantly suppressed the H2O2 and MDA contents in both okra cultivars. Similar results were already observed in Gossypium herbaceum by Deeba et al. [63] and in cucumber by Nie et al. [79].
Drought stress significantly decreased a variety of metabolites such as total phenolics, total soluble sugars, AsA, free amino acids and total soluble proteins determined in okra fruits. Such results have already been observed in Scutellaria baicalensis [80], Vitis vinifera [78], and Adonis amurensis and A. pseudoamurensis [81]. Moreover, exogenous applications of AsA obtained from different sources such as 14 mg L−1 LJ and 24 mg L−1 OJ considerably improved all the above-mentioned metabolites in the fruits of both okra cultivars. These findings are in agreement with those of Khan et al. [66], and Malik et al. [82], wherein it was reported that exogenously applied AsA can mitigate the effects of drought stress. They also suggested that AsA acts as a growth regulator and it can improve plants’ resistance mechanism against different environmental stresses. However, compared with synthetic AsA, exogenous applications of LJ and OJ showed better results in terms of the accumulation of these metabolites. Moreover, it is widely observed in different plants that the stress tolerance mechanism of plants could be improved by increasing endogenous levels of amino acids and proteins, e.g., canola [16], grapes [39] and wheat [83].
Plants under stress conditions protect their cells/tissues by maintaining oxidative defense system by counteracting oxidative stress mechanisms [84,85]. It is widely known that both enzymatic and non-enzymatic antioxidants could enhance the oxidative defensive mechanism of plants [15]. The present experiment also suggested that drought stress considerably enhanced the activities of antioxidant enzymes (POD and CAT) in the fruits of both okra cultivars. It is analogous to what has earlier been recorded in cotton wherein water deficit stress promoted the activities of enzymes including ascorbate peroxidase (APX), SOD, POD and CAT [86]. The current study has shown that under different water deficit regimes, foliar applied varying sources of AsA further improved the activities of antioxidant enzymes (POD and CAT) in the fruits of both okra cultivars, while that of SOD activity only in Cv. Sabz Pari. Overall, 24 mg L−1 OJ was the most effective in upregulating the activities of CAT, POD and SOD enzymes under drought stress conditions. These results are in agreement with those of Ejaz et al. [87] and Halimeh et al. [88]. They reported that exogenously applied AsA boosted the activities of POD and CAT enzymes under water-limited conditions. Moreover, similar results were observed in wheat by Malik et al. [83], and in quinoa by Aziz et al. [28]. They suggested that high level of endogenous AsA may be involved in improving the activities of POD and SOD enzymes in water-deficit plants by foliar-applied AsA.
In conclusion, different water-deficit regimes decreased the plant growth, fruit yield, and the activity of SOD enzyme as well as photosynthetic pigments, while an increase in fruit MDA, H2O2, total phenolics, total soluble sugars, AsA, free amino acids and total soluble proteins and the activities of POD and CAT enzymes in okra fruits was noticed. However, exogenously applied different AsA sources improved plant growth, fruit yield, and key metabolites in okra fruits under both stress and non-stress environments. Overall, the foliar applied LJ and OJ were more efficient than the synthetic AsA in enhancing water deficit tolerance in okra fruits in terms of growth, yield production and key metabolites. So, the findings of the current study suggested that AsA involved in drought tolerance of plants and environment-friendly cheap and multi-nutrient sources could be more beneficial for improving stress tolerance mechanisms involved in growth and yield production.
Acknowledgement: The authors highly acknowledge the support of Department of Botany, Government College University, Faisalabad, Pakistan for providing the facilities to complete different biochemical analyses.
Funding Statement: The authors received no specific funding for this study.
Author Contributions: Nudrat Aisha Akram; study conception and design: Muhammad Younis; data collection, analysis and interpretation of results: Arafat Abdel Hamed Abdel Latef and Muhammad Ashraf; draft manuscript preparation. All authors reviewed the results and approved the final version of the manuscript.
Availability of Data and Materials: The data can be provided on demand.
Ethics Approval: Not applicable.
Conflicts of Interest: The authors declare that they have no conflicts of interest to report regarding the present study.
References
1. Seki, M., Kameiy, A., Yamaguchi-Shinozaki, K., Shinozaki, K. (2003). Molecular responses to drought, salinity and frost: Common and different paths for plant protection. Current Opinion in Biotechnology, 14(2), 194–199. [Google Scholar] [PubMed]
2. Sadiq, M., Akram, N. A., Ashraf, M. (2018). Impact of exogenously applied tocopherol on some key physio-biochemical and yield attributes in mungbean [(Vigna radiata L.) Wilczek] under limited irrigation regimes. Acta Physiologiae Plantarum, 40, 131. [Google Scholar]
3. Nalina, M., Saroja, S., Rajkumar, R., Radhakrishnan, B., Chandrashekara, K. N. (2018). Variations in quality constituents of green tea leaves in response to drought stress under south Indian condition. Scientia Horticulturae, 233, 359–369. [Google Scholar]
4. Ghafar, M. A., Akram, N. A., Saleem, M. H., Wang, J., Wijaya, L. et al. (2021). Ecotypic morphological and physio-biochemical responses of two differentially adapted forage grasses, Cenchrus ciliaris L. and Cyperus arenarius Retz. to drought stress. Sustainability, 13(14), 8069. [Google Scholar]
5. Manivannan, P., Jaleel, C. A., Somasundaram, R., Panneerselvam, R. (2008). Osmoregulation and antioxidant metabolism in drought-stressed Helianthus annuus under triadimefon drenching. Comptes Rendus Biologies, 331(6), 418–425. [Google Scholar] [PubMed]
6. Rao, N. S., Laxman, R. H., Shivashankara, K. S. (2016). Physiological and morphological responses of horticultural crops to abiotic stresses. In: Abiotic stress physiology of horticultural crops, pp. 3–17. India: Springer. [Google Scholar]
7. Latif, M., Akram, N. A., Ashraf, M. (2016). Regulation of some biochemical attributes in drought-stressed cauliflower (Brassica oleracea L.) by seed pre-treatment with ascorbic acid. The Journal of Horticultural Science and Biotechnology, 91(2), 129–137. [Google Scholar]
8. Shahidan, N., Othman, R., Jaswir, I., Hasyun-Hashim, Y. (2018). Effect of abiotic stress under light and dark conditions on carotenoid content in pumpkin (Cucurbita moshata) calluses. Journal of Fundamental and Applied Sciences, 9(2S), 861. [Google Scholar]
9. Langeroodi, A. R. S., Campiglia, E., Mancinelli, R., Radicetti, E. (2018). Can biochar improve pumpkin productivity and its physiological characteristics under reduced irrigation regimes? Scientia Horticulturae, 247, 195–204. [Google Scholar]
10. Aslam, M., Zamir, M. S. I., Afzal, I., Yaseen, M., Mubeen, M. et al. (2013). Drought stress, its effect on maize production and development of drought tolerance through potassium application. Cercetari Agronomice in Moldova, 46(2), 99–114. [Google Scholar]
11. Shafiq, S., Akram, N. A., Ashraf, M., Garcia-caparros, P., Ali, O. M. et al. (2021). Influence of glycine betaine (natural and synthetic) on growth, metabolism and yield production of drought-stressed maize (Zea mays L.) plants. Plants, 10(11), 2540. [Google Scholar] [PubMed]
12. Smirnoff, N. (2000). Ascorbic acid: Metabolism and functions of a multifaceted molecule. Current Opinion in Biotechnology, 3, 229–235. [Google Scholar]
13. Foyer, C. H., Noctor, G. (2011). Ascorbate and glutathione: The heart of the redox hub. Journal of Plant Physiology, 155(1), 2–18. [Google Scholar]
14. Qian, H. F., Peng, X. F., Han, X., Ren, J., Zhan, K. Y. et al. (2014). The stress factor, exogenous ascorbic acid, affects plant growth and the antioxidant system in Arabidopsis thaliana. Russian Journal of Plant Physiology, 61, 467–475. [Google Scholar]
15. Akram, N. A., Shafiq, F., Ashraf, M. (2017). Ascorbic acid-a potential oxidant scavenger and its role in plant development and abiotic stress tolerance. Frontiers in Plant Science, 8, 613. [Google Scholar] [PubMed]
16. Shafiq, S., Akram, N. A., Ashraf, M., Arshad, A. (2014). Synergistic effects of drought and ascorbic acid on growth, mineral nutrients and oxidative defense system in canola (Brassica napus L.) plants. Acta Physiologiae Plantarum, 36, 1539–1553. [Google Scholar]
17. Ertani, A., Pizzeghello, D., Francioso, O., Tinti, A., Nardi, S. (2016). Biological activity of vegetal extracts containing phenols on plant metabolism. Molecules, 21(2), 205. [Google Scholar] [PubMed]
18. Shahid, M. A., Balal, R. M., Perveza, M. A., Abbas, T., Aqueel, M. A. et al. (2015). Foliar spray of phyto-extracts supplemented with silicon: An efficacious strategy to alleviate the salinity-induced deleterious effects in pea (Pisum sativum L.). Turkish Journal of Botany, 39(3), 408–419. [Google Scholar]
19. Szparaga, A., Kocira, S. (2018). Generalized logistic functions in modelling emergence of Brassica napus L. PLoS One, 13(8), e0201980. [Google Scholar] [PubMed]
20. Godlewska, K., Michalak, I., Pacyga, P., Basladynska, S., Chognacka, K. (2019). Potential applications of cyanobacteria: Spirulina platensis filtrates and homogenates in agriculture. World Journal of Microbiology and Biotechnology, 35(6), 80. [Google Scholar] [PubMed]
21. Lopez-Munguia, A., Hernandez-Romero, Y., Pedraza-Chaverri, J., Miranda-Molina, A., Regla, I. et al. (2011). Phenylpropanoid glycoside analogues: Enzymatic synthesis, antioxidant activity and theoretical study of their free radical scavenger mechanism. PLoS One, 6(6), 20115. [Google Scholar]
22. Podgórska, A., Burian, M., Szal, B. (2017). Extra-cellular but extra-ordinarily important for cells: Apoplastic reactive oxygen species metabolism. Frontiers in Plant Science, 8, 1353. [Google Scholar]
23. Hemavathi, C. P., Upadhyaya, N., Akula, H. S., Kim, J. H., Jeon, M. H. et al. (2011). Biochemical analysis of enhanced tolerance in transgenic potato plants overexpressing D-galacturonic acid reductase gene in response to various abiotic stresses. Molecular Breeding, 28, 105–115. [Google Scholar]
24. Mostofa, M. G., Rahman, A., Ansary, M. M. U., Watanabe, A., Fujita, M. et al. (2015). Hydrogen sulfide modulates cadmium-induced physiological and biochemical responses to alleviate cadmium toxicity in rice. Scientific Reports, 5, 14078. [Google Scholar] [PubMed]
25. Zhang, L. X., Zhai, Y., Li, Y., Zhao, Y., Lv, L. et al. (2012). Effects of nitrogen forms and drought stress on growth, photosynthesis, and some physic-chemical properties of stem juice of two maize (Zea mays L.) cultivars at elongation stage. Pakistan Journal of Botany, 44(4), 1405–1412. [Google Scholar]
26. Singh, N., Bhardwaj, R. D. (2016). Ascorbic acid alleviates water deficit induced growth inhibition in wheat seedlings by modulating levels of endogenous antioxidants. Biologia, 71, 402–413. [Google Scholar]
27. Seminario, A., Song, L., Zulet, A., Nguyen, H. T., González, E. M. et al. (2017). Drought stress causes a reduction in the biosynthesis of ascorbic acid in soybean plants. Frontiers in Plant Science, 8, 1042. [Google Scholar] [PubMed]
28. Aziz, A., Akram, N. A., Ashraf, M. (2018). Influence of natural and synthetic vitamin C (ascorbic acid) on primary and secondary metabolites and associated metabolism in quinoa (Chenopodium quinoa Willd.) plants under water deficit regimes. Plant Physiology Biochemistry, 123, 192–203. [Google Scholar] [PubMed]
29. Wang, R., Liu, S., Zhou, F., Ding, C., Hua, C. (2014). Exogenous ascorbic acid and glutathione alleviate oxidative stress induced by salt stress in the chloroplasts of Oryza sativa L. Zeitschrift für Naturforschung C—A Journal of Biosciences, 69(5–6), 226–236. [Google Scholar] [PubMed]
30. Xu, C., Leskovar, D. I. (2015). Effects of A. nodosum seaweed extracts on spinach growth, physiology and nutrition value under drought stress. Scientia Horticulturae, 183, 39–47. [Google Scholar]
31. Terzi, R., Kalaycıoglu, E., Demiralay, M., Kadioglu, A. S. (2015). Exogenous ascorbic acid mitigates accumulation of abscisic acid, proline and polyamine under osmotic stress in maize leaves. Acta Physiolgiae Plantarum, 37, 1–9. [Google Scholar]
32. Akram, M. A., Sajid, M., Ahmad, A., Waqar, M., Kusar, S. et al. (2022). Effect of foliar spray of ascorbic acid on nodulation, gas exchange attributes and mineral ion contents of Pisum sativum under zinc stress. Plant Protection, 6(2), 101–111. [Google Scholar]
33. Amin, B., Mahleghah, G., Mahmood, H. M. R., Hossein, M. (2009). Evaluation of interaction effect of drought stress with ascorbate and salicylic acid on some of physiological and biochemical parameters in okra (Hibiscus esculentus L.). Research Journal of Biological Sciences, 4(4), 380–387. [Google Scholar]
34. Khalil, S. E., Nahed, G., Aziz, A., Bedour, L. A. H. (2010). Effect of water stress and ascorbic acid on some morphological and biochemical composition of Ocimum basilicum plant. The Journal of American Science, 6(4), 33–46. [Google Scholar]
35. Hafez, E. M., Gharib, H. S. (2016). Effect of exogenous application of ascorbic acid on physiological and biochemical characteristics of wheat under water stress. International Journal of Plant Production, 10(4), 579–596. [Google Scholar]
36. Darvishan, M., Tohidi-Moghadam, H. R., Zahedi, H. (2013). The effect of foliar application of ascorbic acid (vitamin C) on physiological and biochemical changes of corn (Zea mays L.) under irrigation withholding in different growth stages. Maydica, 58(1), 195–200. [Google Scholar]
37. Amira, M. S., Qados, A. (2014). Effect of ascorbic acid antioxidant on soybean (Glycine max L.) plants grown under water stress conditions. International Journal of Advanced Research in Biological Sciences, 1(5), 189–205. [Google Scholar]
38. Razaji, A., Farzanian, M., Sayfzadeh, S. (2014). The effects of seed priming by ascorbic acid on some morphological and biochemical aspects of rapeseed (Brassica napus L.) under drought stress condition. International Journal of Biosciences, 4(1), 432–442. [Google Scholar]
39. Zonouri, M., Javadi, T., Ghaderi, N., Saba, M. K. (2014). Effect of foliar spraying of ascorbic acid on chlorophyll a, chlorophyll b, total chlorophyll, carotenoids, hydrogen peroxide, leaf temperature and leaf relative water content under drought stress in grapes. Bulletin of Environment, Pharmacology and Life Sciences, 3, 178–184. [Google Scholar]
40. Abid, M., Malik, S. A., Bilal, K., Wajid, R. A. (2002). Response of okra (Abelmoschus esculentus L.) to EC and SAR of irrigation water. International Journal of Agriculture and Biology, 4(3), 311–314. [Google Scholar]
41. Saleem, A., Ashraf, M., Akram, N. A. (2011). Salt (NaCl)-induced modulation in some key physio-biochemical attributes in okra (Abelmoschus esculentus L.). Journal of Agronomy and Crop Science, 197(3), 202–213. [Google Scholar]
42. Saleem, A., Ashraf, M., Akram, N. A., Al-Qurainy, F. (2012). Salinity-induced changes in key anti-oxidant enzyme activities and in the levels of some anti-oxidants, osmo-protectants, inorganic ions, and chlorophyll pigments in okra fruit (Abelmoschus esculentus L.). The Journal of Horticultural Science and Biotechnology, 87(3), 271–277. [Google Scholar]
43. Ahmed, S., Stepp, J. R., Kapuscinski, A. R., Méndez, E. (2016). Beyond yields: Climate change effects on specialty crop quality and agroecological management. Elementa; Science of Anthropocene, 4, 000092. [Google Scholar]
44. Wang, F. B., Ren, G. L., Li, F. S., Qi, S. T., Xu, Y. et al. (2018). A chalcone synthase gene AeCHS from Abelmoschus esculentus regulates flavonoids accumulation and abiotic stress tolerance in transgenic Arabidopsis. Acta Physiolgiae Plantarum, 40, 97. [Google Scholar]
45. Calisir, S., Ozcaan, M., Haciseferogullari, H., Yidiz, M. U. (2005). A study on some physico-chemical properties of Turkey okra (Hibiscus esculentus L.) seeds. Journal of Food Engineering, 68(1), 73–78. [Google Scholar]
46. Kader, A., Perkins, V. P. M., Lester, G. E. (2004). Nutritional quality of fruits, nuts and vegetables and their importance in human health. In: USDA agriculture handbook 66. Davis, CA, USA: University of California. [Google Scholar]
47. Gest, N., Gautier, H., Stevens, R. (2013). Ascorbate as seen through plant evolution: The rise of a successful molecule? Journal of Experimental Botany, 64(1), 33–53. [Google Scholar] [PubMed]
48. Gupta, K., Sengupta, A., Chakraborty, M., Gupta, B. (2016). Hydrogen peroxide and polyamines act as double-edged swords in plant abiotic stress responses. Frontiers in Plant Science, 7, 1343. [Google Scholar] [PubMed]
49. Mukherjee, S. P., Choudhuri, M. A. (1983). Implications of water stress-induced changes in the levels of endogenous ascorbic acid and hydrogen peroxide in Vigna seedlings. Physiologia Plantarum, 58(2), 166–170. [Google Scholar]
50. Arnon, D. T. (1949). Copper enzymes in isolated chloroplasts polyphenol-oxidase in Beta vulgaris. Journal of Plant Physiology, 24(1), 1–15. [Google Scholar]
51. Bates, L. S., Waldren, R. P., Teare, L. D. (1973). Rapid determination of free proline for water-stress studies. Plant and Soil, 39, 205–207. [Google Scholar]
52. Velikova, V., Yordanov, I., Adreva, A. (2000). Oxidative stress and some antioxi-dant systems in acid rain treated bean plants: Protective role of exogenous polyamines. Plant Science, 151(1), 59–66. [Google Scholar]
53. Heath, R., Packer, L. (1968). Photoperoxidation in isolated chloroplast I. Kinetics and stoichiometry of fatty acid peroxidation. Archives of Biochemistry and Biophysics, 125(1), 189–198. [Google Scholar] [PubMed]
54. Julkenen-Titto, R. (1985). Phenolic constituents in the leaves of northern willows: Methods for the analysis of certain phenolics. Journal of Agricultural and Food Chemistry, 33(2), 213–217. [Google Scholar]
55. Grieve, C. M., Grattan, S. R. (1983). Rapid assay for determination of water-soluble quaternary ammonium compounds. Plant and Soil, 70, 303–307. [Google Scholar]
56. Van Rossum, M. W. P. C., Alberda, M., van der Plas, L. H. W. (1997). Role of oxidative damage in tulip bulb scale micropropagation. Plant Science, 130(2), 207–216. [Google Scholar]
57. Chance, M., Maehly, A. C. (1955). Assay of catalases and peroxidases. Methods in Enzymology, 2, 764–817. [Google Scholar]
58. Bradford, M. M. (1976). A rapid and sensitive method for microgram quantities of protein utilizing the principle of protein-dye binding. Analytical Biochemistry, 72, 248–254. [Google Scholar] [PubMed]
59. Hamilton, P. B., van Slyke, D. D., Lemish, S. (1943). The gasometric determination of free amino acids in blood filtrates by the ninhydrin-carbon dioxide method. Journal of Biological Chemistry, 150(1), 231–250. [Google Scholar]
60. Yemm, E. W., Willis, A. J. (1954). The estimation of carbohydrates in plant extract by anthrone. Biochemical Journal, 57(3), 508–514. [Google Scholar] [PubMed]
61. Briglia, N., Petrozza, A., Hoeberichts, F. A., Verhoef, N., Povero, G. (2019). Investigating the impact of biostimulants on the row crops corn and soybean using high-efficiency phenotyping and next generation sequencing. Agronomy, 9(11), 761. [Google Scholar]
62. Campobenedetto, C., Mannino, G., van Arkel, J., Beekwilder, J., Karolova, R. et al. (2020). A biostimulant seed treatment improved heat stress tolerance during cucumber seed germination by acting on the antioxidant system and glyoxylate cycle. Frontiers in Plant Science, 11, 836. [Google Scholar] [PubMed]
63. Deeba, F., Pandey, A. K., Ranjan, S., Mishra, A., Singh, R. et al. (2012). Physiological and proteomic responses of cotton (Gossypium herbaceum L.) to drought stress. Plant Physiology and Biochemistry, 53, 6–18. [Google Scholar] [PubMed]
64. Okunlola, G. O., Olatunji, O. A., Akinwale, R. O., Tariq, A., Adelusi, A. A. (2017). Physiological response of the three most cultivated pepper species (Capsicum spp.) in Africa to drought stress imposed at three stages of growth and development. Scientia Horticulturae, 224, 198–205. [Google Scholar]
65. Zhang, S. H., Xu, X. F., Sun, Y. M., Zhang, J. L., Li, C. Z. (2018). Influence of drought hardening on the resistance physiology of potato seedlings under drought stress. Journal of Integrative Agriculture, 17(2), 336–347. [Google Scholar]
66. Khan, A., Ahmad, M., Athar, H., Ashraf, M. (2006). Interactive effect of foliarly applied ascorbic acid and salt stress on wheat (Triticum aestivum L.) at the seedling stage. Pakistan Journal of Botany, 38(5), 1407–1414. [Google Scholar]
67. Akram, N. A., Iqbal, M., Muhammad, A., Ashraf, M., Al-Qurainy, F. et al. (2018). Aminolevulinic acid and nitric oxide regulate oxidative defense and secondary metabolisms in canola (Brassica napus L.) under drought stress. Protoplasma, 255(1), 163–174. [Google Scholar] [PubMed]
68. Ashraf, M., Harris, P. J. C. (2013). Photosynthesis under stressful environments: An overview. Photosynthetica, 51, 163–190. [Google Scholar]
69. Amirjani, M. R., Mahdiyeh, M. (2013). Antioxidative and biochemical responses of wheat to drought stress. Journal of Agriculture and Biological Sciences, 8(4), 291–301. [Google Scholar]
70. Bhardwaj, S., Kapoor, D. (2021). Fascinating regulatory mechanism of silicon for alleviating drought stress in plants. Plant Physiology and Biochemistry, 166, 1044–1053. [Google Scholar] [PubMed]
71. Naz, H., Akram, N. A., Ashraf, M. (2016). Impact of ascorbic acid on growth and some physiological attributes of cucumber (Cucumis sativus) plants under water-deficit conditions. Pakistan Journal of Botany, 48(3), 877–883. [Google Scholar]
72. Sharma, A., Shahzad, B., Kumar, V., Kohli, S. K., Sidhu, G. P. S. et al. (2019). Phytohormones regulate accumulation of osmolytes under abiotic stress. Biomolecules, 9(7), 285. [Google Scholar] [PubMed]
73. Akram, N. A., Waseem, M., Ameen, R., Ashraf, M. (2016). Trehalose pretreatment induces drought tolerance in radish (Raphanus sativus L.) plants: Some key physio-biochemical traits. Acta Physiologiae Plantarum, 38, 3. [Google Scholar]
74. Demiral, T., Turkan, I. (2004). Does exogenous glycinebetaine affect antioxidative system of rice seedlings under NaCl treatment. Journal of Plant Physiology, 161(10), 1089–1100. [Google Scholar] [PubMed]
75. Sarker, U., Oba, S. (2018). Drought stress effects on growth, ROS markers, compatible solutes, phenolics, flavonoids, and antioxidant activity in Amaranthus tricolor. Applied Biochemistry and Biotechnology, 186, 999–1016. [Google Scholar] [PubMed]
76. Gechev, T. S., Hille, J. (2005). Hydrogen peroxide as a signal controlling plant programmed cell death. Journal of Cell Biology, 168(1), 17–20. [Google Scholar] [PubMed]
77. Bhattacharjee, S. (2012). An inductive pulse of hydrogen peroxide pretreatment restores redox-homeostasis and oxidative membrane damage under extremes of temperature in two rice cultivars. Plant Growth Regulation, 68, 395–410. [Google Scholar]
78. Ju, Y. L., Yue, X. F., Zhao, X. F., Zhao, H., Fang, Y. L. (2018). Physiological, micro-morphological and metabolomic analysis of grapevine (Vitis vinifera L.) leaf of plants under water stress. Plant Physiology and Biochemistry, 130, 501–510. [Google Scholar] [PubMed]
79. Nie, W., Gong, B., Chen, Y., Wang, J., Wei, M. et al. (2018). Photosynthetic capacity, ion homeostasis and reactive oxygen metabolism were involved in exogenous salicylic acid increasing cucumber seedlings tolerance to alkaline stress. Scientia Horticulturae, 235, 413–423. [Google Scholar]
80. Cheng, L., Cheng, M., Han, L. M., Yang, L., Sun, Z. (2018). Changes in the physiological characteristics and baicalin biosynthesis metabolism of Scutellaria baicalensis Georgi under drought stress. Industrial Crops and Products, 122, 473–482. [Google Scholar]
81. Gao, S., Wang, Y., Yu, S., Huang, Y., Liu, H. (2020). Effects of drought stress on growth, physiology and secondary metabolites of two adonis species in Northeast China. Scientia Horticulture, 259, 108795. [Google Scholar]
82. Malik, S., Ashraf, M. (2012). Exogenous application of ascorbic acid stimulates growth and photosynthesis of wheat (Triticum aestivum L.) under drought. Soil and Environment, 31(1), 72–77. [Google Scholar]
83. Malik, S., Ashraf, M., Arshad, M., Malik, T. A. (2015). Effect of ascorbic acid application on physiology of wheat under drought stress. Pakistan Journal of Agricultural Sciences, 52, 209–217. [Google Scholar]
84. Ashraf, M. (2009). Biotechnological approach of improving plant salt tolerance using antioxidants as markers. Biotechnology Advances, 27(1), 84–93. [Google Scholar] [PubMed]
85. Jabeen, M., Akram, N. A., Ashraf, M., Tayagi, A., El-Sheikh, M. A. et al. (2022). Thiamin stimulates growth, yield quality and key biochemical processes of cauliflower (Brassica oleracea L. var. Botrytis) under arid conditions. PLoS One, 17(5), e0266372. [Google Scholar] [PubMed]
86. Cao, Y., Luo, Q., Tian, Y., Meng, F. (2017). Physiological and proteomic analyses of the drought stress response in Amygdalus mira (Koehne) Yu et Lu roots. BMC Plant Biology, 17, 53. [Google Scholar] [PubMed]
87. Ejaz, B., Sajid, Z. A., Aftab, F. (2012). Effect of exogenous application of ascorbic acid on antioxidant enzyme activities, proline contents, and growth parameters of Saccharum spp., hybrid cv. HSF-240 under salt stress. Turkish Journal of Biology, 36(6), 630–640. [Google Scholar]
88. Halimeh, R., Mahlagh, G., Maryam, P., Pazoki, A. (2013). Effect of drought interactions with ascorbate on some biochemical parameters and antioxidant enzymes activities in Dracocephalum moldavica L. Middle-East Journal of Scientific Research, 13(4), 522–531. [Google Scholar]
Cite This Article
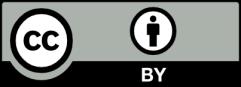
This work is licensed under a Creative Commons Attribution 4.0 International License , which permits unrestricted use, distribution, and reproduction in any medium, provided the original work is properly cited.