Open Access
ARTICLE
The Impact of Inoculum Preparation Media on Pollutant Removal through Phycoremediation of Agricultural Drainage Water by Desmodesmus sp.
1
Plant Physiology Division, Department of Agricultural Botany, Faculty of Agriculture, Cairo University, Giza, P. O. Box 12613,
Egypt
2
Algal Biotechnology Unit, National Research Centre, Dokki, Giza, 12622, Egypt
3
Biological and Geological Science Department, Faculty of Education, Ain Shams University, Cairo, 11341, Egypt
* Corresponding Authors: Heba I. Mohamed. Email: ; Ayman Amin. Email:
(This article belongs to the Special Issue: Molecular and Cellular Communication Between Plants and Plant Growth-Promoting Microorganisms and Their Nanoparticles for Abiotic and Biotic Stress Tolerance)
Phyton-International Journal of Experimental Botany 2023, 92(10), 2875-2890. https://doi.org/10.32604/phyton.2023.031064
Received 11 May 2023; Accepted 13 July 2023; Issue published 15 September 2023
Abstract
Water is the most essential natural resource for the future development. Agriculture production is extensively water-dependent and a significant polluter of water resources. So, this work investigated the effect of two different preparation media [Bold’s Basal Medium (BBM) and Domiati cheese whey (DCW)] for agricultural drainage water (ADW) remediation. All treatments were incubated for 6 days. According to the results of biomass productivity, specific growth rate, photosynthetic pigments, and biochemical composition, Desmodesmus sp. can grow in drainage water without dilution. The two treatments significantly reduced the concentration of nitrate, phosphate, chemical oxygen demand, and sodium in ADW. Finally, using cheese whey with BBM as inoculum preparation media enhanced the lipid accumulation in the algal biomass (25.4% w/w) and gave the best biodiesel properties among treatments. Therefore, combining remediation of drainage water with microalgae mass production can achieve the sustainability of agriculture.Keywords
Agriculture is the world’s largest user of water, using 70% of the freshwater available and consuming more than 90% of it. Additionally, the demand for freshwater resources worldwide is greatly increased by population and economic growth [1]. Egypt’s water resources are becoming increasingly scarce [2]. Population growth is one of the most effective factors in the water problem in Egypt [3], whereas the rate of population growth from 1989 to 2018 was 2.1% annually [1]. This increasing population puts pressure on limited water supplies due to an increase in the demand for food and other products in addition to direct consumption [2]. The application of fertilizers is a way to meet the increasing food demands, but the excessive use of synthetic fertilizers, especially nitrogen, does not always help to increase the yield but is usually lost through denitrification, leaching, or volatilization [4]. Many different types of persistent organic pollutants (POPs) are being released into surface water bodies as a result of these synthetic chemicals. Excessive nutrient stress causes a variety of micro and macro species to thrive in water bodies, causing an ecological imbalance in the nutrient cycle and unabated water pollution in the majority of countries [1]. These leached fertilizers pollute soil and water, which causes serious environmental problem as aquatic eutrophication. The concentrations of soluble forms of heavy metals including Cu, Ni, Pb, and Zn are often higher in soil that has been fertilized with nitrogen, phosphorus, and potassium (NPK) and manure [4]. The eutrophication impact occurs when the growth of algae and microorganisms becomes extreme in the water body which causes destructive impacts on other aquatic organisms, birds, and even people [5].
The treatment of wastewater is very important to meet water demands. The removal of nutrients and heavy metals is required for the discharge or reuse of wastewater, but during 2010 to 2017, 8.1 Km3/y of surface drainage water was used directly in irrigation without treatment [2], which contributed to increasing soil salinity and polluting groundwater with fertilizers and pesticides [6,7]. Applying the same traditional methods that use municipal wastewater treatment for agricultural runoff is not economical [7], which is encouraging many to look towards more eco-friendly and economic options.
The concept of phycoremediation means using algae (such as microalgae, macroalgae, and blue-green algae) in wastewater remediation to remove inorganic or organic pollutants [8,9]. Whereas microalgae are microorganisms that can utilize light, CO2, and inorganic nutrients and produce biomass through the process of photosynthesis. Like plants, microalgae can assimilate nitrogen in the forms of nitrate, nitrite, urea, and ammonium [10], as well as phosphorus in the forms of polyphosphate and orthophosphate [11] that made microalgae able to recycle inorganic fertilizers which leached into agricultural drainage water. Also, microalgae are able to breakdown organic matter, remove heavy metals, and control the levels of chemical oxygen demand (COD) and biological oxygen demand (BOD) in water bodies [12,13].
Interestingly, there are many algae genera such as Chlorella, Scenedesmus, Desmodesmus, and Botryococcus that can remove nutrients and other pollutants from contaminated water [8,14], because microalgae are able to grow under different trophic modes (such as phototrophic, heterotrophic, mixotrophic, and photoheterotrophic) of nutrition [9]. Algae are able to adapt several environmental conditions, including salinity, light intensity, and a wide range of temperatures [9]. Additionally, microalgal biomass has a wide range of uses, from the production of bioenergy to pharmaceuticals, with some varieties of microalgae being used for human and animal nutrition [15]. Microalgae can store a lot of lipids that are suitable for making biodiesel and have characteristics that meet the requirements of the majority of industrialized nations [16]. Following the extraction of fatty acids, biomass residue can be used to make bio-oil, bio-crude, ethanol and methane [16]. Microalgal biomass looks to be a rich resource for human food and animal feed applications due to its high concentrations of carbohydrates, proteins, vitamins, and important minerals [17]. Additionally, microalgae are widely used in the cosmetic and pharmaceutical industries as a source of vital vitamins, antioxidants, pigments and amino acids [18]. Finally, microalgae excrete compounds that can affect the growth and development of plants [19]. Growth-promoting elements, vitamins, amino acids, polypeptides, polymers, and antibacterial and antifungal agents are a few examples of these substances [20]. Moreover, algal biomass has many biotechnological applications in nutrition for humans and animals, bio-fertilizers, biofuel, pharmaceutical and cosmetics production [21]. These applications make phycoremediation more economical.
Castellanos-Estupiñan et al. [22] reported that Hapalosyphon sp., Chlorella sp. and Scendesmus sp. can grow in agricultural runoff without dilution in the presence of harmful pesticides (such as chlorpyrifos). The robust green microalga Desmodesmus sp. has the potential for wastewater remediation [23], Desmodesmus has features like the genus Scenedesmus, these species are regarded as powerful microalgae with a strong ability to survive environmental change [24] such as salinity stress [25]. Chlorella vulgaris can grow in drainage water as an alternative medium [26].
The objective of this study was to assess Desmodesmus sp. capacity to flourish in agricultural runoff as a substitute source of nutrients for biomass production and wastewater treatment and also study the effects of the inoculum preparation media on pollutant elimination, algal growth, and physiological responses. In addition, the ultrastructure and biochemical composition of Desmodesmus sp. were studied.
2.1 Agricultural Drainage Water
The agricultural drainage water was taken from the drainage manholes of the subsurface drainage system of the clover production fields in the Menoufia governorate, Egypt, in November 2021. The effluents were prepared by filtration through filter paper No. 1 and sterilized by autoclave (120°C, 20 min) for cultivation. Table 1 shows the properties of drainage water used in a recent study.
Desmodesmus sp. was obtained from Algal Biotechnology Unite, National Research Centre, Cairo, Egypt. The culture was kept in liquid media with an aseptic method in 500 mL Bold’s Basal Medium (BBM) media as an active growing culture [27].
Desmodesmus sp., which was pre-cultivated on various growth media (BBM) and Domiati cheese whey (DCW) combined with BBM (15% DCW + 50% BBM) for 7 days under cool fluorescent light (5000 lux) at 25°C ± 2°C and 16:8 h light:dark cycle in a 1 litter glass flask with a working volume of 0.7 litter, was used to treat drainage water in the experiment. The media was mixed with sterilized air supplied by an air pump with a 5 W motor and a maximum flow rate.
All treatments were prepared in flasks of 1 liter with a working volume of 700 mL and autoclaved at 121°C, 1.5 bar, for 20 min. Under aspect conditions, three replicates of BBM were inoculated with Desmodesmus sp., (T1), three replicates of drainage water were inoculated with Desmodesmus sp., which was pre-cultivated on BBM (T2), and another three replicates were inoculated with Desmodesmus sp., which was cultivated on 15% DCW + 50% BBM (T3).
Drainage water was used without dilution. The initial algal concentration for each replicate was set approximately to 0.12 g L−1 (day 0). The pH of all cultures was adjusted to 7 and incubated for 6 days under the same growth conditions of pre-cultivation. Dry weight, pigments, and pH were monitored at a 2-day interval and, total protein, total carbohydrates, total lipids and fatty acids composition were determined at the end of the experiments.
2.3.1 Microalgae Growth Determination
Desmodesmus sp. growth was determined through dry weight (DW) measurement, specific growth rate (µ) and biomass productivity calculation. For dry weight, 10 mL of homogenized Desmodesmus sp. culture was filtered over a pre-weighted (W1) sartorius cellulose nitrate sterile membrane filter (pore size 0.45 µm). Filters were dried at 105°C in a dry oven (until getting a constant weight). Dried filters were kept over anhydrous calcium chloride until room temperature and re-weighted (W2). The difference between weights (W1 and W2) indicated the net dry weight of the grown alga within a defined sampling time.
At the exponential growth phase, Desmodesmus sp. specific growth rate and biomass productivity were calculated according to Liang et al. [28] by the following formulae (1) and (2):
Specific growth rate μ(d−1)=LnN2−N1T2−T1 (1)
Biomass productivity(g l−1d−1)=N2−N1T2−T1 (2)
whereas, N1 and N2 are the biomass at the initial time (T1) and final time (T2), respectively.
For further biochemical analysis, Desmodesmus sp. biomass was harvested by sedimentation and further centrifuged at 4000 rpm for 10 min. The obtained algal slurry was washed with distilled water to remove salts and then dried in an oven at 70°C overnight.
2.3.2 Pollutants Removal Efficiency
The concentrations of chemical oxygen demand (COD), nitrate (NO3), phosphate (PO4) and sodium (Na+) were measured according to Rice et al. [29] to monitor the efficiency of pollutant removal before and after agriculture drainage water treatment. 100 mL of culture broth from each experiment replicate was taken after 6 days from the beginning of the experiment. Samples were centrifuged and then filtrated with 0.45 µm membrane filter (Sartorius cellulose nitrate sterile membrane filter).
Pollutant removal efficiency(%)=CI−CFCI×100 (3)
where CI is the initial and CF is the final concentration of target pollutants.
2.3.3 Photosynthetic Pigments Measurement
The photosynthetic pigment content (chlorophyll a (Chl a), chlorophyll b (Chl b), and total carotenoids) of Desmodesmus sp. were extracted using dimethyl sulfoxide (DMOS) and determined according to Wellburn [30].
The concentration of the pigments was then calculated based on absorbance (A) using the formulae (4)–(6) as follows:
Chla(μg/mL)=12.47×A665−3.62 ×A649 (4)
Chlb(μg/mL)=25.06×A649−6.5×A665 (5)
Carotenoids (μg/mL)=(1000×A480−1.29×Chl a−53.78×Chl b)/220 (6)
Carbohydrate content was measured according to the method of Dubois et al. [31], using glucose as a standard. To make a known sample concentration (1 mg mL−1) for each sample, biomass (10 mg) was mixed in water (10 mL). A water bath was used to react aliquots of the 1 mL sample with 3 mL of concentrated H2SO4 (72%) and 1 mL of phenol (5%, w/v). At 90°C, the mixes were incubated for 5 min. Then, a spectrophotometer was used to measure the absorbance at 490 nm.
The elemental CHNS analyzer assessed the dried biomass’ nitrogen content. Using pure oxygen (200 mLmin−1) as the combustion gas and pure helium (the pressure of the gas is 1.41 Pa), the samples (2 mg) were inserted in tin capsules and heated to between 900°C and 1150°C for combustion. The oven was also fitted with a thermal conductivity detector (TCD). According to Lourenço et al. [32], 4.78 were used as the nitrogen-to-protein conversion factor instead of 6.25.
2.3.6 Lipid Quantification by Gravimetric Method
The lipid was extracted from microalgal dried biomass according to the procedure of Bligh et al. [33]. As a solvent, a 2:1 mixture of methanol and chloroform was combined with the dried biomass of microalgae, and the weight of the total lipids was then calculated gravimetrically.
2.4 Ultrastructure Analysis of Desmodesmus sp. Using Transmission Electron Microscope (TEM)
To know how treatments affected Desmodesmus sp., the cellular ultrastructure was studied using TEM (JEOL, Tokyo, Japan). Algal cells grown on all treatments were harvested after 6 days and used for TEM. The microalgal samples were fixed for 0.5 h at room temperature in a 2% (w/v) glutaraldehyde solution in a 0.1 M sodium cacodylate buffer and then postfixed for 4 h in a 1% (w/v) OsO4 solution in the same buffer. The materials were embedded in an epoxy resin after being dehydrated using a graded ethanol series. Ultrathin sections were made with a Leica ultracut UCT Ultramicrotome, stained with lead citrate and uranyl acetate according to Reynolds [34], and examined under Jeol JEM 1400 TEM in Cairo University Research Park (CURP).
Algal lipids were converted to fatty acid methyl ester (FAME) by using the methyl ester boron trifluoride method [35]. FAME was analyzed in a Shimadzu GC-2010, equipped with a Flame Ionization Detector (FID) as follows: injection volume 0.5–2 µL; column: RTX-wax (30 m length × 0.25 mm i.d. × 0.25 mm film thickness), oven temperature of 200°C; total analysis time of 30 min; and detector temperature of 250°C. Calibration curves between peak area and concentration were constructed by introducing reference FAME samples of known concentrations into the GC-FID. The carrier gas, helium (25 psi), was provided at a flow rate of 45.0 mLmin−1. The analysis occurred at the Regional Center for Food and Feed (RCFF), Agricultural Research Center, Giza, Egypt.
The biodiesel properties were analyzed with online software “BiodieselAnalyzer© Ver. 2.2” [36].
The figures and tables expressed the results as means of ± standard deviation (SD). Statistical analysis was carried out by GraphPad Prism 8 software (https://www.graphpad.com). All results were tested statistically using one-way analysis of variance (ANOVA) followed by the Tukey’s test. Differences were considered significant at p ≤ 0.05.
3.1 Growth and Pollutants Removal of Desmodesmus sp. in Drainage Water
Drainage water has been used as a culture medium for Desmodesmus sp. in batch experiments. As shown in Fig. 1a, Desmodesmus sp. was able to grow in undiluted drainage water (T2 and T3) similar to control (T1), so there were no significant differences among treatments, and Figs. 1b and 1c showed that biomass production and specific growth rate of microalga grown on T2 and T3 were quite similar when using BBM (T1), so there were also no significant differences among them.
Figure 1: Impact of drainage water on Desmodesmus sp. growth curve (a), biomass productivity (b), specific growth rate (c). Each value represents the mean of three replicates ± 1 standard deviation (SD). T1 = control (BBM); T2 = drainage water; T3 = 15% DCW+50% BBM)
Moreover, it can be found that Desmodesmus sp. growth on BBM (T1) and drainage water (T2 and T3) was similar during the incubation period. Although the composition of BBM and drainage water is very different, BBM is a medium with a high concentration of nutrients (182.4 mg L−1 nitrate and 163.2 mg L−1 phosphate) and is enriched with essential macro- and microelements for algae growth, but the drainage water has low concentrations of many essential elements (PO4, K, Fe, and Mn), as shown in Table 1. Nitrate availability in algal growth media leads to good growth and chlorophyll accumulation as well [37]. In addition, Roopnarain et al. [38] reported that the suitable concentration of phosphorus for microalgae is between 1 and 179 mg L−1. So Desmodesmus sp. is grown on drainage water, which has 1.7 mg L−1 phosphate.
Microalgae, on excess iron availability, are able to absorb and store high amounts of Fe as ferritins and release and utilize them under Fe limitations [39]. Because of that, Desmodesmus sp. grew well on drainage water despite the lack of iron during the incubation period. Drainage water, which was used in this study, has a high concentration of magnesium (Mg). Gorain et al. [40] recorded a significant increase in biomass yield and lipid yield when Chlorella vulgaris and Scenedesmus obliquus were grown under high concentrations of Mg.
Castellanos-Estupiñan et al. [22] cultivated Chlorella sp., Scenedesmus sp., and Hapalosiphon sp. on agriculture runoff after 20 days; recorded biomass yields were 1.0, 0.71, and 0.83 g L−1, respectively. In addition, García-Galán et al. [41] cultivated a mixture of microalgae on DW for 4 days and found algal biomass increased by 21.7% over first-day biomass. In this study, all treatments obtained a high biomass yield with a 300% increment as compared to zero time.
Comparing the results of drainage water analysis (Table 1) with standard limits of drinking water parameters of both the Egyptian and WHO standards, almost all pollutants and ions were within the permissible limits except COD, NO3, PO4, and Na+, which were over limits. The Nile Chemical Pollution Index records ranged from 13–19 indicating clean (less than 15) to slightly polluted (16–20) waters. After cultivation of Desmodesmus sp. for 6 days, COD, NO3, PO4, and Na+ removal efficiency in T2 were 67.36%, 99.78%, 100%, and 62.03%, respectively. The results of T3 were similar to those of T2, as shown in Fig. 2. The removal efficiency of COD, NO3, PO4, and Na+ was 65.11%, 99.60%, 100%, and 61.695%, respectively. There are no significant differences between T2 and T3 in the removal of COD, NO3, PO4 and Na+. Microalgae can use nitrogen to make biological compounds, including peptides, proteins, enzymes, chlorophylls, and genetic material. Several microalgae are capable of assimilating many types of inorganic (NO3, NO2, NH4) and organic nitrogen, including urea and amino acids [42]. So notably, in T2, the nitrate concentration sharply dropped from 155.75 to 0.29 mg L−1, while in T3, it dropped from 155.75 to 0.645 mg L−1 during 6 days of incubation. These findings were higher than the results reported by Castellanos-Estupiñan et al. [22] using Scenedesmus sp. (88% of NO3 removal rate) to treat agricultural runoff and Díez-Montero et al. [43] with a mixture of microalgae (85% of NO3 removal rate) for agricultural runoff and partially treated domestic wastewater.
Figure 2: Pollutants removal efficiency by Desmodesmus in different treatments (T2, T3) from drainage water. T1 = control (BBM); T2 = drainage water; T3 = 15% DCW+50% BBM). Each value represents the mean of three replicates ± 1 standard deviation
Phosphorus is required for the biosynthetic pathway of DNA, RNA, structural lipids (phospholipids), and components (such as adenosine triphosphate, ATP) essential in a cell’s energy metabolism. So, in this study, Desmodesmus sp. consumed total phosphate content from drainage water in T2 and T3. This result is similar to the results of Díez-Montero et al. [43], whose microalgae removed up to 99% of phosphate from agricultural runoff water, and Vazirzadeh et al. [44], who cultivated Chlorella vulgaris on agricultural runoff, which removed phosphate by more than 91%. Microalgae can remove Na+ and Cl− (desalination) from seawater and brackish water by two main mechanisms; bioabsorption and bioaccumulation [45]. Many microalgae have been investigated for their desalination potential, e.g., Scenedesmus sp. [46], Synechococcus sp. [47], C. vulgaris [48], Desmodesmus subspicatus and D. armatus [49]. Wang et al. [50] cultivated Chlorella sp. in different wastewaters and observed that the Na+ concentration dropped to 6.98%–12.05% after nine days.
The results in Figs. 3a–3c indicated that the highest concentrations of chlorophyll a and b, in addition to total carotenoid, were observed in Desmodesmus sp. cultures grown on BBM (T1). In contrast, the Chl a, Chl b and carotenoids content remarkably decreased in Desmodesmus sp. cultures grown on drainage water (T2 and T3). This decrease in photosynthetic pigments was observed on the 4th day of incubation and then increased on the 6th day because of nutrient limitation at the end of the incubation period. According to Table 1, DW had a high concentration of Mg2+ compared to BBM, but Fe3+ was limited, which reduced photosynthetic pigment. Fe and Mg2+ are considered essential elements for the photosynthesis pigments in algae [51].
Figure 3: Effect of drainage water on photosynthetic pigments of Desmodesmus sp. which grew on T2 and T3 as well as T1 (control) incubated for different intervals (2 days). T1 = control (BBM); T2 = drainage water; T3 = 15% DCW + 50% BBM). Each value represents the mean of three replicates ± 1 standard deviation (SD). **** indicate highly significant difference according to One-way ANOVA with Tukey’s test at ≤0.05
Biochemical composition (as carbohydrates, protein and lipid concentrations) of harvested algal biomass after 6 days of incubation, as affected by different treatments (T2, T3) compared to control (T1) is shown in Fig. 4. Desmodesmus sp., which grew on drainage water and accumulated carbohydrates and lipids more than that, grew on BBM. In addition, biomass from T2 and T3 contained higher amounts of carbohydrates than T1 by 37.13% and 38.46%, respectively. T3 showed the highest concentrations of lipids by about 25.4% w/w, which increased more than T1 and T2 by 10.04% and 9.88%, respectively. However, the control had the highest concentration of protein, which was higher than T2 and T3 by 53% and 51.3%, respectively. These findings differ from those of Castellanos-Estupiñan et al. [22], who found that agricultural runoff did not affect algal metabolic levels and lipids did not override 10% w/w while total protein increased by more than 40% w/w in all studied strains. According to these results, using DCW as an inoculum preparation medium enhanced the accumulation of lipids and carbohydrates in Desmodesmus sp. As a result of their capacity to thrive in wastewater and high lipid content generation, our microalgal strain (Desmodesmus sp.) is highly advised for biodiesel production, as reported by Ji et al. [52].
Figure 4: Biochemical composition of Desmodesmus sp. cultivated in drainage water (T2 and T3) as well as control for 6 days of incubation period. T1 = control (BBM); T2 = drainage water; T3 = 15% DCW+50% BBM). Each value represents the mean of three replicates ± 1 standard deviation (SD). **, *** and **** indicate significant and highly significant difference according to One-way ANOVA with Tukey’s test at p ≤ 0.05
3.4 Ultrastructure Analysis of Desmodesmus sp.
The results in Fig. 5 highlight the effect of different treatments on the ultrastructure of Desmodesmus sp., which is consistent with the previous results. It is obvious in the transmission electron micrographs (Fig. 5) that the chloroplast occupies a large part of the algal cell with a clear nucleus, pyrenoid, and some starch granules in T1. The ultrastructure of algal cells from T2 is similar to T1 ones, but in T2, Desmodesmus sp. accumulated more starch. While in T3, Desmodesmus sp. accumulated more carbohydrates and lipids, which are obvious as oil bodies in Fig. 5c. Microalgae begin lipid accumulation when cells enter the stationary phase and cell division stops; the exact timing of this event varies for individual cells within a population [53]. The reason for the formation of lipid drops in cells of T3 may be attributed to the combination of cheese whey with BBM in the inoculum preparation media.
Figure 5: Transmission electron micrographs of Desmodesmus sp. cultivated in BBM T1 (a), drainage water T2 (b), and 15% DCW+50% BBM) T3 (c). Ch: chloroplast, CW: cell wall, N: nucleus, OB: oil body, P: pyrenoid, SG: starch granule
3.5 Fatty Acids Profile of Desmodesmus sp.
The fatty acid composition of Desmodesmus sp. which is produced from T1, T2 and T3 is shown in Table 2. The isolate accumulated fatty acids in the range of C12:1 to C18:3. Palmitic acid was the predominant fatty acid in T1 (23.91%), T2 (24.94%) and T3 (26.44%), followed by oleic acid in T2 (21.11%) and T3 (23.14%). Moreover, linolenic acid was higher in T1 (23.50%) than in other treatments. Palmitic and oleic acids were also the major fatty acids in Desmodesmus sp., as reported by Chen et al. [54] and Shah et al. [55]. Lauric acid (C12:1) was found only in T2. The results in Table 2 highlight that Desmodesmus sp. cultivated in drainage water had a high content of saturated and mono-unsaturated fatty acids, which made biodiesel suitable for use in different weather environments due to its high oxidative stability and low melting point [56]. Antolın et al. [57] reported that biodiesel could possess a higher cetane number (CN), reduced NOx emissions, quicker ignition, and more oxidative stability by using more saturated oil.
The information in Table 3 shows the predicted biodiesel properties resulting from Desmodesmus sp. grown on BBM (T1) and DW (T2 and T3), with comparison to the American Society of Testing and Materials (ASTM) D6751 and the European (EN) 14214 standards. The biodiesel properties produced from the biomass of T3 were the best among all three treatments in this study. The iodine value (IV) is an important consideration for determining how stable a biodiesel is [58]. The better the gasoline, as indicated by a greater cetane number (CN), the lower the iodine value. With an increase in iodine levels, unsaturation and vulnerability to oxidation and rancidification increase. The iodine value of biodiesel produced from the control treatment was 105.01 g 100 g−1, while in T2 and T3; it was 95.17 and 86.70 g 100g−1. Both values were lower than European and American standards [59,60]. The majority of the biodiesel properties derived from the studied Desmodesmus sp. reportedly complied with international standards and met the limit values set by ASTM D6751 and EN 14214:2012+A2:2019 biodiesel standards, according to the literature, which generally supported the current findings shown in Table 3 [59–61].
Increasing levels of freshwater contaminants, mainly due to anthropogenic activities, have resulted in a great deal of interest in finding new eco-friendly, cost-effective, and efficient methods for remediating polluted waters. According to the study’s findings, Desmodesmus sp. may grow well in drainage water without dilution, which is the best way to generate a lot of algal biomass, lipid content, productivity and biodiesel. Desmodesmus sp. is a good choice for the mass production of biodiesel because it has also been found that its biodiesel characteristics are quite consistent with international biodiesel standards. The best biodiesel qualities among treatments came from utilizing cheese whey and BBM as the inoculum preparation media, which also increased lipid accumulation in the algal biomass.
Acknowledgement: The authors thank Dr. Marwa Reda Afife from National Water Research Center (NWRC) for carrying out the water analysis.
Funding Statement: The authors received no specific funding for this study.
Author Contributions: The authors confirm their contribution to the paper as follows: study conception and design: A.S., H.S., A.B.E., R.M.E. and A.A; data collection: A.S.; analysis and interpretation of results: A.S., H.S., A.B.E., R.M.E., H.I.M. and A.A.; draft manuscript preparation: A.S., H.I.M. and A.A. All authors reviewed the results and approved the final version of the manuscript.
Availability of Data and Materials: The datasets used and/or analyzed during the current study are available from the corresponding author on reasonable request.
Ethics Approval: Not applicable.
Conflicts of Interest: The authors declare that they have no conflicts of interest to report regarding the present study.
References
1. Sevugamoorthy, D., Rangarajan, S. (2023). Comparative analysis of biodegradation and characterization study on agal-assisted wastewater treatment in a bubble column photobioreactor. Environmental Challenges, 10, 100659. [Google Scholar]
2. Nikiel, C. A., Eltahir, E. A. (2021). Past and future trends of Egypt’s water consumption and its sources. Nature Communications, 12(1), 1. https://doi.org/10.1038/s41467-021-24747-9 [Google Scholar] [PubMed] [CrossRef]
3. Abdelkader, A., Elshorbagy, A., Tuninetti, M., Laio, F., Ridolfi, L. F. et al. (2018). National water, food, and trade modeling framework: The case of Egypt. Science of the Total Environment, 639, 485–496. [Google Scholar] [PubMed]
4. Ahmed, M. A., Rauf, M. A., Mukhtar, Z. A., Saeed, N. A. (2017). Excessive use of nitrogenous fertilizers: An unawareness causing serious threats to environment and human health. Environmental Science Pollution Research, 24, 26983–26987. https://doi.org/10.1007/s11356-017-0589-7 [Google Scholar] [PubMed] [CrossRef]
5. Ahmadi, N., Nadoushan, M. A., Abolhasani, M. H., Hosseini, A. (2022). A case study on the efficacy of oil contaminants biological remediation using Scenedesmus obliquus algae. Environmental Engineering Management Journal, 21(1), 1–13. [Google Scholar]
6. FAO (2017). Water for sustainable food and agriculture: A report produced for the 20 G presidency of Germany, food and agriculture organization of the united nations, Rome. https://www.fao.org/3/i7959e/i7959e.pdf [Google Scholar]
7. Cai, T., Park, S. Y., Li, Y. (2013). Nutrient recovery from wastewater streams by microalgae: Status and prospects. Renewable and Sustainable Energy Reviews, 19, 360–369. https://doi.org/10.1016/j.rser.2012.11.030 [Google Scholar] [CrossRef]
8. Cuellar-Bermudez, S. P., Aleman-Nava, G. S., Chandra, R., Garcia-Perez, J. S., Contreras-Angulo, J. R. et al. (2017). Nutrients utilization and contaminants removal. A review of two approaches of algae and cyanobacteria in wastewater. Algal Research, 24, 438–449. [Google Scholar]
9. Pacheco, M. M., Hoeltz, M., Moraes, M. S., Schneider, R. C. (2015). Microalgae: Cultivation techniques and wastewater phycoremediation. Journal of Environmental Science and Health, Part A, 50(6), 585–601. [Google Scholar]
10. Xu, N., Zhang, X., Fan, X., Han, L., Zeng, C. et al. (2001). Effects of nitrogen source and concentration on growth rate and fatty acid composition of Ellipsoidion sp. (Eustigmatophyta). Journal of Applied Phycology, 13(6), 463–469. [Google Scholar]
11. Yaakob, M. A., Mohamed, R. M. S. R., Al-Gheethi, A., Ravishankar, G. A., Ambati, R. R. (2021). Influence of nitrogen and phosphorus on microalgal growth, biomass, lipid, and fatty acid production: An overview. Cells, 10(2), 1–19. https://doi.org/10.3390/cells10020393 [Google Scholar] [PubMed] [CrossRef]
12. Jais, N. M., Mohamed, R. M. S. R., Al-Gheethi, A. A., Hashim, M. K. A. (2017). The dual roles of phycoremediation of wet market wastewater for nutrients and heavy metals removal and microalgae biomass production. Clean Technologies and Environmental Policy, 19(1), 37–52. https://doi.org/10.1007/s10098-016-1235-7 [Google Scholar] [CrossRef]
13. Yaakob, M. A., Mohamed, R. M. S. R., Al-Gheethi, A., Tiey, A., Kassim, A. H. M. (2019). Optimising of Scenedesmus sp. biomass production in chicken slaughterhouse wastewater using response surface methodology and potential utilisation as fish feeds. Environmental Science and Pollution Research, 26(12), 12089–12108. https://doi.org/10.1007/s11356-019-04633-0 [Google Scholar] [PubMed] [CrossRef]
14. Gumbi, S. T., Mutanda, T., Olaniran, A. O. (2021). Nutrient removal from dairy and poultry wastewater with simultaneous biomass and biodiesel production by Chlorella sp. T4 isolated from a freshwater stream in South Africa. Waste and Biomass Valorization, 12, 6931–6943. https://doi.org/10.1007/s12649-021-01492-0 [Google Scholar] [CrossRef]
15. Khoo, K. S., Ahmad, I., Chew, K. W., Iwamoto, K., Bhatnagar, A. et al. (2023). Enhanced microalgal lipid production for biofuel using different strategies including genetic modification of microalgae: A review. Progress in Energy and Combustion Science, 96, 101071. [Google Scholar]
16. Sathya, A. B., Thirunavukkarasu, A., Nithya, R., Nandan, A., Sakthishobana, K. et al. (2023). Microalgal biofuel production: Potential challenges and prospective research. Fuel, 332, 126199. [Google Scholar]
17. El-Beltagi, H. S., Mohamed, A. A., Mohamed, H. I., Ramadan, K. M. A., Barqawi, A. A. et al. (2022). Phytochemical and potential properties of seaweeds and their recent applications: A review. Marine Drugs, 20(6), 342. https://doi.org/10.3390/md20060342 [Google Scholar] [PubMed] [CrossRef]
18. Gonçalves, A. L. (2021). The use of microalgae and cyanobacteria in the improvement of agricultural practices: A review on their biofertilising, biostimulating and biopesticide roles. Applied Sciences, 11(2), 871. [Google Scholar]
19. Quintas-Nunes, F., Brandão, P. R., Barreto Crespo, M. T., Glick, B. R., Nascimento, F. X. (2023). Plant growth promotion, phytohormone production and genomics of the rhizosphere-associated microalga, Micractinium rhizosphaerae sp. nov. Plants, 12(3), 651. [Google Scholar] [PubMed]
20. Parmar, P., Kumar, R., Neha, Y., Srivatsan, V. (2023). Microalgae as next generation plant growth additives: Functions, applications, challenges and circular bioeconomy based solutions. Frontiers in Plant Science, 14, 299. [Google Scholar]
21. Miguel, A. V., Antonio, F., Jose, C. M., Pires Ana, L. G. (2020). CO2 capture using microalgae. Advances in Carbon Capture, 2020. https://doi.org/10.1016/B978-0-12-819657-1.00017-7 [Google Scholar] [CrossRef]
22. Castellanos-Estupiñan, M. A., Carrillo-Botello, A. M., Rozo-Granados, L. S., Becerra-Moreno, D., García-Martínez, J. B. et al. (2022). Removal of nutrients and pesticides from agricultural runoff using microalgae and cyanobacteria. Water, 14(4), 558. https://doi.org/10.3390/w14040558 [Google Scholar] [CrossRef]
23. Sarfraz, R., Taneez, M., Sardar, S., Danish, L., Hameed, A. (2021). Evaluation of Desmodesmus subspicatus for the treatment of wastewater. International Journal of Environmental Analytical Chemistry. https://doi.org/10.1080/03067319.2021.1910681 [Google Scholar] [CrossRef]
24. Ferreira, G. F., Pinto, L. F., Maciel Filho, R., Fregolente, L. V. (2022). Maximizing unsaturated fatty acids production by using sugarcane agroindustry wastes in cultivation of Desmodesmus sp. in a flat plate photobioreactor. Journal of Biotechnology, 360, 117–124. [Google Scholar] [PubMed]
25. El-Sayed, A. B. (2010). Circulation of quaron lake wastes I-solidification of magnesium salts and complementary demineralization by the green alga scenedesmus sp. Journal of American Science, 6(9), 870–875. [Google Scholar]
26. Reda, M. M., El-Sayed, A. E. K. B., Almutairi, A. W., Hassoub, M. A. (2020). Fatty acid profiles and fuel properties of oils from castor oil plants irrigated by microalga-treated wastewater. Egyptian Journal of Botany, 60(3), 797–804. https://doi.org/10.21608/ejbo.2020.22970.1444 [Google Scholar] [CrossRef]
27. Bischoff, H. W., Bold, H. C. (1963). Phycological studies IV. Some soil algae from enchanted rock and related algal species. University of Texas Publication, 6318, 1–95. [Google Scholar]
28. Liang, F., Wen, X., Geng, Y., Ouyang, Z., Luo, L. et al. (2013). Growth rate and biomass productivity of chlorella as culture depth and cell density in an open circular photobioreactor. Journal of Microbiology and Biotechnology, 23(4), 539–544. [Google Scholar] [PubMed]
29. Rice, E. W., Bridgewater, L., Association, A. P. H. (2012). Standard methods for the examination of water and wastewater, vol. 10. Washington DC: American Public Health Association. [Google Scholar]
30. Wellburn, A. R. (1994). The spectral determination of chlorophylls a and b, as well as total carotenoids, using various solvents with spectrophotometers of different resolution. Journal of Plant Physiology, 144(3), 307–313. [Google Scholar]
31. Dubois, M., Gilles, K. A., Hamilton, J. K., Rebers, P. A., Smith, F. (1956). Colorimetric method for determination of sugars and related substances. Analytical Chemistry, 28(3), 350–356. [Google Scholar]
32. Lourenço, S. O., Barbarino, E., Lavín, P. L., Lanfer Marquez, U. M., Aidar, E. (2004). Distribution of intracellular nitrogen in marine microalgae: Calculation of new nitrogen-to-protein conversion factors. European Journal of Phycology, 39(1), 17–32. [Google Scholar]
33. Bligh, E. G., Dyer, W. J. (1959). A rapid method of total lipid extraction and purification. Canadian Journal of Biochemistry and Physiology, 37(8), 911–917. [Google Scholar] [PubMed]
34. Reynolds, E. S. (1963). The use of Lead citrate at high Ph as an electron-opaque stain in electron microscopy. Journal of Cell Biology, 17(1), 208–212. [Google Scholar] [PubMed]
35. AOAC (2000). Official methods of analysis of the association of official analytical chemists. 17th ed.969.3 and 991.39 fatty acids in oils and fats preparation of methyl esters Boron tri fluoride-AOAC-IUPAC method CODEX-adopted-AOAC method, pp. 19–20. [Google Scholar]
36. Talebi, A. F., Tabatabaei, M., Chisti, Y. (2014). BiodieselAnalyzer: A user-friendly software for predicting the properties of prospective biodiesel. Biofuel Research Journal, 1(2), 55–57. [Google Scholar]
37. Daneshvar, E., Zarrinmehr, M. J., Koutra, E., Kornaros, M., Farhadian, O. et al. (2019). Sequential cultivation of microalgae in raw and recycled dairy wastewater: Microalgal growth, wastewater treatment and biochemical composition. Bioresource Technology, 273, 556–564. https://doi.org/10.1016/j.biortech.2018.11.059 [Google Scholar] [PubMed] [CrossRef]
38. Roopnarain, A., Gray, V. M., Sym, S. D. (2014). Phosphorus limitation and starvation effects on cell growth and lipid accumulation in Isochrysis galbana U4 for biodiesel production. Bioresource Technology, 156, 408–411. [Google Scholar] [PubMed]
39. Marchetti, A., Maldonado, M. T. (2016). Iron. In: Borowitzka, M., Beardall, J., Raven, J. (Eds.The physiology of microalgae. Developments in applied phycology, vol. 6. Cham: Springer. https://doi.org/10.1007/978-3-319-24945-2_11 [Google Scholar] [CrossRef]
40. Gorain, P. C., Bagchi, S. K., Mallick, N. (2013). Effects of calcium, magnesium and sodium chloride in enhancing lipid accumulation in two green microalgae. Environmental Technology, 34(13–14), 1887–1894. https://doi.org/10.1080/09593330.2013.812668 [Google Scholar] [PubMed] [CrossRef]
41. García-Galán, M. J., Gutiérrez, R., Uggetti, E., Matamoros, V., García, J. et al. (2018). Use of full-scale hybrid horizontal tubular photobioreactors to process agricultural runoff. Biosystems Engineering, 166(3), 138–149. https://doi.org/10.1016/j.biosystemseng.2017.11.016 [Google Scholar] [CrossRef]
42. Perez-Garcia, O., Escalante, F. M. E., de-Bashan, L. E., Bashan, Y. (2011). Heterotrophic cultures of microalgae: Metabolism and potential products. Water Research, 45(1), 11–36. https://doi.org/10.1016/j.watres.2010.08.037 [Google Scholar] [PubMed] [CrossRef]
43. Díez-Montero, R., Belohlav, V., Ortiz, A., Uggetti, E., García-Galán, M. J. et al. (2020). Evaluation of daily and seasonal variations in a semi-closed photobioreactor for microalgae-based bioremediation of agricultural runoff at full-scale. Algal Research, 47, 101859. https://doi.org/10.1016/j.algal.2020.101859 [Google Scholar] [CrossRef]
44. Vazirzadeh, A., Jafarifard, K., Ajdari, A., Chisti, Y. (2022). Removal of nitrate and phosphate from simulated agricultural runoff water by Chlorella vulgaris. Science of the Total Environment, 802(3), 149988. https://doi.org/10.1016/j.scitotenv.2021.149988 [Google Scholar] [PubMed] [CrossRef]
45. Kumar Patel, A., Tseng, Y. S., Rani Singhania, R., Chen, C. W., Chang, J. S. et al. (2021). Novel application of microalgae platform for biodesalination process: A review. Bioresource Technology, 337, 125343. https://doi.org/10.1016/j.biortech.2021.125343 [Google Scholar] [CrossRef]
46. El-Sayed, A. B., El-Fouly, M. M., Abou El-Nour, E. A. A. (2010). Immobilized microalga Scenedesmus sp. for biological desalination of Red Sea water: I. Effect on growth. Nature and Science, 8(9), 69–76. [Google Scholar]
47. Abedi, S., Astaraei, F. R., Ghobadian, B., Tavakoli, O., Jalili, H. et al. (2019). Decoupling a novel Trichormus variabilis-Synechocystis sp. interaction to boost phycoremediation. Scientific Reports, 9(1), 2511. [Google Scholar] [PubMed]
48. Figler, A., B-Béres, V., Dobronoki, D., Márton, K., Nagy, S. A. et al. (2019). Salt tolerance and desalination abilities of nine common green microalgae isolates. Water, 11(12), 2527. [Google Scholar]
49. Ahamefule, C. S., Ugwuodo, C. J., Idike, P. O., Ogbonna, J. C. (2021). Application of photosynthetic microalgae in the direct desalination pretreatment of seawater. Water and Environment Journal, 35(2), 657–669. [Google Scholar]
50. Wang, L., Min, M., Li, Y., Chen, P., Chen, Y. et al. (2010). Cultivation of green algae Chlorella sp. in different wastewaters from municipal wastewater treatment plant. Applied Biochemistry and Biotechnology, 162(4), 1174–1186. https://doi.org/10.1007/s12010-009-8866-7 [Google Scholar] [PubMed] [CrossRef]
51. Ermis, H., Guven-Gulhan, U., Cakir, T., Altinbas, M. (2020). Effect of iron and magnesium addition on population dynamics and high value product of microalgae grown in anaerobic liquid digestate. Scientific Reports, 10(1), 1–12. [Google Scholar]
52. Ji, F., Zhou, Y., Pang, A., Ning, L., Rodgers, K. et al. (2015). Fed-batch cultivation of Desmodesmus sp. in anaerobic digestion wastewater for improved nutrient removal and biodiesel production. Bioresource Technology, 184(10), 116–122. [Google Scholar] [PubMed]
53. Saad, M. G., Shafik, H. M., Mekki, L., El-Kholy, R. (2018). Impact of different nitrogen concentrations on biomass productivity, lipid content and target fatty acids within Chlorella sp. and desmodesmus quadricaudatus to enhance biodiesel production. International Journal of Scientific & Technology Research, 7(1), 123–130. [Google Scholar]
54. Chen, Z., Shao, S., He, Y., Luo, Q., Zheng, M. et al. (2020). Nutrients removal from piggery wastewater coupled to lipid production by a newly isolated self-flocculating microalga Desmodesmus sp. PW1. Bioresource Technology, 302(8), 122806. [Google Scholar] [PubMed]
55. Shah, S., Sahoo, D., Mishra, G. (2023). Mixotrophic cultivation enhances lipid productivity and fatty acid profile towards efficient production of microalgae-based biofuel from Desmodesmus sp. DLK. Biofuels, 14(2), 211–222. [Google Scholar]
56. Morsi, H. H., El-Sheekh, M. M., Eladel, H., Al-Tuwaijri, M. M., El-Sabbagh, S. M. et al. (2023). Screening the pollution-tolerant Chlorococcum sp. (Chlorophyceae) grown in municipalwastewater for simultaneous nutrient removal and biodiesel production. Water, 15(9), 1723. https://doi.org/10.3390/w15091723 [Google Scholar] [CrossRef]
57. Antolın, G., Tinaut, F. V., Briceno, Y., Castano, V., Perez, C. et al. (2002). Optimisation of biodiesel production by sunflower oil transesterification. Bioresource Technology, 83(2), 111–114. [Google Scholar] [PubMed]
58. Soares, S., Rocha, F. R. (2018). Fast spectrophotometric determination of iodine value in biodiesel and vegetable oils. Journal of the Brazilian Chemical Society, 29, 1701–1706. [Google Scholar]
59. ASTM D6751-08 (2008). Standard specification for biodiesel fuel blend stock (B100) for middle distillate fuels. West Conshohocken, PA, USA: ASTM International. [Google Scholar]
60. EN 14214:2012+A2:2019 (2018). Liquid petroleum products—fatty acid methyl esters (FAME) for use in diesel engines and heating applications—requirements and test methods. Brussels, Belgium: European Committee for Standardization. [Google Scholar]
61. Deshmukh, S., Bala, K., Kumar, R. (2019). Selection of microalgae species based on their lipid content, fatty acid profile and apparent fuel properties for biodiesel production. Environmental Science Pollution Research, 26, 24462–24473. [Google Scholar] [PubMed]
Cite This Article
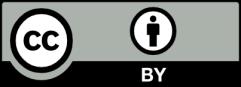
This work is licensed under a Creative Commons Attribution 4.0 International License , which permits unrestricted use, distribution, and reproduction in any medium, provided the original work is properly cited.