Open Access
REVIEW
Aluminum Toxicity: A Case Study on Tobacco (Nicotiana tabacum L.)
1 Department of Botany and Centre for Environmental Studies, Ege University, Izmir, 35100, Turkiye
2 Graduate School of Environmental Engineering, The University of Kitakyushu, Fukuoka, 808-0135, Japan
3 Department of Biology, Faculty of Science and Arts, Hatay Mustafa Kemal University, Hatay, 31060, Turkiye
4 Atta-ur-Rahman School of Applied Biosciences, National University of Sciences & Technology, Islamabad, 44000, Pakistan
5 Department of Biotechnology, Faculty of Science and Arts, Nigde Omer Halisdemir University, Nigde, 51240, Turkiye
6 Department of Molecular Biology and Genetics, Faculty of Science and Arts, University of Bilecik Seyh Edebali, Bilecik, 11230, Turkiye
7 Department of Environmental Science, Sri Pratap College, Cluster University Srinagar, Kashmir, 190008, India
8 Division of Environmental Sciences, Sher-e-Kashmir University of Agricultural Sciences and Technology, Kashmir, 190025, India
9 International Platform for Dryland Research and Education, Tottori University, Tottori, 680-0001, Japan
10 Department of Biology, Faculty of Science, Ankara University, Ankara, 06100, Turkiye
11 Department of Superior School Engineering, University of Almería, Almería, 04120, Spain
12 Department of Medical Pharmacology, Cerrahpasa Medicine Faculty, Istanbul University-Cerrahpasa, Istanbul, 34096, Turkiye
* Corresponding Authors: Munir Ozturk. Email: ; Bengu Turkyilmaz Unal. Email:
Phyton-International Journal of Experimental Botany 2023, 92(1), 165-192. https://doi.org/10.32604/phyton.2022.022038
Received 17 February 2022; Accepted 13 May 2022; Issue published 06 September 2022
Abstract
Aluminum is an abundant metal in the earth’s crust that turns out to be toxic in acidic environments. Many plants are affected by the presence of aluminum at the whole plant level, at the organ level, and at the cellular level. Tobacco as a cash crop (Nicotiana tabacum L.) is a widely cultivated plant worldwide and is also a good model organism for research. Although there are many articles on Al-phytotoxicity in the literature, reviews on a single species that are economically and scientifically important are limited. In this article, we not only provide the biology associated with tobacco Al-toxicity, but also some essential information regarding the effects of this metal on other plant species (even animals). This review provides information on aluminum localization and uptake process by different staining techniques, as well as the effects of its toxicity at different compartment levels and the physiological consequences derived from them. In addition, molecular studies in recent years have reported specific responses to Al toxicity, such as overexpression of various protective proteins. Besides, this review discusses data on various organelle-based responses, cell death, and other mechanisms, data on tobacco plants and other kingdoms relevant to these studies.Keywords
Nomenclature
AOX | Alternative oxidase |
DNA | Deoxyribonucleic acid |
EDTA | Ethylenediaminetetraacetic acid |
MPTP | Mitochondrial permeability transition pore |
MS | Murashige-Skoog |
MT | Microtubule |
NADPH | Nicotinamide adenine dinucleotide phosphate |
PAL | Phenylalanine ammonia lyase |
PCD | Programmed cell death |
POD | Peroxidase |
ROS | Reactive oxygen species |
VPE | Vacuolar processing enzyme |
Aluminum is an abundant metal in the earth’s crust. It is one of the mineral soil components and can be rendered free from mineral soil via lowered pH of the environment [1]. The release of metal occurs in the form of Al (III). Al (III) is toxic to plants and is counted as the most toxic form of aluminum [2,3]. Because acidic soils cover a great portion of the global arable lands, Al toxicity emerges to be a significant limitation in crop production [4,5]. Al has been reported to interfere with cell division quickly, damage the cell structure, decrease nutrient and water intake, and block root elongation and growth [6].
Generally, plants face this metal via their roots. Aluminum leads to biochemical/physiological/anatomical alterations in roots. It is widely accepted that the most pronounced impact of the Al-induced phytotoxicity is blockage of root growth. A heavy impact on the cellular membrane of root cells emerges just in the early minutes of the exposure [2,4]. The inhibition of root elongation has been extensively used as a trait for screening Al-tolerant cultivars [7,8].
The metal actively interferes with plant cell cellular and molecular events [9], such as cell growth [10,11]. For example, in the case of N. tabacum cells, a 50 micromolar dose of the metal reduces the post-treatment growth by 70%, while a 100 micromolar dose nearly eliminates growth [12]. In addition to growth inhibition, another cellular response against Al is callose (1,3-glucan) expression [13–15]. According to Horst et al. [14], callose expression is the most concrete sign of the genotypic toxicity of aluminum. Together with other toxic effects, the metal is also discovered to prevent tubulin polymerization, which promotes a delay in microtubular disassembly in the mitotic division of plant cells [16,17].
Another remarkable subject regarding the relation of Al with plants is to discover the mechanisms of tolerance. There are various ways to res to aluminum among crops [3]. The release of aluminum chelators (for instance, malate and oxalate) from plant roots to the environment is counted among known strategies plants utilize against aluminum. While this is an extracellular mechanism to cope with the metal, there are also intracellular routes to combat the metal. One of these intracellular routes is the chelation of Al with organic acids in the cytoplasm and the subsequent imprisonment into the vacuole [4,18].
Oxidative stress is a mechanism of Al-phytotoxicity [19,20], while improved antioxidative capacity is considered a tolerance mechanism [6]. Besides antioxidative capacity and chelation followed imprisonment into the vacuole, Al-tolerance can also be provided via the induction of Al-resistance genes (for instance, organic acid-associated genes and stress-responsive genes) [6].
It is also noteworthy to state that the metal cannot only connect to organic acids like citrate [4], but it is also able to link with adenosine triphosphate, nucleoside tri-phosphates (DNA and RNA), and phenolic chemicals in addition to some other cellular biochemicals [21,22]. This strong binding ability of Al to the biochemicals, as mentioned above, is suggested to impact the Al-stimulated toxicity.
Tobacco (N. tabacum) is a worldwide cultivated plant and is frequently utilized for scientific studies like Agrobacterium-mediated gene transformation [23]. As aforementioned, this species is too associated with Al phytotoxicity. In addition to cell growth blockage, tobacco experiences alterations in calcium (Ca) influx, cytoskeleton dynamics, phospholipase activities, cell wall structure and composition, cellular oxidative status, the structure of cell membrane and mitochondria, respiration, intake of nutrients, and cell elongation, metabolome, cell viability, the methylation pattern of DNA, root growth and structure, fresh and dry weight and in germination time as a response to the metal.
N. tabacum is a model organism that is both economically and scientifically important. Although the current data on aluminum toxicity, especially on molecular studies, are on mammals and bacteria, widespread molecular studies on tobacco plants also allow inter-kingdom comparisons. In this respect, the N. tabacum was chosen in this review, and it was tried to reveal the aluminum uptake, transport, toxicity and molecular level responses in comparison with the data obtained from other organisms in recent years.
Due to the lack of a radioactive tracer for Al, detecting this metal is hard. Thus, detection of the metal has to be based on staining techniques. The hematoxylin staining technique is a very simple way to detect aluminum. Its simplicity makes the technique one of the most efficacious techniques of Al detection [24]. Hematoxylin staining is utilized to screen Al-resistant plants [25,26]. Despite this, the technique has a defect, as well. Aluminum phosphate is more easily stained than other aluminum species [27]. Morin staining is widely preferred as a more sensitive tool [28–30]. Due to its sensitivity for Al detection (better than morin) and specific reactivity to all inorganic Al species, lumogallion is favored, additionally to hematoxylin in the detection of Al in cells [24,31]. By the lumogallion staining method, detection of trace doses of aluminum is possible as early as starting 15 min after the metal application [24].
In 1996, Vitorello et al. [30] discovered that aluminum is mostly positioned in a special area at the periphery cell, generally at the site of contact with neighboring cells. These sites are proposed to possibly match the growth sites in cell surface and volume [30]. Chang et al. [32] searched for the localization of aluminum. They exposed cells to aluminum and applied cellulase and pectolyase to produce spheroplasts. They found that 76% of the metal is connected to a specific site of the cell wall remaining in the spheroplasts. In addition, just 13% of Al was connected to the major portion of the cell wall. The amount of Al entering the cytoplasm was even less than the amount connected to the major portion of the cell wall. It was only 11%. As cell walls from intact cells subjected to aluminum were studied, the metal was connected to small pectic-polysaccharides. These polysaccharides are approximately 3–7 kDa. Aluminum is known to create electrostatic linkages, preferably with the ligands which donate oxygen, for instance, carboxylate and Pi groups. So, it is believed that aluminum connects to carboxylate groups on pectin. The linkage of the metal to the cell wall content is discovered to be stronger than that of EDTA, an effectual chelator of aluminum. Furthermore, it is found that the content of cell wall pectin arises in the course of aluminum exposure. Chang et al. [32] proposed that the metal might link to the pectin freshly synthesized along with the exposure.
Another issue of interest was “Why is aluminum linked with a specific site of pectin that stays in the cell wall even following cellulose and pectolyase digestion?” Chang et al. [32] described the issue as follows: the linkage of aluminum to pectin in the cell wall could render it resistant to enzyme digestion, or the association of aluminum with the fresh pectin might take place on (or close to) the membrane surface and the pectin locating at the innermost part of the cell walls could be less prone to be digested via exogenous enzymes and stay in the spheroplasts [32].
In 2006, Tanoi et al. [24] reported that Al does not accumulate in the BY-2 cells whose cell membrane is not harmed, while it does accumulate in the nucleus, cell membrane, and other organelles in the case cell membrane is harmed. Therefore, it is proposed that Al accumulates in the organelles following plasma membrane damage [24]. According to Tanoi et al. [24], the metal sorely accumulates in the “organelles” and “cell membrane and wall” of BY-2 cells while no important disparity occurs in the Al content between “organelles” and “cell membrane and wall.” Further, dispersion of the metal on the cell membrane demonstrated not to be uniform. Based on these, it is suggested that there are some specific Al-binding sites on the cell membrane where Al cytotoxicity might be triggered [24].
Al binding to cells is suggested to be more stable in the presence of Fe2+ than in the absence of Fe2+ [33]. In case a combination of iron sulfate and aluminum chloride salts was applied in a modified MS medium, which lacks phosphorous and EDTA, tobacco cells showed a remarkable rise in the cellular concentrations of both metals and were able to be stained by hematoxylin. Moreover, nuclei staining was particularly strong [34].
The length of exposure time, dose, and growth phase determine aluminum uptake [35]. The level of the uptake is a determining factor of Al cytotoxicity [24,36,37].
Uptake of aluminum only occurs at the logarithmic phase. Cells at the stationary phase do not uptake aluminum and are tolerant to this metal [35,37]. Vitorello et al. [38] used actively growing tobacco BY-2 cells to investigate the short-term uptake of aluminum. These researchers applied 20 micromolar aluminum chloride to the cells and found that uptake into cells occurred within 5 min. The initial uptake rate was 16 nmol Al (106 cells)–1 h–1 [30].
In 1999, Vitorello et al. [38] studied the impact of brefeldin A (BFA), which inhibits Golgi-mediated secretion and preincubation in a minimal medium, on the ability of tobacco cells to uptake aluminum. They found that 1–3 h preincubation in a minimal medium leads tobacco cells to lose their ability of Al-uptake, and it limits subsequent growth in a culture medium. The minimal medium triggered stop of Al-uptake is probably depressed or arrested cell growth. In the case of brefeldin A application before and after the incubation in a minimal medium, cells continued to take up aluminum. Sustained uptake was found to be dose-dependent and reversible. A BFA-responsive secretion pathway is essential for treatment-triggered depression in aluminum uptake via actively growing N. tabacum cells resulting in 5–10 micromolar BFA in response near to maximum [38].
Vitorello et al. [38] suggested a simple mechanism to describe the impact of BFA. Here is their suggestion: A growing plant needs transportation of Golgi-originated contents (proteins, polysaccharides) to specialized membrane sites. These contents are utilized to modify the cell membrane or are connected to the cell wall. Growth arrest induced via an alteration in medium results in differences in membrane structure and dynamics and at least partial disassembly of secretion and endocytotic pathways. Via acting on vesicular traffic, BFA treatment interrupts quick disassembly/assembly of cell-surface-associated structures, playing a role in nutrition-related signals. Thus, the plant cell connects to Al using surface structures, followed by Al uptake into the sites where growth is actively engaged [38]. Because iron is a part of nutrient media, the role of iron in Al-stimulated toxicity is questioned. It is found that iron is inevitable for Al-induced toxicity and cell death in tobacco. So, this part of the article is attributed to the role of iron in Al-stimulated growth inhibition, callose expression, accumulation of aluminum, and Al-triggered membrane-related problems of tobacco cells.
To understand the role of medium components in aluminum toxicity, Yamamoto et al. [36,39] applied aluminum to tobacco cells in a modified Murashige-Skoog (MS) media. Among the chemicals of MS medium, phosphate and EDTA lowered the growth-inhibitory impact of aluminum. As opposed to this, FeSO4 was a requisite for aluminum toxicity [36,39]. Tobacco cells in the log phase exposed to a combination of iron sulfate and aluminum chloride salts in a modified MS medium, which lacks Pi and EDTA, exhibit inhibited growth. The cells exposed to just one salt (at doses up to 100 micromolar, respectively) do not display inhibited growth. It is proposed that aluminum and iron ions synergistically inhibit growth. The combined application of iron and aluminum also reduces the number of cells [34,37].
5 Impact on Al Accumulation and Membrane
In the case of a calcium medium, aluminum singly triggers the accumulation of Al on tobacco cells. However, it singly fails to disrupt the cell membrane and causes leakage of K ions (a precise sign of membrane dysfunction), while the insertion of Fe2+ to the cells that accumulated Al instantly stimulates peroxidation of lipids, K leakage, and the disruption of the cell membrane (or cell death). Fe2+ alone too is shown to induce lipid peroxidation. However, the extent of the reaction was less than the combinational application of aluminum and iron. Accumulation of aluminum is found to sensitize the cells to iron-mediated lipid peroxidation. It is proposed that the accumulation of Al to a certain dose is significant for iron-mediated lipid peroxidation, which ends up with the disruption of the cell membrane (or cell death) [33].
In nutrient media, aluminum accumulation appears to be dependent on aluminum-improved, Fe2+-mediated lipid peroxidation and takes place solely in the existence of iron in the media. This event could result in the synthesis of cellular components (or leakage), and aluminum might link to freshly produced ligands. Chang et al. [32] suggested that pectin is a candidate for such a ligand. In nutrient media, two metals together additionally lead to a rise in the amount of cell wall pectin. It is hypothesized that Al-boosted Fe2+-mediated lipid peroxidation could change pectin metabolism and trigger de novo pectin production.
Chang et al. [32] suggested a possible scenario of how aluminum connects to the freshly synthesized pectin. According to their proposal, there is no certain or exact description of why aluminum connects to freshly synthesized pectin instead to the pre-existing one. However, they put forward two hypotheses [32]. Their first hypothesis was based on the fact that the electro-static linkage of aluminum to oxygen donor ligands is inhibited by other cationic ions [40]. According to this hypothesis, aluminum connects to freshly synthesized pectin because carboxylic acid groups on pre-existing pectin are associated with other cationic ions; however, differently, freshly synthesized pectin is less associated with other ions, so more prone to connect to aluminum as compared to pre-existing pectin. The second hypothesis states that the chemical structure of fresh pectin is distinct from the pre-existing one in a way rendering the connection of aluminum to fresh pectin preferable over its linkage to the pre-existing one [32].
Aluminum singly can cause callose expression in a Ca medium. It alone cannot provide callose expression in a nutrient medium lacking iron. Hence, aluminum accumulates preferably in the Ca medium rather than the nutrient media [13]; accumulation of Al in cells might be essential for Al-triggered callose expression. Callose expression appears to be an initial answer to aluminum [33,41]. Additionally, it is hypothesized that aluminum-sourced callose expression happens even in cells in which the cell membrane is intact in appearance [33].
Oppositely, in the existence of Fe2+, aluminum boosts callose expression both in Ca and nutrient media. It is accepted that β-1,3-glucan synthase found in the cell membrane plays a role in the expression of callose [13] and is strictly dependent on Ca in vitro [42]. In the case of nutrient media, callose expression was boosted via Fe and altogether was dependent on both aluminum boosted peroxidation of lipids and intracellular Ca [32]. Therefore, it is believed that callose expression might begin via Ca influx originating from the rupture of the cell membrane. In the case of the Ca medium, the expression of callose boosted via Fe and altogether is also dependent on aluminum boosted peroxidation of lipids, which probably can be described using the same hypothesis [33,43].
It is noteworthy that digestion of callose in the cell wall materials gained from the aluminum-exposed cells by the agency of laminarinase does not lead to the discharge of aluminum. So, it is proposed that callose does not play a role in the binding or trapping of aluminum [13,32].
Blockage of callose synthase activity is observed in addition to arisen callose expression when aluminum and iron co-exist in the media. Based on the results of various experiments, it is proposed that the enhancement of callose production by Al and Fe together is due to the activation of callose synthase by an increase in the cytosolic Ca concentration, but not due to an increase in the specific activity of the enzyme itself during the Al treatment [13,32]. On the other hand, Sivaguru et al. [44] have hypothesized that the accumulation of callose at plasmodesmata results in blocked inter-cellular molecular trafficking, resulting in restricted root growth.
Iron ion (2+, 3+) in nutrient media triggers aluminum toxicity in tobacco cells. In nutrient media lacking iron, aluminum singly cannot induce cell death of tobacco cells. However, as it coexists with Fe2+ in the media, aluminum accumulation occurs concomitantly with the beginning of cell death [34,45,46].
Aluminum and Fe2+ co-existence results in cell shrinkage and internucleosomal DNA fragmentation. Moreover, extracellular Ca and endogenous proteinase are inevitable for the cell death process started via Al and Fe2+. Aluminum and iron appear to activate a cell death program similar to apoptosis. An aluminum and iron combination in a calcium-free media can partially boost lipid peroxidation and hardly drive tobacco cells to death. This demonstrates that Ca further induces lipid peroxidation boosted via aluminum and iron and is a requisite for cell death. It is proposed that most lipid peroxidation and cell death boosted via aluminum and iron are mediated using extracellular Ca and endogenous proteinase, which function downstream from lipid peroxidation [37,47].
7.1 Al-Sensitive TPC1 Calcium Channel
N. tabacum cells exposed to aluminum exhibit a quick superoxide production [48,49]. The metal-induced superoxide leads to the flux of Ca to the intracellular environment. However, great doses of aluminum (>5 mM) prevent aluminum-promoted (superoxide-mediated) Ca flux into the intracellular environment, demonstrating that large doses of aluminum inhibit Ca channels responsive to reactive oxygen species (ROS). The Ca influx is provided by the agency of a voltage-gated calcium channel, TPC1, the only aluminum responsive and ROS-sensitive calcium channel known to date [50,51]. According to Kadota et al. [52], N. tabacum BY-2 cells have two orthologs of Arabidopsis thaliana’s TPC1, which are designated as NtTPC1A and B, most probably functioning as elicitor-responsive calcium channels.
Hydrogen peroxide-stimulated Ca influx is also blocked via aluminum [50]. Additionally, the metal (up to 10 mM) does not display an inhibitive impact on the hypoosmolarity-promoted Ca influx [48]. Furthermore, the Ca influx stimulated by elicitor sourced from the cell wall of Magnaporthe grisea does not alter via aluminum insertion to the medium. This situation shows that Ca channels playing a role in cellular answers to hypoosmotic shock and Magnaporthe elicitor might be distinct from the ROS-responsive ones [53]. Although exposure to aluminum cannot prevent the influx of Ca stimulated by the cell wall elicitor, remarkable retardation in calcium influx is observed. The retardation might be a probable feature of an aluminum-dependent disturbance in calcium signaling in plants [51].
Lin et al. [51] examined the role of Al-responsive Ca influx activity in a ROS-associated stress response, namely in reply to salicylate. The team specifically selected salicylate because salicylate is the most active class of natural plant defense inducer, reportedly inducing the formation of ROS (superoxide and hydrogen peroxide) followed by Ca signaling [54,55]. Consequently, it was found that salicylate-originated Ca influx was blocked using aluminum in a dose-dependent manner [51]. The Ca signaling activation via salicylate is reported to be mediated by the action of ROS like superoxide [55] and peroxide [54], which are produced via extracellular peroxidase activity [48].
Aluminum chloride (AlCl3) is not the only metal possessing the ability to induce superoxide production. Various metal salts are known to trigger the quick production of superoxide [56]. Kawano et al. [57] compared the superoxide production abilities of various salts with different metal valencies. They worked with two trivalent (LaCl3 and GdCl3), two divalent (CaCl2 and MgCl2), and two monovalent salts (KCl and NaCl) on BY-2 cells. As a result, it was found that there is an intense association between metal valency and the amount of superoxide synthesis. As the valency of the inserted metals was arisen from monovalent to divalent and trivalent, the dose needed to produce the maximal level of superoxide synthesis was reduced. Moreover, it is too discovered that NADPH oxidase functions in the trivalent and divalent metal-stimulated formation of superoxide [57].
Similar to the trivalent as mentioned above metal salts, AlCl3 stimulated superoxide generation could be accepted to originate from NADPH oxidase activity in tobacco. In support of this, a study on Arabidopsis [58] suggested a salicylate-mediated loop managing NADPH-mediated superoxide production. In the suggested model,
1) Aluminum triggers NADPH oxidase
2) NADPH oxidase generates superoxide
3) Superoxide triggers activation of the TPC1 channel
4) TPC1 channel provides Ca influx
5) Ca influx triggers salicylic acid synthesis and accumulation
6) Salicylic acid stimulates NADPH oxidase expression
7) More NADPH oxidase, more superoxide, more Ca, more salicylate…
Taken together, we have created Fig. 1 as a summary of Al-induced Ca influx on tobacco cells.
Figure 1: Aluminum induced salicylic acid-mediated Ca influx
7.2 Vacuole-Mediated Cell Death
Vacuolar processing enzyme (VPE) is a cysteine protease that provides caspase-1 function in the plant. Despite VPE being similar to caspase-1 in terms of enzymatic functioning, its structural characteristics are distinct from caspases. In addition, this enzyme is positioned in the vacuole rather than cytosol, where caspases are found [59,60]. VPE is responsible for the execution of programmed cell death in plants. It achieves this mission via disrupting the vacuole, in case of seed development, biotic and abiotic stresses like heat shock. Cellular death induced via the collapse of the vacuole is unique to plants. On the contrary, mitochondria-associated cellular death is common in animals and plants [61–63].
In 2013, Kariya et al. [61] found a new mechanism of Al-phytotoxicity controlled via the activation of VPE and collapsing of vacuole that appears to cause disruption of cell membrane integrity and a lowered capability in growth. They observed that Ac-YVAD-CHO, an inhibitor of caspase-1, blocks the Al-triggered disruption of cell membrane integrity in BY-2 cells. It is proposed that a rise in VPE function might promote disruption of cell membrane integrity in Al-exposed cells. Further, the increase in PVE function is thought to play a stronger role in a lowered growth capability than the disruption of cell membrane integrity. The increment in VPE function appears to be a primary event, causing a lowered capability in growth and disruption of cell membrane integrity, which is found to be heavily associated with the collapse of the vacuole [61].
The increase in VPE function has been controlled transcriptionally [61]. Al causes enhancements in gene expression of VPEs in BY-2 cells. The expression levels of four VPE genes (NtVPE-1a, NtVPE-1b, NtVPE-2, NtVPE-3) were investigated following treatment without (control) and with 100 μM aluminum for 18 h. Real-time quantitative RT-PCR demonstrated that all the four VPE genes were more or less boosted over the control levels via the aluminum treatment. Particularly, expressions of NtVPE-1a and the NtVPE-1b were improved by 2.5-fold [61]. In a study done in 2018, Kariya et al. [64] created RNA interference (RNAi) lines of each of the VPEs (NtVPE1a, NtVPE1b, NtVPE2, NtVPE3) in BY-2 cells. The comparison between wild-type and the RNAi lines showed that both aluminum-boosted VPE function and aluminum-triggered cell death were importantly suppressed in the RNAi lines of VPE1 (dual suppressor of VPE1a and VPE1b), however not in the RNAi lines of VPE2 and that of VPE3. It is concluded that the upregulation of VPE1 gene expression and following improvement of VPE function under aluminum exposure leads to cell death in actively growing or elongating cells of tobacco [64].
7.3 Mitochondria-Mediated Cell Death
The plant’s mitochondrial pathway differs from the animal mitochondrial pathway in possessing a branch point at the level of ubiquinone into a special alternative route. This route is represented via a carrier called alternative oxidase (AOX). AOX reduces oxygen without producing energy. Thus, AOX, in turn, lowers the ROS burden [65,66]. If the ubiquinone pool is reduced, AOX is known to possess a prominent function in repressing oxidative stress.
Furthermore, AOX1 is known to be the most abundant transcript. Exposure of plant cells to respiratory inhibitors and abiotic stress leads to an increment in AOX transcript amounts. Moreover, improved protein expression is observed during aluminum stress [67]. Regulation of AOX function is complicated and controlled both transcriptionally and post-translationally [68]. ROS might stimulate AOX expression [69–71]. AOX amount and activity are low in unstressed plants. Distinctly, alternative respiration improves following different stimuli, particularly under stress conditions (low temperature, wounding, and plant diseases) [72]. AOX1 of tobacco cells (NtAOX1) is quickly stimulated as the cytochrome pathway is blocked via antimycin A [73].
Panda et al. [74] exposed tobacco cells to aluminum and isolated mitochondria to investigate stress-related changes. They observed that respiration was inhibited in state-III, state-IV, succinate-dependent, AOX pathway capacity, and cytochrome pathway capacity. An improvement in ROS production under state-III respiration was too seen because of aluminum [74]. The reduction in the AOX pathway capacity because of metal treatment may be the reason behind increased ROS (superoxide and hydrogen peroxide) formation [74,75].
According to respiratory blockage, a reduction in the mitochondrial ATP amount was also seen. The significant activity of the electron transport chain of mitochondria is to synthesize ATP. Because of a restriction in ATP synthesis or electron transport, leakage of an electron from the transport chain is probable, ending up with the formation of superoxide radicals [74]. In aluminum-treated intact tobacco cells, a large increment in ROS generation and a reduction in respiration and cellular ATP content are observed. Taken together, it is hypothesized that Al-sourced prevention of respiration reduces mitochondrial ATP content, which seriously influences the cellular bio-energetic situation and might signal for programmed cell death (PCD) under energy deficiency (Fig. 2) [74,76,77].
Figure 2: A scheme of potential pathways going to Al-stimulated mitochondrial PCD. Al creates an oxidative stress situation, resulting in an irreversible dysfunction of mitochondria and cell death in tobacco cells [74]
In animal cells, mitochondrial permeability transition pore (MPTP) functions for the permeability transition of the outer mitochondrial membrane. This pore functions in reply to Ca, ROS, and other apoptogenic factors [78]. When opened up, MPTP promotes leakage of 1.5 kDa out of mitochondria. As a result of the opening, the inner membrane potential (ΔΨm) collapses [79]. A similar existence of MPTP is demonstrated in various plant species [80–82]. Like animals, plant MPTP can also be stimulated via Ca and oxidative stress. When stimulated, MPTP renders cytochrome c and other matrix proteins free, which may ultimately end up with PCD. Plant PCD and animal apoptosis have some common properties [80,81,83]. Oxidative stress has been demonstrated to induce the MPTP opening indirectly and possibly via rising Ca levels [84]. The role of ROS has been well attributed to open MPTP [85,86].
In tobacco mitochondria, aluminum promotes the opening of MPTP, which leads to the collapse of ΔΨm. The MPTP opening stimulating collapse of ΔΨm is proposed to be an early event in aluminum cytotoxicity [74,77]. Additionally, cytochrome c release from mitochondria of metal exposed cells was observed with a significant MPTP opening. This indicates that metal-treated tobacco cells follow the animal model of apoptotic death. Metal-originated functioning of MPTP is dependent on calcium [74].
Aluminum exposure also results in the activation of proteases and severely lowers calcium intake in tobacco mitochondria. A severe reduction in calcium uptake suggests an increase in matrix volume sourced from depolarization of the inner mitochondrial membrane. Moreover, a strong correlation between calcium intake and inner membrane potential is found. For instance, an 8% increase in the ATP-dependent mitochondrial protease function is seen in mitochondria obtained from metal applied cells compared to control cells. This function is found to be lower in the case of a serine protease inhibitor (AEBSF) treatment. Another inhibitor, i.e., aspartate protease (pepstatin), fails to lower the protease activity [74].
In addition to the release of cytochrome c, aluminum is also suggested to promote nuclear fragmentation. Cells exposed to this metal display shrunk outer nuclei membrane and many electron-dense bodies. Metal-applied cells exhibit shrunk cytoplasmic regions with the generation of plasma membrane blebbing, whereas cell walls and plasma membrane remain in close contact with normal cells. The metal does not affect the number of mitochondria, but numerous vacuoles are seen in metal-exposed cells. Different from the number of mitochondria, the structure of mitochondria appears to be influenced by aluminum. Aluminum has been shown to induce structural abnormalities in mitochondria. These abnormalities include swelling of mitochondria with abnormal outer membrane architecture, breakage of cristae, presence of electron-dense particles, and sometimes rupture of the outer membrane, all of which are known as characteristics of apoptosis. It is hypothesized that the high-amplitude swelling of mitochondria is a result of the metal-triggered opening of MPTP [74].
Arisen CaCl2 levels (up to 20 mM), low temperature (4°C), and pre-chelation of the metal to citrate largely lowers aluminum intake of N. tabacum cells (by 75%–90%). Metal intake is only decreased by around 10% as the amount of Ca in the uptake solution is arisen from 0.2 to 2.0 mM. Despite this, the existence of 20 mM Ca in both pre-wash and uptake solutions critically lowered metal intake by 90%. Oppositely, the post-application of 20 mM Ca fails to influence cellular aluminum levels. Also, pre-chelation of the metal to 5 mM citrate dramatically lowers metal intake by 85%. Upon decreasing the temperature of the standard pre-wash and uptake solutions from 23°C to 4°C, metal intake is decreased by around 75% [30]. In addition to CaCl2, macronutrients (KNO3, NH4NO3, MgSO4) in nutrient media also interfere with aluminum toxicity in cultured tobacco cells [34].
Phosphate (Pi)-starved tobacco cells are more resistant against aluminum, and the resistance rises as total cellular phosphorus (P) amounts lower. They also accumulate less aluminum as compared to control cells. Both normal and phosphate-starved cells exposed to Al ions release the ions into the media. However, the extrusion rate is greater in phosphate-starved ones. Therefore, the aluminum tolerance that phosphate-starved cells possess is partly attributed to a reduction in the net accumulation originating from the metal efflux. The expression of extracellular proteins is improved in response to aluminum in both normal and phosphate-starved cells.
Moreover, most of these proteins are boosted via phosphate deprivation. So, Al and phosphate deprivation result in common alterations in protein expression. It is proposed that the proteins stimulated via both aluminum and phosphate deprivation might function for the mechanism of resistance against aluminum [39].
Ezaki et al. [28] worked on suspension-cultured cells of N. tabacum L. cv. Samsun to understand the act of phosphate-deprivation on aluminum toxicity. They exposed tobacco cells to phosphate-deprivation and aluminum (two-step treatment: phosphate deprivation + aluminum application). Following the exposure, a cDNA library was derived from the treated cells created, and four cDNA clones were separated from the library by differential screening. It was discovered that the expression of pAL 111 and pAL 142 clones are triggered both by phosphate deprivation and aluminum exposure. When the complete cDNA sequencing of these four clones was determined, pAL111 was found to be identical to the parA gene of N. tabacum, which is known as an auxin-regulated gene, and pAL142 was detected to be highly homologous to the parB gene of N. tabacum whose product has a glutathione S-transferase (GST, EC 2.5.1.18) function [28].
One of the mechanisms of aluminum cytotoxicity is the prevention of cell division because of the suppression of transcription [87,88], and it is believed that the cell cycle is interrupted at a specific phase. Reasonably, it is hypothesized that the pAL111 (parA) gene product is triggered in reply to aluminum toxicity to assist in the recovery of transcription and transit cell cycle from the stopped phase to the next phase [28]. Regarding pAL142, it is assumed that the gene product is a member of GSTs of tobacco and has a function in the conjugation of glutathione to the byproducts of Al treatment; e.g., peroxidated lipid membrane [28].
In addition to pAL111 and pAL142, a different clone pAL201, which is expressed in response to aluminum treatment + phosphate deprivation, was isolated later. The deduced amino acid sequence suggested that pAL201 encodes one of the moderately anionic peroxidases of tobacco [89]. These types of peroxidases are triggered strongly in wounded stem tissue of tobacco [90], playing a pivotal role in wound healing or suberization via creating a water-tight barrier over the wound [91] and appear to be related to the cell wall [89]. In isoelectric focusing experiments, moderately anionic isozymes are seen mainly in the cell wall fraction, but some are observed in the soluble fraction. The ones found in the cell wall fraction are induced by phosphate deprivation rather than Al treatment. Nevertheless, it is proposed that they have a role in recovering the plasma membrane disrupted by the metal. Additionally, the isozymes observed in the soluble fraction might be associated with removing metal-induced peroxides [89].
While maximum expression of pAL111 and pAL142 occurs, respectively, 10 and 20 h after aluminum exposure [28], the transcript level of pAL201 continues to increase during 30 h of the metal exposure. The metal influences expression of each gene distinctly, and the gene product of pAL201 is an end component of an Al-triggered signaling pathway. Furthermore, strong production of the protein of pAL201 could be necessary for tobacco cells to survive in the presence of aluminum [89].
In 1998, Yamamoto et al. [92] discovered that tobacco cells accumulate phenylpropanoids (caffeic acid and chlorogenic acid) in an L-phenylalanine ammonia-lyase (PAL) dependent manner on a phosphate deprived medium. Furthermore, it is demonstrated that these compounds act as antioxidative agents and can save the cells from lipid peroxidation and cell death originating from the combined application of Al and Fe2+. Therefore, it is concluded that one of the reasons behind the tolerance of phosphate-deprived tobacco cells against Fe2+ and Al exposure is the synthesis of cellular antioxidants such as phenylpropanoids [92].
In acidic soils, Al3+ is the major ion responsible for rhizotoxicity, but proton (H+) is also phytotoxic to some sensitive species [93,94]. Low pH (high H+ concentrations) contributes to the solubilization of Al3+ in soil media, affecting the possible phytotoxic impacts of the metal on plants. Just distinctly, low pH also represses the negative charge of the cell membrane surface and thus lowers Al-binding to the membrane surface [93,95]. In the studies done on Arabidopsis, it is discovered that the expression of a transcription factor named AtSTOP1 is so significant for aluminum and H+ tolerance. Arabidopsis stop1 mutant exhibits great elevation in the sensitivity towards Al3+ and H+ rhizotoxicity. The increase in the sensitivity is attributed to the ability of AtSTOP1 to provide the expression of a variety of genes, playing a crucial role in Al3+ and H+ resistance [96,97]. Two Al-related proteins whose expression is controlled by stop1 are the protein of ALS3 (Aluminum sensitive 3) [98] and a type of citrate-transporter MATE (multidrug and toxic compound extrusion). Although the contribution of MATE for Al-resistance in Arabidopsis is minor, it is essential for the tolerance against the metal [99]. In case MATE expression is repressed, an important elevation level is observed in the sensitivity of Arabidopsis against aluminum [100]. It is also noteworthy that, differently from Arabidopsis, MATE-mediated citrate excretion contributes to Al-resistance in some plants [99]. In the case of ALS3, issues are getting more interesting because the origin of plant ALS3 is a question mark. However, it is believed that this gene encodes for a prokaryote-type ATP-binding cassette transporter with a possible role in UDP-glucose transport [98].
In their study, Ohyama et al. [101] searched for the characterization of orthologous genes of AtSTOP1 in a variety of plant species, including N. tabacum. Ohyama team produced RNAi lines for NtSTOP1 (tobacco ortholog of AtSTOP1). RNAi lines were demonstrated to possess an aluminum/proton-sensitive phenotype. Repression of NtSTOP1 also resulted in transcriptional repression of NtALS3 and NtMATE genes, restricted root growth, and interruption of Al-induced citrate release from tobacco roots. The transcript level of NtMATE was correlated with the capacity to excrete citrate. In contrast, RNAi lines displayed no increment in the sensitivity to other rhizotoxic ions (Mn, La, and Cd). In conclusion, NtSTOP1 seems to transcriptionally regulate the tolerance against both H+ and Al in tobacco, and Al-triggered citrate release controlled via NtMATE and NtSTOP1 is an Al-resistance mechanism of tobacco [101]
14 NtGDI1, NtPox, parA and parB Genes
Saccharomyces cerevisiae and Arabidopsis thaliana are two eukaryotic model organisms much utilized to express genes originating from other species to eliminate the functions of genes of interest. Ezaki et al. [102] expressed some Al-associated tobacco genes in both model organisms.
In the case of A. thaliana, expression of parA (pAL111), parB (pAL142), NtPox (an anionic peroxidase, pAL201), and NtGDI1 confers a degree of resistance to Al-mediated root growth blockage [103]. However, among four genes, only NtGDI1 can confer tolerance against Al-originated prevention of yeast growth as these genes are expressed in S. cerevisiae. Compared to control, NtGDI1 transgenic yeast displays the same level of Al absorption while it excretes the metal faster. Based on this knowledge, it is proposed that the NtGDI1 gene is responsible for the excretion of aluminum ions following their uptake [102]. GDI proteins are known to control vesicular traffic of exocytosis and endocytosis [104,105]. It is also known that the Arabidopsis GDI gene, AtGDI1, can complement yeast GDI mutation [106]. Thus, it is not illogical to accept that expression of NtGDI1 might as well influence yeast secretory pathway and NtGDI1 gene product provides Al-tolerance in yeast by Al excretion via acting on vesicular transport mechanisms [102].
Specifically, parB differed from the other three gene expression studies with Arabidopsis. This gene exhibited improved tolerance against oxidative stress stimulated by diamide and provided resistance to copper and sodium, and resistance to Al [103]. Contrary to this, expression of parB in yeast does not provide tolerance against Al. The situation is the same for NtPox. So, the question emerges to be considered: “Why NtPox and parB confer Al tolerance in A. thaliana but not in S. cerevisiae?” Ezaki et al. [102,103] proposed two possible explanations for this condition. The first scenario is those plant proteins of these genes are not expressed in an active form in S. cerevisiae or that overexpression of these genes in S. cerevisiae has no impact on their enzymatic activities. According to the second scenario, S. cerevisiae and A. thaliana might differ in regulating oxidative stress pathways or anti-oxidant enzyme machinery stimulated in response to Al stress [102,103].
A system for studying the impacts of Al has been designed utilizing a pair of isogenic N. tabacum cell lines, named ALT301 and SL. The parental line (SL, wild-type Samsun cells) displays Al-toxicity symptoms similar to roots [77,107], while a derived cell line, ALT301, is Al-tolerant. ALT301 accumulates the metal and produces callose to the same extent as the parental line. Furthermore, it also produces less reactive oxygen and undergoes less cell death [77,108,109]. Suppression of ROS in ALT301 is related to its resistance to Al; for example, ALT301 also tolerates hydrogen peroxide. A reduction in cell growth capability is known as another outcome of aluminum exposure where ALT301 cells display less decline in growth ability relative to SL cells [110].
The isogenic resistant and sensitive lines assist in distinguishing between processes that lead to metal-sourced toxic symptoms and those that accompany them [12].
16 Respiration and AOX Pathway
Aluminum negatively influences respiration and ATP production more extensively in SL mitochondria than in ALT301 mitochondria. AOX pathway and CYT pathway capacities are lowered in SL cells during exposure to the metal [111]. The mitochondria of aluminum applied ALT301 cells reduce state-III respiration, state-IV respiration, and cytochrome path-way [110], while an increase in AOX pathway capacity is observed [110,111]. In ALT301 whole cells, aluminum exposure lowers total respiration capacities of AOX and cytochrome pathways as higher capacities of AOX pathway relative to total respiration and capacity of cytochrome pathway are observed, regardless of the presence/absence of aluminum [110].
Aluminum is shown to trigger increased production of AOX in ALT301 cells. This situation is well correlated with the higher capacities of the AOX pathway. AOX1 expression of ALT301 cells stays stable at high levels up to 12 h Al treatment while a reduction occurs in SL cells. Moreover, AOX1 expression is more prominent in ALT301 than in SL cells, regardless of the presence/absence of the metal. Such consistent AOX expression is proposed to possess an important function in the aluminum resistance of ALT301 mitochondria [110].
The metal triggers an elevation in mitochondrial ROS in SL cells. An apparent reduction in mitochondrial ROS is seen in ALT301 cells [111]. In the case of aluminum-originated ROS accumulation, with doses of 50 and 100 μM, no remarkable alteration occurs in H2O2 content in ALT301 cells, while higher increases can be seen in SL cells. Unlike hydrogen peroxide content, aluminum remarkably acts on both SL and ALT301. In reply to 50 and 100 μM doses of aluminum, the superoxide amount rises in SL respectively by 23% and 30% and decreases in ALT301 respectively by 18% and 40%, after 18 h of the exposure [110]. The increment in AOX pathway capacity in ALT301 might be responsible for the reduced level of ROS formation during aluminum stress [74,110]. Based on these observations, it is proposed that the aluminum resistance of ALT301 is based on AOX, which suppresses mitochondrial oxidative stress [110].
To investigate the function of AOX in aluminum toxicity, Panda et al. [110] overexpressed the NtAOX1 gene in SL lines and compared mutant characteristics with the properties of ALT301 and SL cells. They reached the following conclusions: it is demonstrated that aluminum cannot significantly affect the growth ability of NtAOX1 over-expressing cells. Al negatively influences state-III respiration and cytochrome pathways in NtAOX1 over-expressing mutants. The mutants showed the minimum reduction in state III respiration compared to SL and ALT301 cells. Unlike SL cells, similar to ALT301 cells, NtAOX1 over-expressing cells exhibit an increment in AOX pathway capacity. Even the observed increment is higher than the rise seen in ALT301 cells. Similar to SL cells, transformed mutants display no important difference in the capacity of the CYT pathway. NtAOX1 over-expressing mutants exhibit remarkable decreases in ROS in response to Al stress. In reply to 50 and 100 μM doses of aluminum, amounts of superoxide and hydrogen peroxide in these mutant cells decrease remarkably. So, over-expression of NtAOX1 effectively lowers the aluminum triggered ROS generation in tobacco cells. NtAOX1 over-expression confers resistance against aluminum-induced toxicity symptoms of tobacco cells, including growth inhibition, oxidative stress, and respiratory inhibition.
19 ROS and Antioxidative Response
Aluminum stimulates ROS formation (most likely, superoxide), enhancement of MnSOD gene expression, and loss of growth capability in tobacco cells. Based on experiments on ALT301 and SL cells, it is hypothesized that aluminum-induced superoxide generation is the primary event leading to metal-induced enhancement of manganese superoxide dismutase (MnSOD) gene expression loss of growth capability [77,109].
The metal causes elevation of gene expression of MnSOD in both cell lines, however, at a considerably higher rate in SL than ALT301. In addition, aluminum also boosts the enzymatic function of MnSOD in both cell lines to nearly the same level [109]. A higher rise in MnSOD gene expression in SL probably refers to a higher accumulation of superoxide in SL than ALT301. So, the aluminum-resistant phenotype of ALT301 does not originate from an increase in MnSOD gene expression. It is related to the cellular machinery that minimizes the accumulation of both ROS and lipid peroxides [77,109].
Large portions of cellular ascorbate (reduced form) and glutathione are lower in case of exposure to aluminum, hydrogen peroxide, Fe2+, and copper. ALT301 maintains higher doses of ASC and GSH than SL, which strongly proposes that antioxidants are responsible for aluminum and oxidative stress resistance. Furthermore, ascorbate pre-loading before aluminum application enhances the growth of SL cells after aluminum exposure. It is concluded that higher doses of ascorbate and glutathione in ALT301 appear to assist in the resistance of ALT301 against aluminum and other oxidants. Moreover, both ascorbate and glutathione protect tobacco cells mainly from aluminum-boosted lipid peroxides under growing conditions. As opposed to this, both ascorbate peroxidase and catalase activities are found to be equivalent in SL and ALT301 and did not alter in case of aluminum exposure. Thus, it is proposed that these enzymes do not play a role in the aluminum resistance mechanism of ALT301. Additionally, the tolerance mechanism of ALT301 is indicated to be not related to organic acid (specifical citrate) secretion and efflux of aluminum [108,109]. Taken together, the aluminum resistance mechanism of ALT301 is not due to organic acid secretion (especially citrate), an increase in MnSOD gene expression, and aluminum efflux, but due to higher contents of ASC and GSH, and the evolution of ROS seems to be a key factor on the aluminum cytotoxicity in tobacco cells.
Diacylglycerol (DAG) has a remarkable role in biological membranes’ structural organization and dynamics. It is essential for membrane fusion and fission processes [112,113]. Ca-stimulated fusion of phospholipid vesicles is improved by diacylglycerol in vitro. The fusion rate is enhanced with PC-PLC function within a certain range of activities. It is clear that the catalytic action of PLC producing DAG, not the mere presence of the enzyme molecule, was responsible for the effect on fusion [114,115]. Membrane fusion events occur in many physiological processes, like exocytosis, endocytosis, membrane biogenesis, and cell division [116].
In 2010, Pejchar et al. [116] demonstrated that aluminum application decreased diacylglycerol generation in BY-2 cells. When it is considered that DAG formation is possible via various routes, “Which route is responsible for reduced DAG formation?” The reduction in DAG in reply to aluminum exposure can occur in response to either negative regulation of enzymes leading to the generation of DAG (PC-PLC, PLD/PA-phosphatase pathway, and MAG-acyltransferase) or positive regulation of enzymes consuming DAG (DAG-lipase, DAG-kinase, and choline phosphotransferase). Pejchar team investigated PC-PLC, PLD/PA-phosphatase pathway, MAG-acyltransferase, DAG-lipase, DAG-kinase, and choline phosphotransferase activities to solve the mystery. They observed that reduction in DAG formation is sourced from Al-originated decrease in PC-PLC activity, but not because of changes in activities of MAG-acyltransferase, PLD/PA-phosphatase pathway, and DAG-consuming enzymes. However, in vitro PC-PLC activity is sensitive only to large doses of aluminum chloride, which either suggests that the metal lowers PC-PLC function indirectly or may reflect experimental conditions of the in vitro assay, which certainly differs from intracellular conditions. Alteration of the substrate leading to less availability due to the structural changes caused by aluminum binding must be considered a probable indirect inhibitory mechanism [116].
Aluminum application to pollen tubes of tobacco rapidly inhibits growth and DAG generation. The impact of the metal on DAG generation is dose-dependent. Growth inhibition is rescued as exogenous DAG is inserted into metal-exposed pollen tubes. Distinctly, phosphatidic acid (which can be produced from DAG by DAG-kinase) has a minor impact on metal-induced growth inhibition. These phenomena suggest a significant role for DAG/PC-PLC in aluminum toxicity. Neither DAG nor PA influences pollen tube growth when inserted without AlCl3 [116]. Lastly, it is noteworthy to assume that reducing diacylglycerol amount during Al exposure might influence the cellular activities associated with membrane fusion (such as membrane biosynthesis) and quickly restrict root growth [116].
PLD is the enzyme responsible for the major production of PA via phosphatidylcholine hydrolysis. In addition to PLD, PA is produced via diacylglycerol kinase phosphorylation of DAG formed by PC-PLC or PI-PLC. PLD is too recognized as a microtubule-associated protein (MAP), which connects to and co-localizes with plant microtubules (MTs) [117]. Its block-age stimulates the prevention of root growth, cell swelling, and disruption of cortical MTs in Arabidopsis [118], and its release from the cell membrane and partial depolymerization in BY-2 cells [119]. The most remarkable physiological result of aluminum toxicity is the stop of root growth and alterations in root morphology proposing a role of the root cytoskeleton as a target structure. The significant role of phospholipid degrading enzyme phospholipase D in the regulation of cytoskeleton remodeling in both animal and plant organisms is evident. Both the phospholipid pathway and the cytoskeleton are affected by aluminum. However, their relationship with aluminum stress remains to be explored [120].
Aluminum is detected to reduce in vivo generation of PA and prevented in vitro activity of phosphatidylinositol-4,5-bisphosphate (PIP2) dependent phospholipase D of BY-2 cells. It is further investigated if PA generation is affected by the stabilization of MTs by taxol and destabilization by oryzalin. Tobacco cells do not expose aluminum answer to a taxon by improved PA formation, while treatment with oryzalin has no impact. However, in the presence of aluminum, taxol had no activating effect on PA production, whereas PA formation is highly improved by oryzalin. So, aluminum and PLD interaction are modulated by MT dynamics. Despite this, the interaction of aluminum ions with PLD and MTs cannot be described simply via PLD activation by MTs destabilization. The interaction presumably covers another regulatory machinery, which is aluminum sensitive. Such a regulatory mechanism could be the blockage of PLD by tubulin molecules, as was reported for animal PLD. Conclusively, cytoskeleton and phospholipid signal transduction cooperate throughout the reply to aluminum stress [120].
CMTs play a central role in regulating cell division, expansion, and elongation [121,122]. Quick reorganization of MTs upon aluminum exposure is reported for tobacco cells [116]. The influence of aluminum on tobacco cells in terms of MTs differs between distinct growth phases. Cells at the logarithmic phase lose their structural integrity of phragmoplast and spindle MTs after exposure to the metal. 50 micromolar aluminum depolymerizes the spindle MTs (SMTs) and cortical MTs (CMTs) and triggers growth inhibition in logarithmic phase cells while stabilizing CMTs in stationary-phase cells. However, stationary-phase cells also exhibit depolymerization of CMTs with 100 micromolar doses of the metal. The metal was also discovered to promote a transient elevation in cytosolic Ca of log-phase cells. As opposed to this, not even a transient increase is seen in stationary-phase cells. Aluminum-stimulated increase in cytosolic Ca be the reason behind metal-stimulated progressive depolymerization of CMTs. There may be a threshold of aluminum dose that can effectively depolymerize CMTs or prevent the assembly of individual tubulin molecules into MTs. If there is a threshold, the threshold dose in logarithmic-phase cells appears smaller than in stationary-phase cells [41]. The metal-originated blockage of growth of log-phase cells starts after 6 h from the metal treatment. This timing fits well with the lack of prominent CMTs from this time point. Therefore, the metal-induced depolymerization of CMTs may play a critical role in metal-triggered blockage of growth. Conversely, in stationary-phase cells, after the metal exposure, there is no depolymerization of CMTs, and a considerable level of growth happens, proposing that the metal-stimulated depolymerization of CMTs takes place more specifically in actively growing log-phase cells [41].
23 Sugar Uptake, Cell Elongation, and Root Growth
Aluminum is known to induce inhibition of elongation of plants cells. Al-sourced blockage of cell elongation is partly attributed to preventing water intake [12,123,124]. Preservation of regular water intake and cellular osmolality is heavily determined by soluble sugars, amino acids, and organic and inorganic ions [125]. It has been indicated that aluminum could be negatively influenced by soluble sugar content, reducing sugars, total soluble carbohydrates, and total carbohydrates [12,126–128]. In contrast, no marked alteration could be observed in inorganic ions of tobacco. Oppositely, a reduction in internal soluble sugar amounts (mainly glucose, fructose, and sucrose) of tobacco cells is seen in response to the metal. Moreover, the fresh weight and cellular osmolality of tobacco cells are also radically influenced by the presence of aluminum. The metal triggers a remarkable reduction in fresh and dry weight gains though curiously, it does not reduce the increment in cell number. The decrease in the content of soluble sugars is so much that it plausibly accounts for the lowered osmolality [12].
Al-sourced reduction in cellular osmolality, soluble sugar content, and intake rates of sucrose and glucose sign for a lowered driving force for water intake of tobacco. However, it seems that the metal does not influence water flux directly. The amount of cytoplasmic free sugar reflects metabolism as well as intake. Additionally, Al exposure can, in theory, induce glycolysis or other catabolic routes. However, the high decrease in sugar intake rate is mainly attributed to the metal-mediated reduction of cytoplasmic sugar levels. CCCP, a protonophore, is one of sucrose transporter inhibitor. CCCP blocks sucrose intake remarkably, but the inhibitory impact of CCCP and Al are not additive, proposing that Al blocks sucrose intake using a CCCP-sensitive pathway. Aluminum-stimulated remarkable decrease in sucrose intake occurs before ROS increases. It is proposed that prevention of sucrose intake by aluminum is a primary event, leading to lowered osmolality and hence lowered water intake and blockage of cell elongation, however not for symptoms related to Al toxicity, including callose generation, excessive ROS, and cell death [12].
Sucrose enters plant cells via various routes, including (I) proton sucrose symporter; (II) cleavage of sucrose using apoplastic invertase followed by intake via hexose transporters; (III) intake by endocytosis [129,130]. As a plasma membrane-localized sucrose/proton symporter gene, the role of SUT1 in aluminum toxicity has been studied in tobacco [131,132]. The gene was suppressed and overexpressed in tobacco cells for some deep analysis. The over-expressing mutants possessed 2-fold of the NtSUT1 protein as the suppressed mutants included only 20% of the protein, relative to wild-type cells. The over-expression improved the growth capability of BY-2 cells relative to wild-type cells regardless of previous exposure to the metal. This improvement is thought to originate from the over-expression-induced rise of sucrose intake rates and is followed by an increase in soluble sugar contents. If Al is applied to the over-expressing mutants, sucrose intake is quickly and completely blocked via NtSUT1. This blockage of the transporter suggests that NtSUT1 is one of the likely target proteins of the metal in the cell membrane. The metal is also indicated to negatively act on the expression of NtSUT1 at RNA and protein levels in over-expressing mutant cells and particularly in wild-type cells.
Nevertheless, the over-expressing cell line is accepted as resistant to aluminum because of its better growth capability and improved sucrose intake rate in post-Al treatment culture (lacking the metal) relatively to wild-type cells. This situation is supported by much higher NtSUT1 transcripts in the over-expressing mutants relative to wild-type cells after 18 h of treatment without or with Al. Thus, overexpression of NtSUT1 confers aluminum resistance in tobacco cells [132].
In the case of the suppression of NtSUT1, a limited level of decrease was seen in the cell growth rate. According to this, it is concluded that in suppressed mutants, the other two routes of sucrose intake compensate for the deficiency of the proton sucrose symporter [132].
In 2017, a study from Kariya et al. [131] reported impacts of overexpression and suppression of NtSUT1 at root apex in whole tobacco seedlings in aluminum resistance. Kariya’s team produced two NtSUT1 over-expressing (OX11 and OX12) and two NtSUT1 suppressings (RNAi42 and RNAi51) tobacco derivatives. Al (25 and 50 μM) application drove all lines to reduce root elongation rate. Relatively to wild-type, suppression mutants displayed decreased root elongation in reply to 50 μM Al. As opposed to this, over-expression mutants performed importantly better root elongation than wild-type in the presence of 25 and 50 μM Al. Based on these observations, it is concluded that NtSUT1 positively acts on root growth in tobacco seedlings, both in the presence and omission of the metal, and overexpression of this gene leads to resistance against Al-induced inhibition of root elongation [131].
Kariya’s team measured soluble sugar amounts of the root apex of wild-type OX11 and RNAi51 in the omission and presence of aluminum. With the help of the experiments done in the omission of Al, it is discovered that NtSUT1 is a major player in the uptake of exogenous sucrose at the root apex. Further, NtSUT1-mediated rise in soluble sugar amounts was a determining factor for root growth. In the presence of the metal, a marked reduction in soluble sugar amounts to 49% (wild-type), 79% (OX11), and 33% (RNAi51) of their respective initial levels were detected. The least reduction was observed in OX11 [131].
Consequently, a strong positive association between root elongation rate, the transcript level of NtSUT1, and soluble sugar content at root apex, was discovered in the presence and omission of the metal. Based on this association, it is concluded that soluble sugar content at the root apex is a determining factor in root elongation and NtSUT1 is a primary agent managing soluble sugar amount by sucrose intake via the apoplastic pathway at the root apex. So, NtSUT1 functions to manage root growth [131].
Further, the team attempted to measure the impact of NtSUT1 expression level on Al-triggered cell death with the help of the FDA staining technique. Under aluminum stress, over-expressing and suppression mutants possessed higher and lower fluorescence intensity than the wild-type, respectively, at their root apex. These results indicated that overexpressing mutants were more resistant, and RNAi lines were more susceptible to aluminum-induced cell death. Therefore, NtSUT1 overexpression confers to resistance against aluminum-sourced cell death. It is also noteworthy that the level of fluorescence intensity at the root apex is sorely associated with root growth. According to these results, aluminum-mediated cell death at the root apex seems to be one of the primary events causing aluminum-stimulated inhibition of root elongation. Even more, aluminum sourced cell death and blockage of cell elongation appears to be controlled by the expression level of NtSUT1. Conclusively, more transcripts of NtSUT1 at the root apex, more cell survival, and root elongation under aluminum exposure [131].
In 2012, Kircher et al. [133] reported that sucrose also has an inter-organ signaling function to support primary root elongation in the light in young Arabidopsis seedlings. The authors concluded that sucrose (photoassimilate) transport to the root tip or an external sucrose supply in the dark is necessary and sufficient to regulate root elongation [133]. Based on these endpoints in Arabidopsis, Kariya et al. also accepted that sucrose in tobacco also possesses a signaling role ending up with the induction of root growth [131].
Taken together, NtSUT1 at the root apex supports root elongation via providing sucrose using the apoplastic pathway that functions as an osmolyte, a carbon source, and a signal to regulate root growth. Aluminum prevents NtSUT1 transportation activity, possibly by binding into aspartate and glutamate’s negatively charged amino acid residues [131]. This prevention results in sucrose deficiency in roots, leading to the death of root apex cells and blocked root growth. Moreover, over-expression of NtSUT1 at root apex confers resistance against aluminum-induced phytotoxic impacts, including the death of root apex cells, prevention of root elongation, and decreased sucrose intake [12,131,132].
24 Germination and Root Structure
Vardar et al. [134] tested in vitro impacts of aluminum on germination by exposing tobacco seeds to different metal doses changing from 50 to 200 μM. Regardless of the metal dose, aluminum did not alter the germination rate in comparison to the control. However, the germination time was delayed with rising Al concentrations. Furthermore, root growth is determined to be influenced by the exposure, particularly at 200 μM. The metal induces a marked thickening of the apical root part and stubby/stiff root tips. In addition to cracks on the root surface, different doses of Al prevent elongation and curvature of roots. Schiff’s reagent application to metal exposed plants reveals the localization of the metal boosted peroxidation of lipids in situ on root surface with high sensitivity. Rising doses of the metal (between 50–200 μM) progressively induce POD activity. 19.9%, 23.8%, 31.7% and 77.7% increase is observed for doses of 50, 100, 150 and 200 μM, respectively. Arise in POD function correlates with the extent of root growth reduction. Conclusively, tobacco root tips are sensitive to aluminum exposure, especially in vitro [134].
A glycerophosphodiesterase-like protein (NtGPDL) is found to be expressed in the moment of Al stress. When detached leaves from wild-type tobacco plants are exposed to the metal, NtGPDL transcripts are triggered within 6 h, and associated genomic loci are demethylated at CCGG sites within 1 h. It is demonstrated that CG sites in coding regions are selectively demethylated and that promoter regions are unmethylated regardless of the exposure [135]. A similar decrease in methylation and transcriptional activation of NtGPDL is also observed in the case of exposure to paraquat, an influential ROS generator. Nearly identical demethylation patterns as with aluminum application (featuring selective demethylation at CGs in exon regions) are seen in the case of paraquat exposure. Because the metal is known to form ROS, the observed demethylation may have been mediated through oxygen radicals [135]. The amount of m5C over total cytosine residues is 13.00 ± 0.87% in control, while it is 10.95 ± 0.67% in metal-applied samples. So, it appears that aluminum has a slightly lower level of total DNA methylation [135].
In conclusion, we can divide the topic of Al-relation with tobacco into two phases. The first phase is “The Siege and Resistance Phase” (Fig. 3). In this phase, tobacco cells newly meet with the metal (which sieges them) and activate some tolerance mechanism to overcome possible side impacts. As time passes, Al starts to strongly accumulate in the cells, which means the start of “The Fall Phase.” In “The Fall Phase” (Fig. 4), Al begins to destroy and weaken the defense mechanisms of tobacco and leads to toxicity which can end up with cellular death and other phytotoxicity symptoms.
Figure 3: The siege and resistance phase
Figure 4: The fall phase. (A) VPE protein controls a vacuolar PCD mechanism which Al activates; (B–D) Blockage of NtSUT1 function via Al leads to sucrose deficiency. Due to this deficiency, cell elongation and root growth are inhibited; (C) Arisen lipid peroxidation by Al results in Ca influx and endogenous peroxidase activity, which ultimately stimulate activation of an apoptosis-like cellular death; (E) Callose in high doses is thought to prevent plasmodesmata function. Al-sourced overproduction of callose can block tobacco plasmodesmata and depress inter-cellular trafficking. These negative impacts can result in root growth inhibition; (F) Al itself and Al boosted lipid peroxidation can increase ROS, driving tobacco cells to death. In addition, Al uptake and Al-boosted lipid peroxidation seem to create a loop that exacerbates Al accumulation and cytotoxicity; (G) Al activates a salicylic acid-mediated positive feedback loop that causes increased NADPH oxidase expression and function. Increased levels of NADPH oxidase activity give birth to rising levels of superoxide. Superoxide itself is a ROS and can lead to other types of ROS. Arisen level of ROS is a danger for plant cells and can lead to cell death. Moreover, superoxide is known to activate Ca influx via TPC1. Specifically, we want to emphasize that TPC1 is the only aluminum responsive calcium channel known to date; thus use of aluminum is suggested to be a handy tool for the examination of the involvement of distinct types of calcium channels in a variety of cellular answers [53]; (H) Al can trigger mitochondrial PCD mainly via slowing down respiration
There are various tolerance mechanisms against Al. However, these mechanisms can differ from one cultivar to another cultivar, such as in the case of ALT301 and SL. Comparative studies with ALT301 and SL cell lines provided considerable information regarding aluminum tolerance and toxicity and seemed to possess high potential for future research about the relation between aluminum and plant cells. Additional to ALT301, the reader may be interested in one more Al-resistant tobacco line named ALT401. ALT401 is a slightly resistant line accumulating Al to a similar degree to SL cells but depositing less callose than SL [108]. We want to summarize our understanding of disparities between SL and ALT301 lines in terms of Al-toxicity via Table 1.
Although citrate released into the growth medium is generally recognized as a mechanism of Al-resistance of plants, it was not the case for the ALT301 cell line. However, it is important to note that ALT301 studies remained at the cell level and could not reach the whole-plant level. Therefore, at the whole plant level, it may be possible to observe that the ALT301 variety equips citrate excretion as one of the major mechanisms of Al-resistance. Another reason why citrate secretion is not recognized as a major source of Al-resistance of ALT301 could be characteristics of the Samsun cultivar. ALT301 cells are derived from Samsun cells. Although the Xanthi cultivar is shown to equip citrate release as a mechanism of Al-tolerance [101], the Samsun cultivar (thus also ALT301 and SL) may not own this strategy as one of the major Al-toxicity prevention mechanisms. Thus, varietal differences (genetics-based) can create the basis of disparities seen in responses to Al.
In summary, the use of different varieties may lead to highly different results, and species-level emphasis could be insufficient, so varieties utilized in heavy metal research should be specifically emphasized. In addition to genetic background, the difference in the growth environment can refer to differences in Al-toxicity levels. For instance, phosphate deficient growth medium is demonstrated to refer to Al-tolerance.
In this review article, we summarize the Al-associated biology of N. tabacum species. Even though there is valuable data regarding Al’s relation with the tobacco plant, there is also a requirement for more research.
Funding Statement: The authors received no specific funding for this study.
Conflicts of Interest: The authors declare that they have no conflicts of interest to report regarding the present study.
References
1. Vitorello, V. A., Capaldi, F. R., Stefanuto, V. A. (2005). Recent advances in aluminum toxicity and resistance in higher plants. Brazilian Journal of Plant Physiology, 17(1), 129–143. DOI 10.1590/S1677-04202005000100011. [Google Scholar] [CrossRef]
2. Rout, G., Samantaray, S., Das, P. (2001). Aluminium toxicity in plants: A review. Agronomie, 21(1), 3–21. DOI 10.1051/agro:2001105. [Google Scholar] [CrossRef]
3. Sathyaseelan, N., Karthika, K. (2019). Aluminium toxicity in soil and plants. Har Dhara, 2(1), 15–19. [Google Scholar]
4. Singh, S., Tripathi, D. K., Singh, S., Sharma, S., Dubey, N. K. et al. (2017). Toxicity of aluminium on various levels of plant cells and organism: A review. Environmental and Experimental Botany, 137(2), 177–193. DOI 10.1016/j.envexpbot.2017.01.005. [Google Scholar] [CrossRef]
5. Rahman, R., Upadhyaya, H. (2021). Aluminium toxicity and its tolerance in plant: A review. Journal of Plant Biology, 64(2), 101–121. DOI 10.1007/s12374-020-09280-4. [Google Scholar] [CrossRef]
6. He, H., Li, Y., He, L. F. (2019). Aluminum toxicity and tolerance in Solanaceae plants. South African Journal of Botany, 123(25), 23–29. DOI 10.1016/j.sajb.2019.02.008. [Google Scholar] [CrossRef]
7. Dipierro, N., Mondelli, D., Paciolla, C., Brunetti, G., Dipierro, S. (2005). Changes in the ascorbate system in the response of pumpkin (Cucurbita pepo L.) roots to aluminium stress. Journal of Plant Physiology, 162(5), 529–536. DOI 10.1016/j.jplph.2004.06.008. [Google Scholar] [CrossRef]
8. Lu, H. L., Dong, G., Hua, H., Zhao, W. L., Li, J. Y. et al. (2020). Method for initially selecting Al-tolerant rice varieties based on the charge characteristics of their roots. Ecotoxicology and Environmental Safety, 187, 109813. DOI 10.1016/j.ecoenv.2019.109813. [Google Scholar] [CrossRef]
9. Kochian, L. V., Pineros, M. A., Hoekenga, O. A. (2005). The physiology, genetics and molecular biology of plant aluminum resistance and toxicity. Plant and Soil, 274(1), 175–195. DOI 10.1007/s11104-004-1158-7. [Google Scholar] [CrossRef]
10. Matsumoto, H. (2000). Cell biology of aluminum toxicity and tolerance in higher plants. International Review of Cytology, 200, 1–46. DOI 10.1016/S0074-7696(00)00001-2. [Google Scholar] [CrossRef]
11. Silva, I. R., Smyth, T. J., Moxley, D. F., Carter, T. E., Allen, N. S. et al. (2000). Aluminum accumulation at nuclei of cells in the root tip. Fluorescence detection using lumogallion and confocal laser scanning microscopy. Plant Physiology, 123(2), 543–552. DOI 10.1104/pp.123.2.543. [Google Scholar] [CrossRef]
12. Abdel-Basset, R., Ozuka, S., Demiral, T., Furuichi, T., Sawatani, I. et al. (2010). Aluminium reduces sugar uptake in tobacco cell cultures: A potential cause of inhibited elongation but not of toxicity. Journal of Experimental Botany, 61(6), 1597–1610. DOI 10.1093/jxb/erq027. [Google Scholar] [CrossRef]
13. Chang, Y. C., Yamamoto, Y., Matsumoto, H. (1999). Enhancement of callose production by a combination of aluminum and iron in suspension-cultured tobacco (Nicotiana tabacum) cells. Soil Science and Plant Nutrition, 45(2), 337–347. DOI 10.1080/00380768.1999.10409348. [Google Scholar] [CrossRef]
14. Horst, W., Püschel, A. K., Schmohl, N. (1997). Induction of callose formation is a sensitive marker for genotypic aluminium sensitivity in maize. Plant and Soil, 192(1), 23–30. DOI 10.1023/A:1004204120863. [Google Scholar] [CrossRef]
15. Larsen, P. B., Tai, C. Y., Kochian, L. V., Howell, S. H. (1996). Arabidopsis mutants with increased sensitivity to aluminum. Plant Physiology, 110(3), 743–751. DOI 10.1104/pp.110.3.743. [Google Scholar] [CrossRef]
16. Frantzios, G., Galatis, B., Apostolakos, P. (2000). Aluminium effects on microtubule organization in dividing root-tip cells of Triticum turgidum. I. Mitotic cells. New Phytologist, 145(2), 211–224. DOI 10.1046/j.1469-8137.2000.00580.x. [Google Scholar] [CrossRef]
17. Vardar, F., Ünal, M. (2007). Aluminum toxicity and resistance in higher plants. Advances in Molecular Biology, 1, 1–12. [Google Scholar]
18. Tsuchiya, Y., Nakamura, T., Izumi, Y., Okazaki, K., Shinano, T. et al. (2021). Physiological role of aerobic fermentation constitutively expressed in an aluminum-tolerant cell line of tobacco (Nicotiana tabacum). Plant and Cell Physiology, 62(9), 1460–1477. DOI 10.1093/pcp/pcab098. [Google Scholar] [CrossRef]
19. Tabaldi, L. A., Cargnelutti, D., Gonçalves, J. F., Pereira, L. B., Castro, G. Y. et al. (2009). Oxidative stress is an early symptom triggered by aluminum in Al-sensitive potato plantlets. Chemosphere, 76(10), 1402–1409. DOI 10.1016/j.chemosphere.2009.06.011. [Google Scholar] [CrossRef]
20. Shi, W. W. (2008). Influences of existing forms of aluminum in soil on the resistance of potato to infection of Soft Rot Bacteria. Journal of Anhui Agricultural Sciences, 19, 1. [Google Scholar]
21. Godbold, D., Jentschke, G. (1998). Aluminium accumulation in root cell walls coincides with inhibition of root growth but not with inhibition of magnesium uptake in Norway spruce. Physiologia Plantarum, 102(4), 553–560. DOI 10.1034/j.1399-3054.1998.1020410.x. [Google Scholar] [CrossRef]
22. Martin, R. B. (1992). Aluminium speciation in biology. In: Derek, J., Chadwick, Julie, Whelan (EdsAluminium in biology and medicine. pp. 5–25. England: Wiley. [Google Scholar]
23. Niedbała, G., Niazian, M., Sabbatini, P. (2021). Modeling agrobacterium-mediated gene transformation of tobacco (Nicotiana tabacum)—A model plant for gene transformation studies. Frontiers in Plant Science, 12, 695110. DOI 10.3389/fpls.2021.695110. [Google Scholar] [CrossRef]
24. Tanoi, K., Iikura, H., Nakanishi, T. M. (2006). Aluminum distribution and the condition of the plasma membrane in cultured tobacco cells detected using staining methods. Bioimages, 14, 19–26. DOI 10.11169/bioimages.14.19. [Google Scholar] [CrossRef]
25. Ryan, P. R., Ditomaso, J. M., Kochian, L. V. (1993). Aluminium toxicity in roots: An investigation of spatial sensitivity and the role of the root cap. Journal of Experimental Botany, 44(2), 437–446. DOI 10.1093/jxb/44.2.437. [Google Scholar] [CrossRef]
26. Singh, C. K., Singh, D., Sharma, S., Chandra, S., Taunk, J. et al. (2021). Morpho-physiological characterization coupled with expressional accord of exclusion mechanism in wild and cultivated lentil under aluminum stress. Protoplasma, 258(5), 1029–1045. DOI 10.1007/s00709-021-01619-z. [Google Scholar] [CrossRef]
27. Ownby, J. D. (1993). Mechanisms of reaction of hematoxylin with aluminium-treated wheat roots. Physiologia Plantarum, 87(3), 371–380. DOI 10.1111/j.1399-3054.1993.tb01744.x. [Google Scholar] [CrossRef]
28. Ezaki, B., Yamamoto, Y., Matsumoto, H. (1995). Cloning and sequencing of the cDNAs induced by aluminium treatment and Pi starvation in cultured tobacco cells. Physiologia Plantarum, 93(1), 11–18. DOI 10.1034/j.1399-3054.1995.930103.x. [Google Scholar] [CrossRef]
29. Vitorello, V. A., Haug, A. (1997). An aluminum-morin fluorescence assay for the visualization and determination of aluminum in cultured cells of Nicotiana tabacum L. cv. BY-2. Plant Science, 122(1), 35–42. DOI 10.1016/S0168-9452(96)04546-3. [Google Scholar] [CrossRef]
30. Vitorello, V. A., Haug, A. (1996). Short-term aluminium uptake by tobacco cells: Growth dependence and evidence for internalization in a discrete peripheral region. Physiologia Plantarum, 97(3), 536–544. DOI 10.1111/j.1399-3054.1996.tb00514.x. [Google Scholar] [CrossRef]
31. Kataoka, T., Mori, M., Nakanishi, T. M., Matsumoto, S., Uchiumi, A. (1997). Highly sensitive analytical method for aluminum movement in soybean root through lumogallion staining. Journal of Plant Research, 110(3), 305–309. [Google Scholar]
32. Chang, Y. C., Yamamoto, Y., Matsumoto, H. (1999). Accumulation of aluminium in the cell wall pectin in cultured tobacco (Nicotiana tabacum L.) cells treated with a combination of aluminium and iron. Plant, Cell & Environment, 22(8), 1009–1017. DOI 10.1046/j.1365-3040.1999.00467.x. [Google Scholar] [CrossRef]
33. Ikegawa, H., Yamamoto, Y., Matsumoto, H. (2000). Responses to aluminium of suspension-cultured tobacco cells in a simple calcium solution. Soil Science and Plant Nutrition, 46(2), 503–514. DOI 10.1080/00380768.2000.10408803. [Google Scholar] [CrossRef]
34. Ono, K., Yamamoto, Y., Hachiya, A., Matsumoto, H. (1995). Synergistic inhibition of growth by aluminum and iron of tobacco (Nicotiana tabacum L.) cells in suspension culture. Plant and Cell Physiology, 36(1), 115–125. DOI 10.1093/oxfordjournals.pcp.a078727. [Google Scholar] [CrossRef]
35. Yamamoto, Y., Rikiishi, S., Chang, Y. C., Ono, K., Kasai, M. et al. (1994). Quantitative estimation of aluminium toxicity in cultured tobacco cells: Correlation between aluminium uptake and growth inhibition. Plant and Cell Physiology, 35(4), 575–583. DOI 10.1093/oxfordjournals.pcp.a078632. [Google Scholar] [CrossRef]
36. Yamamoto, Y., Ono, K., Matsumoto, H. (1995). Determining factors for aluminium toxicity in cultured tobacco cells: Medium components and cellular growth conditions. In: Date, R. A., Grundon, N. J., Rayment, G. E., Probert, M. E. (Eds.Plant-soil interactions at low pH: Principles and management, pp. 359–361. Dordrecht: Springer. DOI 10.1007/978-94-011-0221-6_52. [Google Scholar] [CrossRef]
37. Yamamoto, Y. (2019). Aluminum toxicity in plant cells: Mechanisms of cell death and inhibition of cell elongation. Soil Science and Plant Nutrition, 65(1), 41–55. DOI 10.1080/00380768.2018.1553484. [Google Scholar] [CrossRef]
38. Vitorello, V., Haug, A. (1999). Capacity for aluminium uptake depends on brefeldin A-sensitive membrane traffic in tobacco (Nicotiana tabacum L. cv. BY-2) cells. Plant Cell Reports, 18(9), 733–736. DOI 10.1007/s002990050651. [Google Scholar] [CrossRef]
39. Yamamoto, Y., Mametsuka, K., Ezaki, B., Matsumoto, H. (1995). Aluminium tolerance caused by phosphate starvation in cultured tobacco cells. In: Date, R. A., Grundon, N. J., Rayment, G. E., Probert, M. E. (Eds.Plant-soil interactions at low pH: Principles and management, pp. 363–366. Dordrecht: Springer. DOI 10.1007/978-94-011-0221-6_53. [Google Scholar] [CrossRef]
40. Kinraide, T. B., Ryan, P. R., Kochian, L. V. (1992). Interactive effects of Al3+, H+, and other cations on root elongation considered in terms of cell-surface electrical potential. Plant Physiology, 99(4), 1461–1468. DOI 10.1104/pp.99.4.1461. [Google Scholar] [CrossRef]
41. Sivaguru, M., Yamamoto, Y., Matsumoto, H. (1999). Differential impacts of aluminium on microtubule organisation depends on growth phase in suspension—Cultured tobacco cells. Physiologia Plantarum, 107(1), 110–119. DOI 10.1034/j.1399-3054.1999.100115.x. [Google Scholar] [CrossRef]
42. Kauss, H. (1987). Some aspects of calcium-dependent regulation in plant metabolism. Annual Review of Plant Physiology, 38(1), 47–71. [Google Scholar]
43. Chandra, J., Keshavkant, S. (2021). Mechanisms underlying the phytotoxicity and genotoxicity of aluminum and their alleviation strategies: A review. Chemosphere, 278, 130384. DOI 10.1016/j.chemosphere.2021.130384. [Google Scholar] [CrossRef]
44. Sivaguru, M., Fujiwara, T., Šamaj, J., Baluška, F. E., Yang, Z. et al. (2000). Aluminum-induced 1→3-β-D-glucan inhibits cell-to-cell trafficking of molecules through plasmodesmata. A new mechanism of aluminum toxicity in plants. Plant Physiology, 124(3), 991–1006. DOI 10.1104/pp.124.3.991. [Google Scholar] [CrossRef]
45. Ikegawa, H., Yamamoto, Y., Matsumoto, H. (1998). Cell death caused by a combination of aluminum and iron in cultured tobacco cells. Physiologia Plantarum, 104(3), 474–478. DOI 10.1034/j.1399-3054.1998.1040324.x. [Google Scholar] [CrossRef]
46. Yamamoto, Y., Hachiya, A., Matsumoto, H. (1997). Oxidative damage to membranes by a combination of aluminum and iron in suspension-cultured tobacco cells. Plant and Cell Physiology, 38(12), 1333–1339. DOI 10.1093/oxfordjournals.pcp.a029126. [Google Scholar] [CrossRef]
47. Yamaguchi, Y., Yamamoto, Y., Matsumoto, H. (1999). Cell death process initiated by a combination of aluminium and iron in suspension-cultured tobacco cells (Nicotiana tabacumApoptosis-like cell death mediated by calcium and proteinase. Soil Science and Plant Nutrition, 45(3), 647–657. DOI 10.1080/00380768.1999.10415828. [Google Scholar] [CrossRef]
48. Kawano, T., Kadono, T., Furuichi, T., Muto, S., Lapeyrie, F. (2003). Aluminum-induced distortion in calcium signaling involving oxidative bursts and channel regulation in tobacco BY-2 cells. Biochemical and Biophysical Research Communications, 308(1), 35–42. [Google Scholar]
49. Chauhan, D. K., Yadav, V., Vaculik, M., Gassmann, W., Pike, S. et al. (2021). Aluminum toxicity and aluminum stress-induced physiological tolerance responses in higher plants. Critical Reviews in Biotechnology, 41(5), 715–730. DOI 10.1080/07388551.2021.1874282. [Google Scholar] [CrossRef]
50. Kawano, T., Kadono, T., Fumoto, K., Lapeyrie, F., Kuse, M. et al. (2004). Aluminum as a specific inhibitor of plant TPC1 Ca2+ channels. Biochemical and Biophysical Research Communications, 324(1), 40–45. DOI 10.1016/j.bbrc.2004.09.015. [Google Scholar] [CrossRef]
51. Lin, C., Yu, Y., Kadono, T., Iwata, M., Umemura, K. et al. (2005). Action of aluminum, novel TPC1-type channel inhibitor, against salicylate-induced and cold-shock-induced calcium influx in tobacco BY-2 cells. Biochemical and Biophysical Research Communications, 332(3), 823–830. DOI 10.1016/j.bbrc.2005.05.030. [Google Scholar] [CrossRef]
52. Kadota, Y., Furuichi, T., Ogasawara, Y., Goh, T., Higashi, K. et al. (2004). Identification of putative voltage-dependent Ca2+-permeable channels involved in cryptogein-induced Ca2+ transients and defense responses in tobacco BY-2 cells. Biochemical and Biophysical Research Communications, 317(3), 823–830. DOI 10.1016/j.bbrc.2004.03.114. [Google Scholar] [CrossRef]
53. Lin, C., Kawano, T. (2007). Effect of rare earth elements on cold-responsive calcium signaling in tobacco cells–A mini review. ITE Letters on Batteries, New Technologies & Medicine, 8(4), 414–420. [Google Scholar]
54. Kawano, T., Muto, S. (2000). Mechanism of peroxidase actions for salicylic acid-induced generation of active oxygen species and an increase in cytosolic calcium in tobacco cell suspension culture. Journal of Experimental Botany, 51(345), 685–693. DOI 10.1093/jexbot/51.345.685. [Google Scholar] [CrossRef]
55. Kawano, T., Sahashi, N., Takahashi, K., Uozumi, N., Muto, S. (1998). Salicylic acid induces extracellular superoxide generation followed by an increase in cytosolic calcium ion in tobacco suspension culture: The earliest events in salicylic acid signal transduction. Plant and Cell Physiology, 39(7), 721–730. DOI 10.1093/oxfordjournals.pcp.a029426. [Google Scholar] [CrossRef]
56. Río, L. A. D, Corpas, F. J., López-Huertas, E., Palma, J. M. (2018). Plant superoxide dismutases: Function under abiotic stress conditions. In: Gupta, D., Palma, J., Corpas, F. (Eds.Antioxidants and antioxidant enzymes in higher plants, pp. 1–26. Cham: Springer. DOI 10.1007/978-3-319-75088-0_1. [Google Scholar] [CrossRef]
57. Kawano, T., Kawano, N., Muto, S., Lapeyrie, F. (2001). Cation-induced superoxide generation in tobacco cell suspension culture is dependent on ion valence. Plant, Cell & Environment, 24(11), 1235–1241. DOI 10.1046/j.1365-3040.2001.00766.x. [Google Scholar] [CrossRef]
58. Kunihiro, S., Hiramatsu, T., Kawano, T. (2011). Involvement of salicylic acid signal transduction in aluminum-responsive oxidative burst in Arabidopsis thaliana cell suspension culture. Plant Signaling & Behavior, 6(5), 611–616. DOI 10.4161/psb.6.5.14895. [Google Scholar] [CrossRef]
59. Hatsugai, N., Kuroyanagi, M., Nishimura, M., Hara-Nishimura, I. (2006). A cellular suicide strategy of plants: Vacuole-mediated cell death. Apoptosis, 11(6), 905–911. [Google Scholar]
60. Yamada, K., Basak, A. K., Goto-Yamada, S., Tarnawska-Glatt, K., Hara-Nishimura, I. (2020). Vacuolar processing enzymes in the plant life cycle. New Phytologist, 226(1), 21–31. DOI 10.1111/nph.16306. [Google Scholar] [CrossRef]
61. Kariya, K., Demiral, T., Sasaki, T., Tsuchiya, Y., Turkan, I. et al. (2013). A novel mechanism of aluminium-induced cell death involving vacuolar processing enzyme and vacuolar collapse in tobacco cell line BY-2. Journal of Inorganic Biochemistry, 128, 196–201. DOI 10.1016/j.jinorgbio.2013.07.001. [Google Scholar] [CrossRef]
62. Li, Z., Yue, H., Xing, D. (2012). MAP Kinase 6-mediated activation of vacuolar processing enzyme modulates heat shock-induced programmed cell death in Arabidopsis. New Phytologist, 195(1), 85–96. DOI 10.1111/j.1469-8137.2012.04131.x. [Google Scholar] [CrossRef]
63. Reape, T. J., McCabe, P. F. (2008). Apoptotic-like programmed cell death in plants. New Phytologist, 180(1), 13–26. DOI 10.1111/j.1469-8137.2008.02549.x. [Google Scholar] [CrossRef]
64. Kariya, K., Tsuchiya, Y., Sasaki, T., Yamamoto, Y. (2018). Aluminium-induced cell death requires upregulation of NtVPE1 gene coding vacuolar processing enzyme in tobacco (Nicotiana tabacum L.). Journal of Inorganic Biochemistry, 181, 152–161. DOI 10.1016/j.jinorgbio.2017.09.008. [Google Scholar] [CrossRef]
65. Vanlerberghe, G. C., McIntosh, L. (1997). Alternative oxidase: From gene to function. Annual Review of Plant Biology, 48(1), 703–734. DOI 10.1146/annurev.arplant.48.1.703. [Google Scholar] [CrossRef]
66. Saha, B., Borovskii, G., Panda, S. K. (2016). Alternative oxidase and plant stress tolerance. Plant Signaling & Behavior, 11(12), e1256530. DOI /10.1080/15592324.2016.1256530. [Google Scholar]
67. Umbach, A. L., Fiorani, F., Siedow, J. N. (2005). Characterization of transformed Arabidopsis with altered alternative oxidase levels and analysis of effects on reactive oxygen species in tissue. Plant Physiology, 139(4), 1806–1820. DOI 10.1104/pp.105.070763. [Google Scholar] [CrossRef]
68. Affourtit, C., Albury, M. S., Crichton, P. G., Moore, A. L. (2002). Exploring the molecular nature of alternative oxidase regulation and catalysis. FEBS Letters, 510(3), 121–126. DOI 10.1016/S0014-5793(01)03261-6. [Google Scholar] [CrossRef]
69. Amirsadeghi, S., Robson, C. A., McDonald, A. E., Vanlerberghe, G. C. (2006). Changes in plant mitochondrial electron transport alter cellular levels of reactive oxygen species and susceptibility to cell death signaling molecules. Plant and Cell Physiology, 47(11), 1509–1519. DOI 10.1093/pcp/pcl016. [Google Scholar] [CrossRef]
70. Amirsadeghi, S., Robson, C. A., Vanlerberghe, G. C. (2007). The role of the mitochondrion in plant responses to biotic stress. Physiologia Plantarum, 129(1), 253–266. DOI 10.1111/j.1399-3054.2006.00775.x. [Google Scholar] [CrossRef]
71. Vanlerberghe, G. C., Mclntosh, L. (1996). Signals regulating the expression of the nuclear gene encoding alternative oxidase of plant mitochondria. Plant Physiology, 111(2), 589–595. DOI 10.1104/pp.111.2.589. [Google Scholar] [CrossRef]
72. Purvis, A. C., Shewfelt, R. L. (1993). Does the alternative pathway ameliorate chilling injury in sensitive plant tissues? Physiologia Plantarum, 88(4), 712–718. DOI 10.1111/j.1399-3054.1993.tb01393.x. [Google Scholar] [CrossRef]
73. Vanlerberghe, G. C., McIntosh, L. (1994). Mitochondrial electron transport regulation of nuclear gene expression (studies with the alternative oxidase gene of tobacco). Plant Physiology, 105(3), 867–874. DOI 10.1104/pp.105.3.867. [Google Scholar] [CrossRef]
74. Panda, S. K., Yamamoto, Y., Kondo, H., Matsumoto, H. (2008). Mitochondrial alterations related to programmed cell death in tobacco cells under aluminium stress. Comptes Rendus Biologies, 331(8), 597–610. DOI 10.1016/j.crvi.2008.04.008. [Google Scholar] [CrossRef]
75. van Breusegem, F., Slooten, L., Stassart, J. M., Moens, T., Botterman, J. et al. (1999). Overproduction of Arabidopsis thaliana FeSOD confers oxidative stress tolerance to transgenic maize. Plant and Cell Physiology, 40(5), 515–523. [Google Scholar]
76. Brown, G. C. (1999). Nitric oxide and mitochondrial respiration. Biochimica et Biophysica Acta (BBA)-Bioenergetics, 1411(2–3), 351–369. DOI 10.1016/S0005-2728(99)00025-0. [Google Scholar] [CrossRef]
77. Yamamoto, Y., Kobayashi, Y., Devi, S. R., Rikiishi, S., Matsumoto, H. (2002). Aluminum toxicity is associated with mitochondrial dysfunction and the production of reactive oxygen species in plant cells. Plant Physiology, 128(1), 63–72. DOI 10.1104/pp.010417. [Google Scholar] [CrossRef]
78. Cui, Y., Pan, M., Ma, J., Song, X., Cao, W. et al. (2021). Recent progress in the use of mitochondrial membrane permeability transition pore in mitochondrial dysfunction-related disease therapies. Molecular and Cellular Biochemistry, 476(1), 493–506. DOI 10.1007/s11010-020-03926-0. [Google Scholar] [CrossRef]
79. Kowaltowski, A. J., Castilho, R. F., Vercesi, A. E. (2001). Mitochondrial permeability transition and oxidative stress. FEBS Letters, 495(1–2), 12–15. DOI 10.1016/S0014-5793(01)02316-X. [Google Scholar] [CrossRef]
80. Arpagaus, S., Rawyler, A., Braendle, R. (2002). Occurrence and characteristics of the mitochondrial permeability transition in plants. Journal of Biological Chemistry, 277(3), 1780–1787. DOI 10.1074/jbc.M109416200. [Google Scholar] [CrossRef]
81. Tiwari, B. S., Belenghi, B., Levine, A. (2002). Oxidative stress increased respiration and generation of reactive oxygen species, resulting in ATP depletion, opening of mitochondrial permeability transition, and programmed cell death. Plant Physiology, 128(4), 1271–1281. DOI 10.1104/pp.010999. [Google Scholar] [CrossRef]
82. Virolainen, E., Blokhina, O., Fagerstedt, K. (2002). Ca2+-induced high amplitude swelling and cytochrome c release from wheat (Triticum aestivum L.) mitochondria under anoxic stress. Annals of Botany, 90(4), 509–516. DOI 10.1093/aob/mcf221. [Google Scholar] [CrossRef]
83. Zancani, M., Casolo, V., Petrussa, E., Peresson, C., Patui, S. et al. (2015). The permeability transition in plant mitochondria: The missing link. Frontiers in Plant Science, 6(95), 1120. DOI 10.3389/fpls.2015.01120. [Google Scholar] [CrossRef]
84. Xu, H., Heath, M. C. (1998). Role of calcium in signal transduction during the hypersensitive response caused by basidiospore-derived infection of the cowpea rust fungus. The Plant Cell, 10(4), 585–597. DOI 10.1105/tpc.10.4.585. [Google Scholar] [CrossRef]
85. Scorrano, L., Petronilli, V., Bernardi, P. (1997). On the voltage dependence of the mitochondrial permeability transition pore: A critical appraisal. Journal of Biological Chemistry, 272(19), 12295–12299. DOI 10.1074/jbc.272.19.12295. [Google Scholar] [CrossRef]
86. Rottenberg, H., Hoek, J. B. (2017). The path from mitochondrial ROS to aging runs through the mitochondrial permeability transition pore. Aging Cell, 16(5), 943–955. DOI 10.1111/acel.12650. [Google Scholar] [CrossRef]
87. Matsumoto, H., Morimura, S. (1980). Repressed template activity of chromatin of pea roots treated by aluminium. Plant and Cell Physiology, 21(6), 951–959. DOI 10.1093/oxfordjournals.pcp.a076083. [Google Scholar] [CrossRef]
88. Wallace, S., Anderson, I. (1984). Aluminum toxicity and DNA synthesis in wheat roots 1. Agronomy Journal, 76(1), 5–8. DOI 10.2134/agronj1984.00021962007600010002x. [Google Scholar] [CrossRef]
89. Ezaki, B., TsugUa, S., Matsumoto, H. (1996). Expression of a moderately anionic peroxidase is induced by aluminum treatment in tobacco cells: Possible involvement of peroxidase isozymes in aluminum ion stress. Physiologia Plantarum, 96(1), 21–28. DOI 10.1111/j.1399-3054.1996.tb00178.x. [Google Scholar] [CrossRef]
90. Lagrimini, L. M., Rothstein, S. (1987). Tissue specificity of tobacco peroxidase isozymes and their induction by wounding and tobacco mosaic virus infection. Plant Physiology, 84(2), 438–442. DOI 10.1104/pp.84.2.438. [Google Scholar] [CrossRef]
91. Espelie, K. E., Franceschi, V. R., Kolattukudy, P. (1986). Immunocytochemical localization and time course of appearance of an anionic peroxidase associated with suberization in wound-healing potato tuber tissue. Plant Physiology, 81(2), 487–492. DOI 10.1104/pp.81.2.487. [Google Scholar] [CrossRef]
92. Yamamoto, Y., Hachiya, A., Hamada, H., Matsumoto, H. (1998). Phenylpropanoids as a protectant of aluminum toxicity in cultured tobacco cells. Plant and Cell Physiology, 39(9), 950–957. DOI 10.1093/oxfordjournals.pcp.a029459. [Google Scholar] [CrossRef]
93. Kinraide, T. B. (1998). Three mechanisms for the calcium alleviation of mineral toxicities. Plant Physiology, 118(2), 513–520. DOI 10.1104/pp.118.2.513. [Google Scholar] [CrossRef]
94. Szurman-Zubrzycka, M., Chwiałkowska, K., Niemira, M., Kwaśniewski, M., Nawrot, M. et al. (2021). Aluminum or low pH-which is the bigger enemy of barley? Transcriptome analysis of barley root meristem under Al and low pH stress. Frontiers in Genetics, 12, 712. DOI 10.3389/fgene.2021.675260. [Google Scholar] [CrossRef]
95. Kinraide, T. (2003). Toxicity factors in acidic forest soils: Attempts to evaluate separately the toxic effects of excessive Al3+ and H+ and insufficient Ca2+ and Mg2+ upon root elongation. European Journal of Soil Science, 54(2), 323–333. DOI 10.1046/j.1365-2389.2003.00538.x. [Google Scholar] [CrossRef]
96. Iuchi, S., Koyama, H., Iuchi, A., Kobayashi, Y., Kitabayashi, S. et al. (2007). Zinc finger protein STOP1 is critical for proton tolerance in Arabidopsis and coregulates a key gene in aluminum tolerance. PNAS, 104(23), 9900–9905. DOI 10.1073/pnas.0700117104. [Google Scholar] [CrossRef]
97. Sawaki, Y., Iuchi, S., Kobayashi, Y., Kobayashi, Y., Ikka, T. et al. (2009). STOP1 regulates multiple genes that protect Arabidopsis from proton and aluminum toxicities. Plant Physiology, 150(1), 281–294. DOI 10.1104/pp.108.134700. [Google Scholar] [CrossRef]
98. Larsen, P. B., Geisler, M. J., Jones, C. A., Williams, K. M., Cancel, J. D. (2005). ALS3 encodes a phloem-localized ABC transporter-like protein that is required for aluminum tolerance in Arabidopsis. Plant Journal, 41(3), 353–363. DOI 10.1111/j.1365-313X.2004.02306.x. [Google Scholar] [CrossRef]
99. Magalhaes, J. V., Liu, J., Guimaraes, C. T., Lana, U. G., Alves, V. et al. (2007). A gene in the multidrug and toxic compound extrusion (MATE) family confers aluminum tolerance in sorghum. Nature Genetics, 39(9), 1156–1161. [Google Scholar]
100. Liu, J., Magalhaes, J. V., Shaff, J., Kochian, L. V. (2009). Aluminum-activated citrate and malate transporters from the MATE and ALMT families function independently to confer Arabidopsis aluminum tolerance. Plant Journal, 57(3), 389–399. DOI 10.1111/j.1365-313X.2008.03696.x. [Google Scholar] [CrossRef]
101. Ohyama, Y., Ito, H., Kobayashi, Y., Ikka, T., Morita, A. et al. (2013). Characterization of AtSTOP1 orthologous genes in tobacco and other plant species. Plant Physiology, 162(4), 1937–1946. DOI 10.1104/pp.113.218958. [Google Scholar] [CrossRef]
102. Ezaki, B., Sivaguru, M., Ezaki, Y., Matsumoto, H., Gardner, R. C. (1999). Acquisition of aluminum tolerance in Saccharomyces cerevisiae by expression of the BCB or NtGDI1 gene derived from plants. FEMS Microbiology Letters, 171(2), 81–87. DOI 10.1111/j.1574-6968.1999.tb13415.x. [Google Scholar] [CrossRef]
103. Ezaki, B., Gardner, R. C., Ezaki, Y., Matsumoto, H. (2000). Expression of aluminum-induced genes in transgenic Arabidopsis plants can ameliorate aluminum stress and/or oxidative stress. Plant Physiology, 122(3), 657–666. DOI 10.1104/pp.122.3.657. [Google Scholar] [CrossRef]
104. Matsui, Y., Kikuchi, A., Araki, S., Hata, Y., Kondo, J. et al. (1990). Molecular cloning and characterization of a novel type of regulatory protein (GDI) for smg p25A, a ras p21-like GTP-binding protein. Molecular and Cellular Biology, 10(8), 4116–4122. DOI 10.1128/mcb.10.8.4116-4122.1990. [Google Scholar] [CrossRef]
105. Willard, F. S., Kimple, R. J., Siderovski, D. P. (2004). Return of the GDI: The GoLoco motif in cell division. Annual Review of Biochemistry, 73(1), 925–951. DOI 10.1146/annurev.biochem.73.011303.073756. [Google Scholar] [CrossRef]
106. Ueda, T., Matsuda, N., Anai, T., Tsukaya, H., Uchimiya, H. et al. (1996). An Arabidopsis gene isolated by a novel method for detecting genetic interaction in yeast encodes the GDP dissociation inhibitor of Ara4 GTPase. Plant Cell, 8(11), 2079–2091. DOI 10.1105/tpc.8.11.2079. [Google Scholar] [CrossRef]
107. Yamamoto, Y., Kobayashi, Y., Devi, S. R., Rikiishi, S., Matsumoto, H. (2003). Oxidative stress triggered by aluminum in plant roots. In: Abe, J. (ed.Roots: The dynamic interface between plants and the earth, pp. 239–243. Dordrecht: Springer. DOI 10.1007/978-94-017-2923-9_23. [Google Scholar] [CrossRef]
108. Rama Devi, S., Yamamoto, Y., Matsumoto, H. (2001). Isolation of aluminum-tolerant cell lines of tobacco in a simple calcium medium and their responses to aluminum. Physiologia Plantarum, 112(3), 397–402. DOI 10.1034/j.1399-3054.2001.1120313.x. [Google Scholar] [CrossRef]
109. Devi, S. R., Yamamoto, Y., Matsumoto, H. (2003). An intracellular mechanism of aluminum tolerance associated with high antioxidant status in cultured tobacco cells. Journal of Inorganic Biochemistry, 97(1), 59–68. DOI 10.1016/S0162-0134(03)00182-X. [Google Scholar] [CrossRef]
110. Panda, S. K., Sahoo, L., Katsuhara, M., Matsumoto, H. (2013). Overexpression of alternative oxidase gene confers aluminum tolerance by altering the respiratory capacity and the response to oxidative stress in tobacco cells. Molecular Biotechnology, 54(2), 551–563. DOI 10.1007/s12033-012-9595-7. [Google Scholar] [CrossRef]
111. Panda, S., Yamamoto, Y., Sasaki, T., Matsumoto, H. (2005). Changes in mitochondrial redox status and regulation of AOX1 gene expression in tobacco cells under aluminium stress. Plant and Cell Physiology, 46, 179. DOI 10.14841/jspp.2005.0.179.0. [Google Scholar] [CrossRef]
112. Carrasco, S., Mérida, I. (2007). Diacylglycerol, when simplicity becomes complex. Trends in Biochemical Sciences, 32(1), 27–36. DOI 10.1016/j.tibs.2006.11.004. [Google Scholar] [CrossRef]
113. Haucke, V., di Paolo, G. (2007). Lipids and lipid modifications in the regulation of membrane traffic. Current Opinion in Cell Biology, 19(4), 426–435. DOI 10.1016/j.ceb.2007.06.003. [Google Scholar] [CrossRef]
114. Gómez-Fernández, J. C., Corbalán-García, S. (2007). Diacylglycerols, multivalent membrane modulators. Chemistry and Physics of Lipids, 148(1), 1–25. DOI 10.1016/j.chemphyslip.2007.04.003. [Google Scholar] [CrossRef]
115. Goñi, F. M., Alonso, A. (1999). Structure and functional properties of diacylglycerols in membranes. Progress in Lipid Research, 38(1), 1–48. [Google Scholar]
116. Pejchar, P., Potocký, M., Novotná, Z., Veselková, Š., Kocourková, D. et al. (2010). Aluminium ions inhibit the formation of diacylglycerol generated by phosphatidylcholine-hydrolysing phospholipase C in tobacco cells. New Phytologist, 188(1), 150–160. DOI 10.1111/j.1469-8137.2010.03349.x. [Google Scholar] [CrossRef]
117. Gardiner, J. C., Harper, J. D., Weerakoon, N. D., Collings, D. A., Ritchie, S. et al. (2001). A 90-kD phospholipase D from tobacco binds to microtubules and the plasma membrane. Plant Cell, 13(9), 2143–2158. DOI 10.1105/TPC.010114. [Google Scholar] [CrossRef]
118. Gardiner, J., Collings, D. A., Harper, J. D., Marc, J. (2003). The effects of the phospholipase D-antagonist 1-butanol on seedling development and microtubule organisation in Arabidopsis. Plant and Cell Physiology, 44(7), 687–696. DOI 10.1093/pcp/pcg095. [Google Scholar] [CrossRef]
119. Dhonukshe, P., Laxalt, A. M., Goedhart, J., Gadella, T. W., Munnik, T. (2003). Phospholipase D activation correlates with microtubule reorganization in living plant cells. Plant Cell, 15(11), 2666–2679. DOI 10.1105/tpc.014977. [Google Scholar] [CrossRef]
120. Pejchar, P., Pleskot, R., Schwarzerová, K., Martinec, J., Valentová, O. et al. (2008). Aluminum ions inhibit phospholipase D in a microtubule-dependent manner. Cell Biology International, 32(5), 554–556. DOI 10.1016/j.cellbi.2007.11.008. [Google Scholar] [CrossRef]
121. Cyr, R. J., Palevitz, B. A. (1995). Organization of cortical microtubules in plant cells. Current Opinion in Cell Biology, 7(1), 65–71. DOI 10.1016/0955-0674(95)80046-8. [Google Scholar] [CrossRef]
122. Hashimoto, T. (2015). Microtubules in plants. In: Arabidopsis book, vol. 13, e0179. DOI 10.1199/tab.0179. [Google Scholar] [CrossRef]
123. Rufyikiri, G., Dufey, J. E., Nootens, D., Delvaux, B. (2001). Effect of aluminium on bananas (Musa spp.) cultivated in acid solutions. II. Water and nutrient uptake. Fruits, 56(1), 5–16. DOI 10.1051/fruits:2001107. [Google Scholar] [CrossRef]
124. Yang, Z. B., Eticha, D., Albacete, A., Rao, I. M., Roitsch, T. et al. (2012). Physiological and molecular analysis of the interaction between aluminium toxicity and drought stress in common bean (Phaseolus vulgaris). Journal of Experimental Botany, 63(8), 3109–3125. DOI 10.1093/jxb/ers038. [Google Scholar] [CrossRef]
125. Sharp, R. E., Hsiao, T. C., Silk, W. K. (1990). Growth of the maize primary root at low water potentials: II. Role of growth and deposition of hexose and potassium in osmotic adjustment. Plant Physiology, 93(4), 1337–1346. DOI 10.1007/BF00201032. [Google Scholar] [CrossRef]
126. Graham, C. (2002). Nonstructural carbohydrate and prunasin composition of peach seedlings fertilized with different nitrogen sources and aluminum. Scientia Horticulturae, 94(1–2), 21–32. DOI 10.1016/S0304-4238(01)00345-4. [Google Scholar] [CrossRef]
127. Abdalla, M. M. (2008). Physiological aspects of aluminium toxicity on some metabolic and hormonal contents of Hordeum vulgare seedlings. Australian Journal of Basic and Applied Sciences, 2, 549–560. [Google Scholar]
128. Tabuchi, A., Kikui, S., Matsumoto, H. (2004). Differential effects of aluminium on osmotic potential and sugar accumulation in the root cells of Al-resistant and Al-sensitive wheat. Physiologia Plantarum, 120(1), 106–112. DOI 10.1111/j.0031-9317.2004.0206.x. [Google Scholar] [CrossRef]
129. Etxeberria, E., Baroja-Fernandez, E., Muñoz, F. J., Pozueta-Romero, J. (2005). Sucrose-inducible endocytosis as a mechanism for nutrient uptake in heterotrophic plant cells. Plant and Cell Physiology, 46(3), 474–481. DOI 10.1093/pcp/pci044. [Google Scholar] [CrossRef]
130. Sauer, N. (2007). Molecular physiology of higher plant sucrose transporters. FEBS Letters, 581(12), 2309–2317. DOI 10.1016/j.febslet.2007.03.048. [Google Scholar] [CrossRef]
131. Kariya, K., Sameeullah, M., Sasaki, T., Yamamoto, Y. (2017). Overexpression of the sucrose transporter gene NtSUT1 alleviates aluminum-induced inhibition of root elongation in tobacco (Nicotiana tabacum L.). Soil Science and Plant Nutrition, 63(1), 45–54. DOI 10.1080/00380768.2017.1283646. [Google Scholar] [CrossRef]
132. Sameeullah, M., Sasaki, T., Yamamoto, Y. (2013). Sucrose transporter NtSUT1 confers aluminum tolerance on cultured cells of tobacco (Nicotiana tabacum L.). Soil Science and Plant Nutrition, 59(5), 756–770. DOI 10.1080/00380768.2013.830230. [Google Scholar] [CrossRef]
133. Kircher, S., Schopfer, P. (2012). Photosynthetic sucrose acts as cotyledon-derived long-distance signal to control root growth during early seedling development in Arabidopsis. PNAS, 109(28), 11217–11221. DOI 10.1073/pnas.120374610. [Google Scholar] [CrossRef]
134. Vardar, F., Arican, E., Gozukirmizi, N. (2006). Effects of aluminum on in vitro root growth and seed germination of tobacco (Nicotiana tabacum L.). Advances in Food Sciences, 28(2), 85–88. [Google Scholar]
135. Choi, C. S., Sano, H. (2007). Abiotic-stress induces demethylation and transcriptional activation of a gene encoding a glycerophosphodiesterase-like protein in tobacco plants. Molecular Genetics and Genomics, 277(5), 589–600. DOI 10.1007/s00438-007-0209-1. [Google Scholar] [CrossRef]
Cite This Article
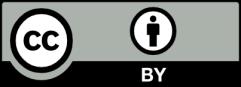
This work is licensed under a Creative Commons Attribution 4.0 International License , which permits unrestricted use, distribution, and reproduction in any medium, provided the original work is properly cited.