Open Access
ARTICLE
GhSCL4 Acts as a Positive Regulator in Both Transgenic Arabidopsis and Cotton during Salt Stress
1
Plant Genomics & Molecular Improvement of Colored Fiber Lab, College of Life Sciences and Medicine, Zhejiang Sci-Tech
University, Hangzhou, 310018, China
2
China Agricultural University, Beijing, 100193, China
* Corresponding Author: Yanyan Zhao. Email:
(This article belongs to the Special Issue: Symbiotic Associations for Nutrients Management and Complexes Formation for Better Agricultural Crops Productivity under Biotic and Abiotic Stresses)
Phyton-International Journal of Experimental Botany 2023, 92(1), 1-15. https://doi.org/10.32604/phyton.2022.022384
Received 08 March 2022; Accepted 13 April 2022; Issue published 06 September 2022
Abstract
GRAS transcription factors play important roles in plant abiotic stress response, but their characteristics and functions in cotton have not been fully investigated. A cotton SCL4/7 subgroup gene in the GRAS family, GhSCL4, was found to be induced by NaCl treatments. Nuclear localization and transactivation activity of GhSCL4 indicate its potential role in transcriptional regulation. Transgenic Arabidopsis thaliana over-expressing GhSCL4 showed enhanced resistance to salt and osmotic stress. What’s more, the transcript levels of salt stress-induced genes (AtNHX1 and AtSOS1) and oxidation-related genes (AtAPX3 and AtCSD2) were more highly induced in the GhSCL4 over-expression lines than in wild type after salt treatment. Furthermore, silencing of GhSCL4 resulted in reduced salt tolerance in cotton caused by reactive oxygen species (ROS) enrichment under salt treatment, and antioxidant enzyme activities were accordingly significantly reduced in GhSLC4-silenced lines. These results indicated that GhSCL4 enhances salt tolerance of cotton by detoxifying ROS. In addition, the transient expression assay confirmed an interactive relationship between GhSCL4 and GhCaM7, which indicated that salt tolerance conferred by GhSCL4 might be associated with salt-induced Ca2+/CaM7-mediated signaling. Taken together, GhSCL4 acts as a positive regulator in cotton during salt stress that is potentially useful for engineering salt-tolerant cotton.Keywords
Supplementary Material
Supplementary Material FileUpland cotton (Gossypium hirsutum) is widely cultivated as an important fiber and oil crop. Salinity and drought are two major environmental factors that limit cotton productivity worldwide [1,2]. As such, it is of the utmost importance to improve cotton yield, uncover the molecular mechanisms of cotton in response to stress, and breed cotton cultivars with enhanced salt and drought tolerance [3,4]. Being sessile organisms, plants cannot escape from abiotic stresses and have naturally developed a well-coordinated adaptive network to perceive stress signals and respond to various stresses [5–7].
Calcium (Ca2+) is a universal second messenger for the conveyance of signals and it induces series of molecular, biochemical and physiological reactions to confer resistance or tolerance in plants to adverse stresses [8,9]. Both salt and drought stresses elicit changes in cytosolic Ca2+ levels. This perturbation in cytosolic Ca2+ concentration is sensed by downstream Ca2+ sensor proteins. Calmodulins (CaMs) are the crucial Ca2+ sensors and they function in a calcium dependent manner and lack enzymatic activity [10–14]. Furthermore, Ca2+ induces conformational changes in CaM and affects their interactions with interacting partners and modulates target protein activity, which results in a cellular response that helps the plant overcome the unfavorable conditions [15,16].
Most, if not all, abiotic stresses are intimately linked to reactive oxygen species (ROS) metabolism [17]. In plants, the most commonly produced ROS include hydroxyl radical (·OH), superoxide (O2·–), ephemeral singlet oxygen (1O2) and hydrogen peroxide (H2O2) [18]. Extracellular ROS are mainly produced by membrane-dependent NADPH oxidases, and intracellular ROS are generated in multiple organelles. In response to abiotic stress, the production of ROS, such as superoxide radical and hydrogen peroxide, is enhanced [19]. ROS have dual functions in plants. On the one hand, low levels of ROS act as signal molecules, and on the other hand excess ROS causes oxidative stress that results in oxidative damage to plants (e.g., lipid peroxidation, reduced photosynthetic activity, and cell death) [20]. Consequently, for a plant to protect itself against diverse abiotic and biotic stresses, it must maintain moderate levels of ROS, which is principally accomplished by antioxidant systems [21]. Several enzymes, such as superoxide dismutase (SOD), catalase (CAT), and ascorbate peroxidase (APX), can remove the excess oxidants produced to avoid the damages associated with oxidative stress [22].
Transcription factors play crucial roles in linking stress-sensory pathways to many tolerance responses and the transcriptional regulation network is complex [2,3]. Many transcription factors, including WRKY [23], basic leucine zipper (bZIP) [24], APETALA2/ETHYLENE RESPONSE FACTOR (AP2/ERF) [25], MYB [26,27], basic helix–loop–helix (bHLH) [28] and GRAS family genes [29], are differentially expressed in response to abiotic stress and play regulatory roles in stress response. Here, we focus on the functional characterization of the GRAS family in abiotic stress. The acronym GRAS is derived from its three initially identified members, GIBBERELLIN ACID INSENSITIVE (GAI), REPRESSOR of GA1 (RGA), and SCARECROW (SCR) [29]. Analysis of the sequences of GRAS proteins showed that they contain several conserved amino acid signatures in the C-terminus, including LHR I, VHIID, LHR II, PFYRE and SAW [30].
At least 33 GRAS protein family members have been identified in Arabidopsis, and they are divided into several sub-families, such as SCL9, SHR, PAT1, SCR, HAM, SCL4/7, Ls and DELLA [31]. Molecular genetic studies have demonstrated that GRAS proteins are involved in diverse biological processes in plants, such as root development, gibberellin signal transduction, phytochrome A signal transduction, and shoot apical meristem development [30,32]. Moreover, recent studies have reported the involvement of GRAS proteins in abiotic stress responses. For instance, the Arabidopsis GRAS protein SCL14 serves as a TGA–interacting protein and participates in the detoxification of harmful chemicals [33]. A wheat GRAS gene, TaSCL14, was reported to be a positive regulator of plant tolerance to photooxidative stress [34]. In rice, overexpression of OsGRAS23 confers drought and oxidative stress tolerance [35].
Despite the important regulatory roles of GRAS proteins in plant response to abiotic stress, the functional characterization of them in cotton are largely unknown. In this study, we report on the functional characterization of a GRAS family gene GhSCL4, and found that GhSCL4 acts as a positive regulator of salt stress response, which could be a potential candidate for genetic engineering in improving the salt stress tolerance in cotton.
2.1 Plant Materials and Growth Conditions
The accession ZM24 of upland cotton was used as the research material. Seedlings of ZM24 were grown hydroponically in half-strength Hoagland nutrient solution until the third true leaf expanded, and then they were exposed to 200 mM NaCl and 6% polyethylene glycol 6000 (PEG6000) solution between 0 and 24 h. The roots were harvested at 0, 3, 6, 12 and 24 h. Seeds of A. thaliana were surface-sterilized with 50% sodium hypochlorite for 4 min and then rinsed three times using sterile water. The seeds were sown on solidified Murashige and Skoog (MS) medium and then vernalized at 4°C for two days in the dark before being transferred to grow at 22°C.
2.2 Quantitative Real-Time PCR
Total RNAs were isolated from Arabidopsis or cotton seedlings using RNAprep Pure Plant Kit (Tiangen, Beijing, China). Total RNA (1 μg) was reverse transcribed into cDNA using the MonScript™ RTIII kit (Monad). Gene-specific primers are listed in Supplementary Table S1. The housekeeping genes were G. hirsutum histone3 (GhHis3, Gh_D03G0370) and A. thaliana actin2 (AtActin2, At3G18780). Quantitative real-time PCR (qRT-PCR) was performed using MonAmp™ SYBR® Green qPCR Mix and reactions were run in a Monad Selected q225 PCR system. Relative expression levels of genes were calculated by the 2−ΔΔCt method.
2.3 Subcellular Localization and Transactivation Assay of GhSCL4
For the subcellular localization analysis, GhSCL4 coding sequences (CDS) were ligated into the EcoRV site of the pEarlyGate101 vector to construct the 35S::GhSCL4-YFP vector [36]. The positive construct was then transformed into tobacco (Nicotiana benthamiana) cells. After 48 h of inoculation, the epidermal cells of the infiltrated tobacco leaves were visualized using a confocal microscope (OLYMPUS FV1200). For transactivation assay, the full-length CDS of GhSCL4 was inserted between the EcoR I and Sal I sites of the pGBKT7 vector. The recombined construct and inset-less vector pGBKT7 were both transformed into yeast strain AH109. Transformants were selected by growing on SD\-Trp medium at 30°C for two days and the surviving colonies were then transferred to both SD\-Trp and SD\-Trp\-His\-Ade medium to grow for three days. The primers used in these two assays are listed in Supplementary Table S1.
2.4 Transformation of GhSCL4 in Arabidopsis and Stress Tolerance Tests
The full-length coding sequence of GhSCL4 (Gh_D12G0611) was retrieved from the CottonGen database (https://www.cottongen.org/data/download) [37]. Subsequently, it was amplified using specific primers p2300-GhSCL4-F and p2300-GhSCL4-R (see Supplementary Table S1) and cloned between the Xba I and Kpn I sites of the pCAMBIA2300 vector to generate the 35S::GhSCL4 construct. The construct was transformed into wild-type Arabidopsis (Columbia ecotype) (for gene overexpression) by the Agrobacterium tumefaciens-mediated floral dip method. The salt and osmotic tolerance of GhSCL4 transgenic Arabidopsis during the germination period was tested on MS agar plates saturated with 150 mM NaCl and 400 mM mannitol, respectively. Plant growth was monitored based on cotyledon greening rates on the tenth day post-germination. To evaluate the salt tolerance of adult seedlings grown in soil, 3-week-old Arabidopsis plants were irrigated with 200 mM NaCl for two weeks. To observe the primary root length of plants, Arabidopsis seeds were grown on 1/2 MS medium supplemented with NaCl or mannitol for 10 d and then the root length was measured.
2.5 Construction of Tobacco Tattle Virus Vectors
The tobacco rattle virus (TRV) system was used for virus induced gene silencing (VIGS) as previously reported [38]. A nearly 200-bp fragment of each of the candidate genes GhSCL4 and GhCaM7 was inserted into TRV-based inset-less vector pYL156 to produce TRV::GhSCL4 and TRV::GhCaM7, respectively, for VIGS in cotton plants. Plants injected with the pYL156 vector (TRV::00) were used as controls. Plasmids containing the positive recombinant VIGS vectors were transformed into A. tumefaciens strain GV3101. The cotyledons of 7-day-old ZM24 cotton seedlings were inoculated with equal amounts of the Agrobacterium expressing the TRV vectors as previously described [39]. qRT-PCR was performed to detect the interference efficiency of GhSCL4/GhCaM7 approximately two weeks after infiltration, and empty vector agro-infiltrated cotton plantlets were the control. For the salt tolerance assay, control and GhSCL4/GhCaM7-silenced plants were irrigated with 300 mM NaCl solution when plants produced the third true leaf.
2.6 Measurements of the Antioxidant Enzyme Activities
To further detect the antioxidant enzyme activities in cotton plants exposed to salt treatment for 10 days, approximately 200 mg of fresh leaf materials were collected and ground using 2 ml of pre-chilled 50 mM phosphate buffer. Activities of superoxide dismutase (SOD), peroxidase (POD), and catalase (CAT) were performed as described previously [40].
2.7 Bimolecular Fluorescence Complementation Assays
The CDS of GhSCL4 was ligated into the pXY104 (cYFP) vector. The CDS of GhCaM7 was cloned into the pXY106 (nYFP) vector [41]. The primers used in this assay are listed in Supplementary Table S1. The recombinant constructs were introduced into Agrobacterium strain GV3101. Agrobacterium cells were collected and resuspended in infiltration medium to an OD600 of 1.2. Agrobacterium cells containing nYFP and cYFP fusion proteins were then mixed at a ratio of 1:1 and infiltrated into tobacco leaves. After 48 h of inoculation, the infiltrated leaves were imaged using a confocal microscope (OLYMPUS FV1200).
2.8 Firefly Luciferase Complementation Imaging Assay
The CDS of GhSCL4 and GhCaM7 were ligated into the pCAMBIA1300-nLuc and pCAMBIA1300-cLuc vectors, respectively [42]. Agrobacterium cells containing the indicated constructs were collected and resuspended to a final concentration of OD600 = 0.5. Bacterial suspensions were infiltrated into leaves of N. benthamiana plants and then placed at 23°C for 48 h in the dark. Subsequently, plants were incubated in an artificial growth chamber (25°C, 16 h light/8 h dark photoperiod) before luciferase (LUC) activity was measured.
3.1 GhSCL4 is Induced by Abiotic Stresses and Has Transactivation Activity
To select the GRAS family genes involved in salt stress, the raw data of RNA-seq was download from NCBI Sequence Read Archive (SRA: PRJNA248163). A salt-induced SCL4/7 subgroup gene in cotton, namely GhSCL4 (Gh_D12G0611), was identified from the RNA-seq data of salt-treated cotton (Fig. 1A). Subsequently, the expression level of this gene was confirmed by qRT-PCR, and the result showed that the amounts of transcripts of GhSCL4 were higher than that in H2O-treated controls, especially after 3 and 6 h of salt treatment (Fig. 1B). In addition, the transient expression assay using N. benthamiana cells showed that the GhSCL4-YFP fusion protein accumulated mainly in the nucleus (Fig. 1C). Furthermore, GhSCL4 was cloned into the pGBKT7 vector and expressed in the AH109 yeast cells to confirm its transactivation activity. As shown in Fig. 1D, only transformants expressing the pGBKT7-GhSCL4 fusion protein could grow normally on both SD\-Trp and SD\-Trp\-Ade\-His media, whereas those transformed with the empty pGBKT7 vector did not survive on the SD\-Trp\-Ade\-His plates.
Figure 1: Expression pattern and transactivation assay of GhSCL4. (A) Gene expression profiles of nine SCL4/7 subgroup genes in the GRAS family under salt stress. (B) Expression patterns of GhSCL4 after salt treatment. Significant differences between groups were calculated using Student’s t test (**P < 0.01, *P < 0.05). (C) Subcellular localization of GhSCL4 in Nicotiana benthamiana cells. YFP, yellow fluorescent protein. Bars, 50 μm. (D) Transactivation activities of GhSCL4 in yeast cells
3.2 Over-Expressed GhSCL4 Increases Tolerance to Salt Stress
To investigate the function of GhSCL4 in plants, GhSCL4 was ectopically overexpressed in wild-type Arabidopsis plants and three homozygous overexpression lines (OE1, OE2 and OE3) were obtained. To determine whether GhSCL4 is involved in plant responses to salt, the growth of OE and WT plants grown on solidified medium was compared. When grown on MS medium, the growth of OE plants was similar to that of WT plants (data not shown). However, in the presence of 150 mM NaCl, the GhSCL4-overexpression plants exhibited higher tolerance to NaCl as indicated by the higher cotyledon greening rates of the OE plants than that of the control plants. An estimated 31.41% ± 4.24% of WT seedlings had green leaves, while 92.59% ± 4.24%, 78.7% ± 6.99% and 88.89% ± 5.56% of the OE1, OE2 and OE3 lines had green cotyledons, respectively (Figs. 2A, 2B).
Figure 2: GhSCL4 acts as a positive regulator of salt tolerance in transgenic Arabidopsis. (A) Morphology of 10-d-old seedlings of wild-type (WT) and transgenic lines (OE1, OE2 and OE3) grown on MS medium with 150 mM NaCl. (B) Quantitative analysis of the cotyledon greening rates of WT and OE lines shown in A. (C) Root lengths of different lines under normal (MS) or salt-treated conditions. (D) Statistical analysis comparing root lengths of seedlings grown in treatment conditions as described in C. (E) Photographs of representative seedlings of WT and three overexpression lines were taken after a 2-week 200-mM NaCl treatment. (F) The leaf withering rates of 15 individuals from each salt-treated lines of WT and GhSCL4 OEs. Significant differences between groups were calculated using Student’s t test (**P < 0.01, *P < 0.05)
Considering that root architecture has an important role in the response of plants to abiotic stress, root lengths of transgenic and wild-type plants under salt stress were examined. Regardless of whether NaCl was present, lengths of the primary roots of GhSCL4-overexpressing plants were generally longer than roots of WT. Roots of OE lines were also longer than that of WT lines in the NaCl treatments at concentrations of 100 and 120 mM (Figs. 2C, 2D). Thus GhSCL4-overexpressing plants were able to maintain higher root activity under either normal or salt stress conditions, indicating enhanced salt tolerance in overexpressing plants.
Salt tolerance assays of the seedlings grown in soil were also performed, and the result showed that the growth of GhSCL4-overexpressing plants and wild type plants were both severely inhibited, though the wild type plants were more sensitive to salt stress (Fig. 2E). After salt treatment, the leaf withering rate of OE lines was approximately 15%, which is significantly lower than that (>55%) of the WT plants (Fig. 2F).
3.3 Over-Expressed GhSCL4 Improves Tolerance to Osmotic Stress
The tolerance of GhSCL4-overexpressed lines and control plants to mannitol-simulated osmotic stress was determined. After 10 days of growth on mannitol medium, the cotyledon greening rates of three overexpression lines (OE1, OE2 and OE3) were all significantly higher than that of WT (36.11% ± 2.77%) (Figs. 3A, 3B). Additionally, the effect of osmotic stress inhibited elongation of primary roots. The root lengths from both overexpression and WT lines gradually decreased as the mannitol concentration increased from 200 to 250 mM, though the root lengths of overexpression lines were significantly longer than that of wild-type plants in the presence of various concentrations of mannitol (Figs. 3C, 3D). Thus, over-expression of GhSCL4 could enhance the osmotic tolerance of plants.
Figure 3: Osmotic tolerance of GhSCL4-overexpressing Arabidopsis. (A) Morphology of GhSCL4-overexpressing lines and WT grown on MS medium with 400 mM mannitol at day 10 of treatment. (B) Cotyledon greening rates of OE lines and WT as shown in A. (C) Root lengths of different lines under normal (0 mM mannitol) or mannitol-treated conditions. (D) Statistical analysis of root lengths of seedlings grown in treatment conditions as described in C. Significant differences between groups were calculated using Student’s t test (**P < 0.01, *P < 0.05)
3.4 The Expression of Salt Stress-Induced and Oxidation-Related Genes were Enhanced in GhSCL4 Over-Expression Arabidopsis Lines under Salt Stress
To study the molecular mechanism of GhSCL4 in response to salt stress, the transcript levels of salt-responsive genes were determined under both normal conditions and NaCl treatments by real-time PCR analysis. The result showed that the transcript levels of salt-induced genes, including AtNHX1 and AtSOS1, were stress inducible in both OE lines and WT, but the transcript levels were substantially higher in the OE lines than in WT plants after salt treatment (Fig. 4). Salt stress can lead to accumulation of ROS and result in oxidative damage, the transcript levels of oxidation-related genes were calculated as well. In transgenic Arabidopsis over-expressing GhSCL4, the oxidation-related genes AtAPX3 and AtCSD2 had more transcripts under salt-stressed conditions than those in WT plants (Fig. 4). Overall, the results showed that GhSCL4 may enhance tolerance to salt in part by promoting the expression of salt stress-induced and oxidation-related genes under salt condition.
Figure 4: Real time PCR analysis of salt stress-induced and oxidation-related genes in WT and transgenic (OE) Arabidopsis plants in the presence or absence of 175 mM NaCl. AtACTIN2 was taken as the reference gene. Significant differences between groups were calculated using Student’s t test (**P < 0.01, *P < 0.05)
3.5 Silencing of GhSCL4 Decreased Salt Tolerance in Cotton
To further confirm the role of GhSCL4 in modulating abiotic stress in cotton, TRV-based VIGS was conducted to interfere with the expression of GhSCL4 and obtain GhSCL4-silenced cotton. Cotton lines injected with the empty vector were taken as the control. The interference efficiency was assessed using qRT-PCR, and the result showed that the transcript level of GhSCL4 in GhSCL4-silenced plants was significantly lower than that of the control (Fig. 5A). Under normal growth condition, no obvious difference was detected between the control and GhSCL4-silenced plants. However, following irrigation with 300 mM NaCl solution for three weeks, the leaves of GhSCL4-silenced plants yellowed, shrunk, rolled or wilted, while the control plants grew normally (Fig. 5B).
Figure 5: Phenotypes of GhSCL4-silenced plants in response to salt stress. (A) The expression levels of GhSCL4 in GhSCL4-silenced (TRV::GhSCL4) and control (TRV::00) plants. (B) Phenotype analysis of salt-stressed GhSCL4-silenced and control cotton plants. (C) DAB staining to observe H2O2 in cotton. (D) Measurement of antioxidant enzyme (CAT, SOD, and POD) activities from leaves harvested from GhSCL4-silenced and control cotton plants. Significant differences between groups were calculated using Student’s t test (**P < 0.01, *P < 0.05)
To investigate whether GhSCL4 could participate in regulating H2O2 content under salt stress, we detected the accumulation of H2O2 in GhSCL4-silenced and control plants using DAB staining. Under normal conditions (no salt stress), very little H2O2 accumulated in both GhSCL4-silenced and control plants and there were no significant differences between them. However, when they were exposed to NaCl, H2O2 was highly accumulated in the leaves of NaCl-treated plants, as indicated by the extensive staining by DAB (Fig. 5C). However, the GhSCL4-silenced plants accumulated more H2O2 than control plants under salt treatment (Fig. 5C). In addition, changes in the activities of antioxidant enzyme (CAT, SOD, and POD) in GhSCL4-silenced plants and control plants were investigated. The results showed that under normal conditions, there were no significant differences in the antioxidant enzyme activities between GhSCL4-silenced and control plants, while GhSCL4-silenced plants exhibited lower CAT and SOD activities than those of control plants under salt treatment (Fig. 5D). Overall, these results suggest that GhSCL4 enhances salt tolerance of plants by detoxifying ROS.
3.6 GhSCL4 Physically Interacts with GhCaM7
In order to provide insight into the regulation network of GhSCL4 in response to abiotic stress, we predicted the GhSCL4-interacting proteins based on the database of co-expression networks for cotton (http://structuralbiology.cau.edu.cn/gossypium/cytoscape/network.php) and identified 14 genes that co-expressed with GhSCL4. Gene Ontogeny analysis was conducted to detect the pathways of these co-expression genes. The genes classified into nine pathways listed in Fig. 6A, including calcium ion binding, MAP kinase activity, ATP binding, chromatin binding, protein tyrosine kinase activity. Among the pathways, the number of co-expression genes belonging to the calcium ion binding pathway was the highest, and annotation of these genes revealed that they showed high similarity to calmodulin proteins (CaM7 and CaM5) and CaM-like proteins (CML11 and CML8) in Arabidopsis, respectively (Supplementary Table S2). To further confirm the interaction of GhSCL4 and these calcium ion binding proteins, a LCI assay was conducted to confirm the interaction between GhSCL4 and GhCaM7. As shown in Fig. 6B, only the coexpression of CLuc-GhCaM7 and NLuc-GhSCL4 in tobacco epidermal cells complemented the LUC activity. Additionally, BiFC assay also showed that GhCaM7 (Gh_A05G3893) could interact with GhSCL4 in the nucleus (Fig. 6C). Additionally, VIGS assay was conducted to obtain GhCaM7 silenced plants and investigate the role of GhCaM7 in response to salt stress in cotton. qRT-PCR analysis confirmed that the transcript level in GhCaM7-silenced plants was significantly lower than that in control plants (Fig. 6D). When all plants were treated with 300 mM NaCl for nearly four weeks, results showed that the leaves of GhCaM7-silenced plants were more yellow and wilted, indicating GhCaM7 is a positive regulator in response to salt stress (Fig. 6E).
Figure 6: The interaction between GhSCL4 and GhCaM7. (A) Gene Ontology enrichment analysis of GhSCL4 co-expression genes. (B) Luciferase assay to determine interaction between GhSCL4 and GhCaM7. (C) BiFC assays showing the interaction of GhSCL4 and GhCaM7 in tobacco leaves. (D) The expression levels of GhCaM7 in GhCaM7-silenced (TRV::GhCaM7) and control (TRV::00) plants. Significant differences between groups were calculated using Student’s t test (**P < 0.01, *P < 0.05). (E) Control cotton plants exhibited more enhanced salt resistance than GhCaM7-silenced plants did
4.1 GhSCL4 Functions as a Positive Regulator in Salt Stress Responses
With the growing conflict of land use to grow grain crops or cotton, cotton has been gradually displaced to areas with saline-alkali soils [3]. Therefore, mining salt tolerance genes is important for breeding salt-tolerant cotton cultivars. Several SCL4/7 subgroup genes in the GRAS family of genes have been reported to participate in plant development and abiotic stress [43–45]. For example, transgenic Arabidopsis overexpressing the poplar GRAS gene PeSCL7 exhibited significantly increased salt and drought tolerance at the seedling stage [43]. In this study, transgenic Arabidopsis overexpressing GhSCL4 showed enhanced resistance to salt and osmotic stress. Silencing of GhSCL4 resulted in reduced salt and drought tolerance in cotton. Salt stress can led to osmotic stress, which in turn can result in turgor loss in plant cells [45]. Thus, the osmotic stress tolerance conferred by GhSCL4 may contribute to the increased salt resistance in plants. Recently, several GRAS proteins, such as LISCL and NSP1/NSP2, have been confirmed as transcription factors with transcription activation activity [46]. Our transient expression assay showed that the GhSCL4 protein could localize to the cell nucleus, and our transactivation activity assay in the AH109 yeast cells showed that GhSCL4 could activate the reporter genes Ade and His of the yeast (Figs. 1B, 1C). Thus, GhSCL4 may function as a transcriptional regulator.
When a plant is subjected to abiotic stress in the soil, root tips are the plant parts that are first exposed to stress signals, and consequently the adverse conditions will inhibit primary root growth by decreasing cell production and size [7,47]. Plants that can produce longer and more extensive roots can explore farther soil regions in search of available water, thus some studies have demonstrated that root length is positively associated with salt stress in several crop species such as cotton and soybean [40,48]. Here, our comparison of the primary root lengths of GhSCL4 transgenic and wild-type Arabidopsis showed that regardless of whether roots were exposed to NaCl or mannitol, the primary root length of GhSCL4 transgenic plants was significantly longer than that of control plans (Figs. 2C, 3C). Thus, GhSCL4 functions as a positive regulator of primary root elongation, and the longer primary root length of GhSCL4 transgenic seedlings likely enhanced its ability to reach and absorb more water in deeper soils to provide partial relief from the low availability of water from the high osmotic conditions. Although the exact molecular mechanism underlying the role of GhSCL4 in primary root elongation requires further investigation, we report that root architecture may be a key determinant in plant response to soil abiotic stresses.
4.2 The Regulation Network of GhSCL4 in Response to Salt Stress
At present, there are only a few studies that report the mechanisms of GRAS family genes in plant abiotic stress response. A poplar SCL gene, PeSCL7, alleviated salt toxicity in transgenic plants by elevating enzyme activity of the ROS-scavenging SOD [43]. Salt stress induces the accumulation of ROS, and excessive accumulation of ROS causes oxidative damage to membrane lipids [19]. Thus, the increased activity of the SOD enzyme induced by overexpression of PeSCL7 likely controlled the accumulation of ROS to more tolerable levels under the abiotic stress. Here, we demonstrated that GhSCL4 may regulate ROS levels in response to salt stress. The major species of ROS, H2O2, was detected at higher amounts in GhSCL4-silenced plants than in control plants under salt treatment, and GhSCL4-silenced plants exhibited lower CAT and SOD activities than those of control plants under salt treatment (Fig. 5D). Furthermore, after salt treatment, the transcripts of oxidation-related genes (AtAPX3 and AtCSD2) of GhSCL4 over-expression Arabidopsis were significantly higher than those in WT plants (Fig. 4). Overall, these results suggest that GhSCL4 enhances salt tolerance of plants by detoxifying ROS.
The transient expression assay using both LCI and BiFC confirmed the interaction between GhSCL4 and GhCaM7 (Figs. 6B, 6C). Similarly, AtSCL4, the homologous protein of GhSCL4, was also identified as an AtCaM7-interacting protein via protein microarrays [49]. Calmodulin is one of the prominent calcium sensors in plants and are reportedly involved in plant response to abiotic stress [13,15,16]. Here, silenced GhCaM7 in cotton decreased its level of salt tolerance, indicating that GhCaM7 is a positive regulator in cotton response to salt stress. Intracellular CaM may also play a critical role in maintaining ROS homeostasis. In Arabidopsis, CaM interacted with the H2O2-scavenging enzyme CAT3 to control ROS levels in a Ca2+ dependent manner [50], and CaM also participated indirectly in regulating ROS levels through CaM-regulated λ-aminobutyrate (GABA) synthesis and the GABA shunt metabolic pathway [51]. Thus, the enhanced ROS-scavenging capacity conferred by GhSCL4 might be associated with Ca2+/CaM7-mediated signaling. Thus, we surmised that the interaction between GhSCL4 and GhCaM7 may be occurring at the protein level, so further exploration using biochemical and molecular experiments are needed to elucidate the complex signalling mechanism.
Overall, we identified a new SCL gene GhSCL4 that conferred enhanced tolerance to salt treatments in cotton plants. The interaction between GhSCL4 and GhCAM7 indicated that GhSCL4 might be involved in Ca2+/CaM7-mediated control of ROS levels when plants are under salt stress. Gene function analysis of GhSCL4 provides beneficial information in developing bio-engineering strategies to improve the stress tolerance of cotton.
Authorship: Yanyan Zhao, Yanpeng Ding and Bailin Duan conceived and designed the experiments. Yanyan Zhao and Qingzhou Xie drafted the manuscript. Yanyan Zhao revised the manuscript.
Funding Statement: This work was supported by funding from the National Natural Science Foundation of China (Grant No. 32101683), and the Fundamental Research Funds of Zhejiang Sci-Tech University (Grant No. 11612932612116).
Conflicts of Interest: The authors declare that they have no conflict of interest.
References
1. Apse, M. P., Aharon, G. S., Snedden, W. A., Blumwald, E. (1999). Salt tolerance conferred by overexpression of a vacuolar Na+/H+ antiport in Arabidopsis. Science, 285(5431), 1256–1258. DOI 10.1126/science.285.5431.1256. [Google Scholar] [CrossRef]
2. Chinnusamy, V., Zhu, J., Zhu, J. K. (2006). Salt stress signaling and mechanisms of plant salt tolerance. Genetic Engineering, 27, 141–177. DOI 10.1007/0-387-25856-6_9. [Google Scholar] [CrossRef]
3. Ashraf, M. (2002). Salt tolerance of cotton: Some new advances. Critical Reviews in Plant Sciences, 21(1), 1–30. DOI 10.1080/0735-260291044160. [Google Scholar] [CrossRef]
4. Munns, R., Tester, M. (2008). Mechanisms of salinity tolerance. Annual Review of Plant Biology, 59(1), 651–681. DOI 10.1146/annurev.arplant.59.032607.092911. [Google Scholar] [CrossRef]
5. Glenn, E. P., Brown, J. J., Blumwald, E. (1999). Salt tolerance and crop potential of halophytes. Critical Reviews in Plant Sciences, 18(2), 227–255. DOI 10.1080/07352689991309207. [Google Scholar] [CrossRef]
6. Munns, R. (2005). Genes and salt tolerance: Bringing them together. The New Phytologist, 167(3), 645–663. DOI 10.1111/j.1469-8137.2005.01487.x. [Google Scholar] [CrossRef]
7. Xiong, L., Zhu, J. K. (2002). Salt tolerance. Arabidopsis Book, 1(3), 1–22. DOI 10.1199/tab.0048. [Google Scholar] [CrossRef]
8. Weinl, S., Kudla, J. (2009). The CBL-CIPK Ca2+-decoding signaling network: Function and perspectives. The New Phytologist, 184(3), 517–528. DOI 10.1111/j.1469-8137.2009.02938.x. [Google Scholar] [CrossRef]
9. Deinlein, U., Stephan, A. B., Horie, T., Luo, W., Xu, G. et al. (2014). Plant salt-tolerance mechanisms. Trends in Plant Science, 19(6), 371–379. DOI 10.1016/j.tplants.2014.02.001. [Google Scholar] [CrossRef]
10. Cheng, S. H., Willmann, M. R., Chen, H. C., Sheen, J. (2002). Calcium signaling through protein kinases. The Arabidopsis calcium-dependent protein kinase gene family. Plant Physiology, 129(2), 469–485. DOI 10.1104/pp.005645. [Google Scholar] [CrossRef]
11. Ludwig, A. A., Romeis, T., Jones, J. D. (2004). CDPK-mediated signalling pathways: Specificity and cross-talk. Journal of Experimental Botany, 55(395), 181–188. DOI 10.1093/jxb/erh008. [Google Scholar] [CrossRef]
12. Xu, J., Tian, Y. S., Peng, R. H., Xiong, A. S., Zhu, B. et al. (2010). AtCPK6, a functionally redundant and positive regulator involved in salt/drought stress tolerance in Arabidopsis. Planta, 231(6), 1251–1260. DOI 10.1007/s00425-010-1122-0. [Google Scholar] [CrossRef]
13. Reddy, V. S., Reddy, A. S. (2004). Proteomics of calcium-signaling components in plants. Phytochemistry, 65(12), 1745–1776. DOI 10.1016/j.phytochem.2004.04.033. [Google Scholar] [CrossRef]
14. Mehlmer, N., Wurzinger, B., Stael, S., Hofmann-Rodrigues, D., Csaszar, E. et al. (2010). The Ca2+-dependent protein kinase CPK3 is required for MAPK-independent salt-stress acclimation in Arabidopsis. The Plant Journal, 63(3), 484–498. DOI 10.1111/j.1365-313X.2010.04257.x. [Google Scholar] [CrossRef]
15. McCormack, E., Tsai, Y. C., Braam, J. (2005). Handling calcium signaling: Arabidopsis CaMs and CMLs. Trends in Plant Science, 10(8), 383–389. DOI 10.1016/j.tplants.2005.07.001. [Google Scholar] [CrossRef]
16. Rao, S. S., El-Habbak, M. H., Havens, W. M., Singh, A., Zheng, D. et al. (2014). Overexpression of GmCaM4 in soybean enhances resistance to pathogens and tolerance to salt stress. Molecular Plant Pathology, 15(2), 145–160. DOI 10.1111/mpp.12075. [Google Scholar] [CrossRef]
17. Choudhury, F. K., Rivero, R. M., Blumwald, E., Mittler, R. (2017). Reactive oxygen species, abiotic stress and stress combination. The Plant Journal, 90(5), 856–867. DOI 10.1111/tpj.13299. [Google Scholar] [CrossRef]
18. van Breusegem, F., Bailey-Serres, J., Mittler, R. (2008). Unraveling the tapestry of networks involving reactive oxygen species in plants. Plant Physiology, 147(3), 978–984. DOI 10.1104/pp.108.122325. [Google Scholar] [CrossRef]
19. You, J., Chan, Z. (2015). ROS regulation during abiotic stress responses in crop plants. Front Plant Science, 6, 1092. DOI 10.3389/fpls.2015.01092. [Google Scholar] [CrossRef]
20. Del Río, L. A. (2015). ROS and RNS in plant physiology: An overview. Journal of Experimental Botany, 66(10), 2827–2837. DOI 10.1093/jxb/erv099. [Google Scholar] [CrossRef]
21. Zhang, M., Smith, J. A., Harberd, N. P., Jiang, C. (2016). The regulatory roles of ethylene and reactive oxygen species (ROS) in plant salt stress responses. Plant Molecular Biology, 91(6), 651–659. DOI 10.1007/s11103-016-0488-1. [Google Scholar] [CrossRef]
22. Huang, H., Ullah, F., Zhou, D. X., Yi, M., Zhao, Y. (2019). Mechanisms of ROS regulation of plant development and stress responses. Frontiers in Plant Science, 10, 800. DOI 10.3389/fpls.2019.00800. [Google Scholar] [CrossRef]
23. Jiang, Y., Deyholos, M. K. (2009). Functional characterization of Arabidopsis NaCl-inducible WRKY25 and WRKY33 transcription factors in abiotic stresses. Plant Molecular Biology, 69(1–2), 91–105. DOI 10.1007/s11103-008-9408-3. [Google Scholar] [CrossRef]
24. Yang, O., Popova, O. V., Süthoff, U., Lüking, I., Dietz, K. J. et al. (2009). The Arabidopsis basic leucine zipper transcription factor AtbZIP24 regulates complex transcriptional networks involved in abiotic stress resistance. Gene, 436(1–2), 45–55. DOI 10.1016/j.gene.2009.02.010. [Google Scholar] [CrossRef]
25. Kasuga, M., Liu, Q., Miura, S., Yamaguchi-Shinozaki, K., Shinozaki, K. (1999). Improving plant drought, salt, and freezing tolerance by gene transfer of a single stress-inducible transcription factor. Nature Biotechnology, 17(3), 287–291. DOI 10.1038/7036. [Google Scholar] [CrossRef]
26. Dubos, C., Stracke, R., Grotewold, E., Weisshaar, B., Martin, C. et al. (2010). MYB transcription factors in Arabidopsis. Trends in Plant Science, 15(10), 573–581. DOI 10.1016/j.tplants.2010.06.005. [Google Scholar] [CrossRef]
27. Zhao, Y., Yang, Z., Ding, Y., Liu, L., Han, X. et al. (2019). Over-expression of an R2R3 MYB Gene, GhMYB73, increases tolerance to salt stress in transgenic Arabidopsis. Plant Science, 286, 28–36. DOI 10.1016/j.plantsci.2019.05.021. [Google Scholar] [CrossRef]
28. Wang, P., Su, L., Gao, H., Jiang, X., Wu, X. et al. (2018). Genome-wide characterization of bHLH genes in grape and analysis of their potential relevance to abiotic stress tolerance and secondary metabolite biosynthesis. Frontiers in Plant Science, 9(64), 63. DOI 10.3389/fpls.2018.00064. [Google Scholar] [CrossRef]
29. Bolle, C. (2004). The role of GRAS proteins in plant signal transduction and development. Planta, 218(5), 683–692. DOI 10.1007/s00425-004-1203-z. [Google Scholar] [CrossRef]
30. Gallagher, K. L., Benfey, P. N. (2009). Both the conserved GRAS domain and nuclear localization are required for SHORT-ROOT movement. Plant Journal, 57(5), 785–797. DOI 10.1111/j.1365-313X.2008.03735.x. [Google Scholar] [CrossRef]
31. Lee, M. H., Kim, B., Song, S. K., Heo, J. O., Yu, N. I. et al. (2008). Large-scale analysis of the GRAS gene family in Arabidopsis thaliana. Plant Molecular Biology, 67(6), 659–670. DOI 10.1007/s11103-008-9345-1. [Google Scholar] [CrossRef]
32. Benfey, P. N., Linstead, P. J., Roberts, K., Schiefelbein, J. W., Hauser, M. T. et al. (1993). Root development in Arabidopsis: Four mutants with dramatically altered root morphogenesis. Development, 119(1), 57–70. DOI 10.1242/dev.119.1.57. [Google Scholar] [CrossRef]
33. Fode, B., Siemsen, T., Thurow, C., Weigel, R., Gatz, C. (2008). The Arabidopsis GRAS protein SCL14 interacts with class II TGA transcription factors and is essential for the activation of stress-inducible promoters. Plant Cell, 20(11), 3122–3135. DOI 10.1105/tpc.108.058974. [Google Scholar] [CrossRef]
34. Chen, K., Li, H., Chen, Y., Zheng, Q., Li, B. et al. (2015). TaSCL14, a novel wheat (Triticum aestivum L.) GRAS gene, regulates plant growth, photosynthesis, tolerance to photooxidative stress, and senescence. Journal of Genetics Genomics, 42(1), 21–32. DOI 10.1016/j.jgg.2014.11.002. [Google Scholar] [CrossRef]
35. Xu, K., Chen, S., Li, T., Ma, X., Liang, X. et al. (2015). OsGRAS23, a rice GRAS transcription factor gene, is involved in drought stress response through regulating expression of stress-responsive genes. BMC Plant Biology, 15(141), 3509. DOI 10.1186/s12870-015-0532-3. [Google Scholar] [CrossRef]
36. Qiao, Y., Liu, L., Xiong, Q., Flores, C., Wong, J. et al. (2013). Oomycete pathogens encode RNA silencing suppressors. Nature Genetics, 45(3), 330–333. DOI 10.1038/ng.2525. [Google Scholar] [CrossRef]
37. Zhang, T., Hu, Y., Jiang, W., Fang, L., Guan, X. et al. (2015). Sequencing of allotetraploid cotton (Gossypium hirsutum L. acc. TM-1) provides a resource for fiber improvement. Nature Biotechnology, 33(5), 531–537. DOI 10.1038/nbt.3207. [Google Scholar] [CrossRef]
38. Pang, J., Zhu, Y., Li, Q., Liu, J., Tian, Y. et al. (2013). Development of Agrobacterium-mediated virus-induced gene silencing and performance evaluation of four marker genes in Gossypium barbadense. PLoS One, 8(9), e73211. DOI 10.1371/journal.pone.0073211. [Google Scholar] [CrossRef]
39. Li, Z., Li, L., Zhou, K., Zhang, Y., Han, X. et al. (2019). GhWRKY6 acts as a negative regulator in both transgenic arabidopsis and cotton during drought and salt stress. Frontiers in Genetics, 10, 392. DOI 10.3389/fgene.2019.00392. [Google Scholar] [CrossRef]
40. Wei, Z., Shi, X., Wei, F., Fan, Z., Mei, L. et al. (2019). The cotton endocycle-involved protein SPO11-3 functions in salt stress via integrating leaf stomatal response, ROS scavenging and root growth. Physiologia Plantarum, 167(1), 127–141. DOI 10.1111/ppl.12875. [Google Scholar] [CrossRef]
41. Yu, X., Li, L., Li, L., Guo, M., Chory, J. et al. (2008). Modulation of brassinosteroid-regulated gene expression by Jumonji domain-containing proteins ELF6 and REF6 in Arabidopsis. PNAS, 105(21), 7618–7623. DOI 10.1073/pnas.0802254105. [Google Scholar] [CrossRef]
42. Chen, H., Zou, Y., Shang, Y., Lin, H., Wang, Y. et al. (2008). Firefly luciferase complementation imaging assay for protein-protein interactions in plants. Plant Physiology, 146(2), 368–376. DOI 10.1104/pp.107.111740. [Google Scholar] [CrossRef]
43. Ma, H. S., Liang, D., Shuai, P., Xia, X. L., Yin, W. L. (2010). The salt- and drought-inducible poplar GRAS protein SCL7 confers salt and drought tolerance in Arabidopsis thaliana. Journal of Experimental Botany, 61(14), 4011–4019. DOI 10.1093/jxb/erq217. [Google Scholar] [CrossRef]
44. Tang, W., Tu, L., Yang, X., Tan, J., Deng, F. et al. (2014). The calcium sensor GhCaM7 promotes cotton fiber elongation by modulating reactive oxygen species (ROS) production. The New Phytologist, 202(2), 509–520. DOI 10.1111/nph.12676. [Google Scholar] [CrossRef]
45. Krasensky, J., Jonak, C. (2012). Drought, salt, and temperature stress-induced metabolic rearrangements and regulatory networks. Journal of Experimental Botany, 63(4), 1593–1608. DOI 10.1093/jxb/err460. [Google Scholar] [CrossRef]
46. Smit, P., Raedts, J., Portyanko, V., Debellé, F., Gough, C. et al. (2005). NSP1 of the GRAS protein family is essential for rhizobial Nod factor-induced transcription. Science, 308(5729), 1789–1791. DOI 10.1126/science.1111025. [Google Scholar] [CrossRef]
47. West, G., Inzé, D., Beemster, G. T. (2004). Cell cycle modulation in the response of the primary root of Arabidopsis to salt stress. Plant Physiology, 135(2), 1050–1058. DOI 10.1104/pp.104.040022. [Google Scholar] [CrossRef]
48. Li, S., Wang, N., Ji, D., Zhang, W., Wang, Y. et al. (2019). A GmSIN1/GmNCED3s/GmRbohBs feed-forward loop acts as a signal amplifier that regulates root growth in soybean exposed to salt stress. Plant Cell, 31(9), 2107–2130. DOI 10.1105/tpc.18.00662. [Google Scholar] [CrossRef]
49. Popescu, S. C., Popescu, G. V., Bachan, S., Zhang, Z., Seay, M. et al. (2007). Differential binding of calmodulin-related proteins to their targets revealed through high-density Arabidopsis protein microarrays. PNAS, 104(11), 4730–4735. DOI 10.1073/pnas.0611615104. [Google Scholar] [CrossRef]
50. Yang, T., Poovaiah, B. W. (2002). Hydrogen peroxide homeostasis: Activation of plant catalase by calcium/calmodulin. PNAS, 99(6), 4097–4102. DOI 10.1073/pnas.052564899. [Google Scholar] [CrossRef]
51. Bouché, N., Fait, A., Bouchez, D., Møller, S. G., Fromm, H. (2003). Mitochondrial succinic-semialdehyde dehydrogenase of the gamma-aminobutyrate shunt is required to restrict levels of reactive oxygen intermediates in plants. PNAS, 100(11), 6843–6848. DOI 10.1073/pnas.1037532100. [Google Scholar] [CrossRef]
Supplemental Data
Supplementary Table S1: Primer sequences used in this study.
Supplementary Table S2: Annotations of GhSCL4 co-expression genes.
Cite This Article
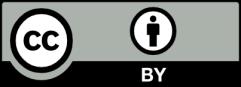
This work is licensed under a Creative Commons Attribution 4.0 International License , which permits unrestricted use, distribution, and reproduction in any medium, provided the original work is properly cited.