Open Access
REVIEW
The Role of Salicylic Acid Signal in Plant Growth, Development and Abiotic Stress
1 Shanghai Key Laboratory of Bio-Energy Crops, School of Life Sciences, Shanghai University, Shanghai, 200444, China
2 Department of Applied Sciences, University of the West of England, Bristol, BS16 1QY, UK
* Corresponding Author: Xiangyang Hu. Email:
Phyton-International Journal of Experimental Botany 2022, 91(12), 2591-2605. https://doi.org/10.32604/phyton.2022.023733
Received 12 May 2022; Accepted 26 July 2022; Issue published 29 August 2022
Abstract
In nature, plants are constantly affected by adverse conditions. Unlike animals, plants can resist these adverse stresses only by insisting on their original positions. Stress can be divided into biological stress and abiotic stress, abiotic stress directly affects the growth, development and yield of plants, it spans all developmental stages from seed germination to senescence. In order to adapt to changing environment, plants have evolved well-developed mechanisms that help to perceive the stress signals and enable optimal growth response. Salicylic acid (SA) is an important endogenous signal molecule in plants, which not only regulate some plant growth and development processes, but also plays an important part in plant stress resistance. Much work about salicylic acid has been done on the immunity of plants to pathogens, and the synthesis and signal transduction of SA are clearly understood, its function in plant growth, development and abiotic stress is also well learned, we systemically summarized the multiple function of SA signal in non-pathogen-related response, such review should help us understand the common but essential function of SA signal in modulating plant growth, development and abiotic stress.Keywords
Plants are exposed to a variety of environmental stresses throughout their lives [1]. In addition to biotic stresses, plants can also be impacted by a variety of abiotic stresses (drought, cold, high temperature, high salt, or toxic metal ions) [2]. The frequency of extreme weather increases due to climate change, and the adverse effects of abiotic stress on plants deepen. Plants have to evolve more sophisticated mechanisms to cope with these stresses. One of the strategies for plants is to adjust their growth to the changing environment through signals transmitted by plant hormones [3].
Salicylic acid (SA), also called ortho-hydroxybenzoic acid, is a simple phenolic compound that participates in the regulation of diverse metabolism in plants. It is considered as a new plant hormone and plays an important role in improving plant stress resistance [4]. SA, classified under the group of plant hormones, has been reported to induce heat production in inflorescences of thermogenic species [5], stimulate flowering and enhance flower longevity, inhibit ethylene biosynthesis, block the wound response, and reverse the effect of abscisic acid [6]. Studies have demonstrated that SA can induce plant resistance to heavy metal, ozone, ultraviolet radiation, high temperature, salt stress and other abiotic stresses [7]. SA also plays a major role in plant growth and development, as there is evidence that SA regulates seed germination, vegetative growth, photosynthesis, respiration, thermogenesis, flower formation, seed production, senescence and other life processes [8].
In the past two decades, increasing evidence has shown that SA plays essential roles in regulating plant development by affecting the immunity of plants to pathogens. However, the action of SA in the regulation of plant growth has not been comprehensively described yet in most reviews, compared to the role of other plant hormones, such as auxin and gibberellin. This review mainly describes the mechanisms of SA in regulating plant in abiotic stress. This knowledge of the SA-mediated development regulation will contribute to the future crop improvement.
2 The Synthesis, Conjugation and Transport of SA
SA is a small phenolic compound that plays a significant role in plant pathogen defense [9]. Studies have shown that chorismate produced by the shikimic acid pathway is an important precursor substance for the synthesis of SA in plants. There are two pathways for the synthesis of SA, the isochorismate synthase (ICS) pathway and the phenylalanine ammonia-lyase (PAL) pathway (Fig. 1) [10].
Figure 1: Salicylic acid biosynthesis in plants. There are two pathways of SA biosynthesis: the ICS1/2 (isochromatic synthase) pathway and the PAL (phenylalanine ammonia-lyase) pathway. Both pathways begin with chorismite as the substrate, which is produced via a seven-step enzymatic reaction of shikimate pathway in chloroplast. (1) ICS pathway: After isochorismate is generated by ISC1/2, it interacts with PBS3 binds isochorismate to Glu to generate IC-9-Glu (isochorismoyl-9-glutamate), which can break down into SA spontaneously. (2) PAL pathway: In the cytosol, phenylalanine generates mandelonitrile or trans-cinnamic acid under the action of phenylalanine ammonia-lyase. BA2H (Benzoic acid 2-hydroxylase) hydroxylated benzoate to SA
Studies on Arabidopsis sid2 (SA-DEFICIENT 2) mutants found that after induction with pathogenic bacteria, ICS1 (ISOCHORISMATE SYNTHASE 1) was expressed at a time similar to the time of SA accumulation under these patho genic bacteria, the study showed that SA can be synthesized by chorismate through the ICS pathway, and plays an important role in the Arabidopsis SAR (systemic acquired resistance) defense response [11]. The homologue of ICS1, ICS2, is another protein that encodes synthetic SA, but ICS2 contributes much less to SA synthesis in Arabidopsis [12]. Fluorescence localization experiments demonstrated that both ICS1 and ICS2 proteins were located in the plastids, while SA was synthesized in the cytosol [13]. After isochorismate is generated by ISC1/2, it interacts with PBS3 (AvrPphB susceptible3) localized on the chloroplast plasma membrane to translocate isochorismate into the cytosol [13,14]. PBS3 is an acyl-adenylate/thioester-forming enzyme from GH3 (GLYCOSIDE HYDROLASE 3) protein family, hence also is named as GH3.12. PBS3 binds isochorismate to Glu via the amino acids (AAs) transferase activity of PBS3 (GH3.12) to generate IC-9-Glu (isochorismoyl-9-glutamate) [12,14]. IC-9-Glu is very unstable, it can spontaneously break down into SA when SA at a very low concentration. In a forward genetic mutant screen of Arabidopsis, EPS1(EXOPOLYSACCHARIDE 1) recently found in nature as IPGL (IC-9-Glu pyruvoyl-glutamatelyase), also can enhance SA biosynthesis.
In the PAL pathway, chorismate produced by the shikimate pathway undergoes a series of reactions to generate phenylalanine, which serves as a precursor for SA synthesis. PAL activity decreased by 90% after PAL gene mutation, while SA accumulation was decreased, indicating the involvement of the PAL pathway in SA biosynthesis [15,16]. In the cytosol, phenylalanine further generates mandelonitrile or generates trans-cinnamic acid under the action of PAL [17]. Trans-cinnamate acid and mandelonitrile are first converted to benzaldehyde, and then to benzoic acid. It is proposed that the role of benzoic acid 2-hydroxylase (BA2H) hydroxylated benzoate to SA, but the role of BA2H remains to be verified. In addition, trans-cinnamate acid can be converted to ortho-courmaric acid and it can form SA spontaneously [18]. The synthetic SA will be found in responses to the plant stress.
2.2 Conjugation and Transport of SA
SA can be chemically modified into various bioactive derivatives by glycation, methylation, sulfonation, AAs (amino acids) conjugation and hydroxylation [19]. With one hydroxyl and one carboxyl group, SA can be converted into two major derivatives via glycosylation. In Arabidopsis, two UGTs (uridine diphosphate-glucosyltransferases) have been reported to play roles in the glycosylation of SA. Both UGT74F1 and UGT74F2 in Arabidopsis can catalyze the conjugation of glucosyl group from UDP-glucose into the hydroxyl group of SA and produce SAG (SA 2-O-β-D-glucoside) [20]. UGT74F2, was also named as SGT1 (S-glucosyl transferase 1), which can also catalyze the conjugation of glycosyl onto the carboxyl group of SA and produce SGE (salicylate glucose ester) [21]. The carboxyl group of SA can be methylated by the SAM (S-adenosyl-L-methionine)-dependent MTs (methyltransferases) in plants. In Arabidopsis, the SAM-MT is encoded by the gene BSMT1 (BA/SA CARBOXYL METHYLTRANSFERASE 1) [22], which belongs to a 24-gene SABATH family of MTs. SABATH MTs is collectively called as the three earliest-identified genes in this family: SAMT (SALICYLIC ACID CARBOXYL METHYLTRANSFERASE), BAMT (BENZOIC ACID CARBOXYL METHYLTRANSFERASE), and THS (THEOBROMINE SYNTHASE) [23]. In bsmt1 mutant, MeSA (pathogen-induced methyl salicylate) accumulation is completely abolished. Similar to the glycosylation, methylation inactivates SA for its function and, reversibly, MeSA can be converted into active SA through the methylesterases (MESs) [24]. These conjugation reactions are catalyzed by cytoplasmic SA glucosyltransferases, and SAG is actively transported from the cytoplasm to the vacuoles of soybean and tobacco cells, where it may function as an inactive form of storage that can release free SA. Interestingly, SA is also converted by SA carboxymethyl transferase to MeSA, a volatile derivative that is an essential remote signal of resistance acquisition in tobacco and Arabidopsis systems. MeSA can be further glycosylated to produce methyl salicylate 2-O-β-D-glucose (MeSAG). But this SA-bound form is not stored in vacuoles [25].
Although free SA is not the primary signal that induces systemic acquired resistance, it also moves from the infected leaf to the upper uninoculated leaves via the sieve tubes [26]. It may be supposed that, in mature infected tissues [27], SA transport to the sieve elements occurs via either the symplastic or the apoplastic pathway according to the species, as described in the past for assimilates [28]. In the case of apoplastic phloem loading, SA must cross the plasma membrane of the companion cell-sieve element complex. Similarly, exogenous SA and analogs applied to the leaf surface must cross the plasma membrane of a cell to reach the phloem sap. The first biosynthesis of SA takes place in chloroplast, where chorismate is converted into isochorismate by ICS1, one of the most critical enzymes. Isochorismate, in turn, is transported by the membrane-located transporter EDS5 (ENHANCED DISEASE SUSCEPTIBILITY 5) in the chloroplast and is converted into SA in the cytoplasm through a series of processes [29]. As the cytoplasmic pH is higher than that of the pKa of SA, the SA in the cytoplasm is deprotonated and transformed into an active form by a series of methylation and glycosylation modifications [30]. At the same time, some SAG and SGE can enter the vacuole through active transport and be stored in an inactive form [29].
In late 1990s, the characterization of the bio-synthesis pathway was initiated in Arabidopsis through forward genetic screens [31]. Three key genes ICS1, EDS5 and PBS3 were cloned and were later considered to encode three enzymes that defined the canonic IC pathway of SA biosynthesis [32]. In plants, many resistance (R) genes are involved in pathogen reorganization and innate immune response activation, they share common binding sites for transcription factors, such as nucleotide binding sites (NBS) and leucine-rich repeat domains (LRR) and some of the NBS-LRR type resistance proteins in dicots have Toll/Interleukin-1 Receptor (TIR) motifs. Downstream of TIR-NBS-LRR R generequires EDS1 (ENHANCED DISEASE SUSCEPTIBILITY 1). While CC-NBS-LRR R gene NPR1 (NONEXPRESSER OF PATHOGENESIS-RELATED GENE 1) is required downstream of CCAs a lipase-like protein without lipase activity [33]. EDS1 plays a key role in plant basal resistance, SA accumulation, and effector protein-induced innate immunity (ETI) [34]. EDS1 causes the initial accumulation of SA and process of hypersensitivity reaction (HR), which then acts with PAD4(PHYTOALEXIN DEFICIENT4) to cause further accumulation of SA [35]. EDS5 and SID2 are located downstream of EDS1 and PAD4, and can directly affect the synthesis of SA [36]. In a genetic and transcriptomic analysis of Arabidopsis autoimmunity caused by constitutive or conditional EDS1/PAD4 overexpression, intrinsic EDS1/PAD4 signaling properties and their relation to SA were uncovered. SA also promotes the expression of PR genes, EDS1, PAD4, EDS5 and SID2 which amplify SA signal rapidly [37].
Arabidopsis carries five NPR1 homologues, which can be divided into three groups: NPR1 and NPR2 are the first group, NPR3 and NPR4 are the second group, and BOP1 (Blade-On-Petiole1) and BOP2 on petiole are the third group [38]. NPR1 cannot be the only SABP (SA-binding protein) in plants. NPR1 paralogs, NPR2, NPR3 and NPR4, also bind SA [39]. And almost all NPR1 analogues contain anchor protein repeats, which play an important role in protein-protein interaction. Among the five analogs, NPR1/2/3/4 achieves strong ability to bind SA in vitro, while BOP1/2 only has weak interaction with SA [40]. Recent studies have shown that NPR1 and NPR2 play an up-regulation role in the regulation of downstream genes that respond to SA, while NPR3 and NPR4 seem to play an opposite role to NPR1 and NPR2, that is, they negatively regulate the process of SA signaling (Fig. 2) [41].
Figure 2: Biosynthesis, transport and signal perception of SA in plants. There are two pathways of SA: the ICS1/2 pathway and the PAL pathway. SA can be converted into functional or nonfunctional metabolites via different modifications. All glycosylated salicylates are stored in vacuoles in plant cells. When c[SA] is low, NPR3 and NPR4 serve as negative regulators to suppress gene expressions via their direct interactions with plant-specific TGA transcription factors, while monomeric NPR1 protein is maintained in a steady and relatively low level. NPR1 cannot be the only SABP (SA-binding protein) in plants, NPR2, NPR3 and NPR4, also bind SA. In the nucleus, NPR1 forms a protein complex with CDK8 and EDS1, promoting the expression of the PR1 gene. The turnover of NPR1 protein is mediated by 26S proteasome complex. When plant facing pathogens and stress, the level of SA increase. Higher SA level can increase monomerization process of NPR1 and induced NPR1-dependent gene expression through direct interactions with TGA transcription factors
Recently, Chen et al. [42] throw new light onto how NPR1 regulates pathogenesis-related (PR1) expression. NPR1 forms a complex with TGA (TGACG cis-element-binding protein) transcription factors TGA5, TGA7, and CDK8 (CYCLIN-DEPENDENT-KINASE8), which forms a bridge between the RNA polymerase II and the PR1 promoter and consequently facilitates PR1 transcription [42]. In general, the monomer NPR1 protein in the plant is maintained at a relatively low and stable level, while the tetramer from NPR1 protein is maintained at a high level in the cytoplasm [43]. NPR1 and NPR3/4 work in anti-parallel in response to SA, in which SA activates NPR1 positive regulation and deactivates NPR3/4 negative regulation by direct binding to the respective NPR proteins [44]. Though the unique mutant allele of NPR4 reported in the second model has strongly suggested the later one is more likely to be real, it has not fully ruled out the biochemical possibility of NPR3/4 degrading NPR1. More importantly, it has not yet been fully understood how NPRs are regulated in response to basal level of SA vs. higher level of SA accumulation upon immune activation [45]. At low SA concentrations, S-nitrosylation of NPR1Cys156 by S-nitrosoglutathione (GSNO) encourages the existence of NPR1 in its oligomeric form. Furthermore, it was not known whether all NPR1 homologs would undergo similar biochemical processes to NPR1 in response to SA accumulation, such as monomerization, translocation from cytosol to nuclear, interaction with transcription factors (TFs), polyubiquitination, and rapid protein turnover.
However, when abiotic stress or pathogen invasion, plants will accumulate higher levels of SA. Increased SA in plants induces NPR1 protein monomerization and downstream SA responsive gene expression through direct interaction with TGA2 transcription factor [44]. In the nucleus, NPR1 forms a protein complex with CDK8 and EDS1, promoting the expression of the PR1 gene. NPR1 is degraded by the 26S proteasome complex through a series of polyubiquitination processes by CUL3 (Cullin 3) and the UBE4 (ubiquitin E4 ligase), and its de-ubiquitination is mediated by de-ubiquitinase UBP6 and UBP7, which are closely linked to 26S proteasome [45]. SA-induced gene expression requires efficient turnover of the monomer NPR1 protein in the nucleus, that is, the extracellular tetramer protein is degraded into monomer protein and transported into the nucleus [46]. When the concentration of monomer protein in the nucleus reaches a certain height, the protein interacts with some transcription factor to induce responsive gene expression, this is also dependent on the dynamic balance of the ubiquitination of NPR1 [46].
WRKY transcription factors play crucial roles in plants, including in response to various stresses. There are 74 WRKY transcription factors in Arabidopsis thaliana and 109 WRKY transcription factors in rice, which plays important roles in plant stress resistance [47]. Shan et al. [48] pointed that the expression level of MdWRKY17 in 6 susceptible apple (Malus domestica) germplasm was significantly higher than that in 6 tolerant apple germplasm, and the corresponding SA content was decreased, confirming the key role of MdWRKY17-mediated SA degradation in Glomerella leaf spot (GLS) tolerance. In a recent study, exogenous SA treatment was found to significantly inhibit the occurrence of Penicillium digitatum in citrus fruits. The researchers identified a transcription factor CsWRKY70 that responded positively to exogenous SA induction by transcriptomic sequencing, and found that SA carboxyl methyltransferase (CsSAMT) gene related to methyl salicylate synthesis also responded positively to SA induction [49].
4 The Role of Salicylic Acid in Plant Growth, Development and Abiotic Stresses
SA signaling can mediate a persistent, broad-spectrum disease resistance to pathogen infection known as systemic acquired resistance (SAR). In addition, as a signal molecule, SA regulates physiological processes such as plant respiration and metabolism, seed germination, flower induction, senescence and stress resistance. SA can also regulate stomatal movement, photosynthetic pigment content, photosynthetic mechanism performance and photosynthetic carbon assimilating enzyme activity in plant leaves. Experimental results showed that SA could induce plant stress resistance under abiotic stress such as high temperature, low temperature, salt stress and heavy metal stress [50].
Seed germination is an important trait affecting plant growth cycle and yield [51]. The germination process of seeds is influenced by multiple hormones, the most important of which are GA and ABA [51]. The application of exogenous GA increases SA biosynthesis in wild Arabidopsis plants or promotes seed germination and seedling growth in the sid2 mutant under adverse conditions, suggesting a synergistic interplay between SA and GA [52]. ET and JA also play vital roles in seed germination. Mutations in positive regulators of ET signaling pathway led to deep dormancy, while negative regulators the CTR1 (CONSTITUTIVE TRIPLE RESPONSE 1) mutations can accelerate seed germination. The effects of JA on seed dormancy and germination seem to be different in different plant species. According to the report, Synergism or antagonism between JA and ABA occurs during germination, as in JA and ABA Synergistic effects occurred during seed germination of Arabidopsis and Brassica napus [53], while JA decreased wheat ABA levels in seeds. SA mainly plays a role in seed germination under stress, although its exact role and the underlying molecular mechanisms have not been fully elucidated [54,55].
Seed germination is usually affected by a variety of abiotic stresses (drought, high temperature, low temperature, salt stress, etc.) [56]. Yang et al. [57] proposed to the effects of different SA gradients and MeSA saturation concentrations on germination, initial growth and other indicators of Arabidopsis thaliana seeds using wild-type and mutants lacking SA accumulation capacity (nahg) as experimental materials [57]. The results showed that: low SA concentration can promote the germination rate of wild-type in Arabidopsis, but has little effect on SA synthesis mutant. Meanwhile, high SA concentration can inhibit the germination rate of seeds.
SA affects seed germination under high salinity and osmotic stress. We therefore decided to investigate the effects of SA on germination under high salinity [58]. Germination of wild-type seed was significantly delayed by NaCl, with more severe inhibition at higher concentrations, as was previously reported [59]. We examined the effects of SA on germination in the presence of 150 mM NaCl. The results showed that germination was further inhibited when the concentration of SA was 0.2 mM on MS medium. In contrast, lower concentrations of SA (0.01–0.01 mM) reduced the inhibitory effects of high salinity [55,60]. These observations indicate that lower concentrations of SA, which are close to the estimated physiological concentrations of SA in Arabidopsis plants eliminate the inhibitory effects of high salinity on germination [61].
Hamada [62] soaked wheat seed with different concentrations of MeSA and found that it could reduce the inhibition effect of drought, increase the photosynthetic rate of plants, inhibit dark respiration, and promote seedling growth. Generally speaking, SOD (super oxide dismutase) activity under water stress is positively correlated with plant antioxidant capacity. It is reported that under salt stress, 0.7 and 1.4 mM SA could significantly increase the germination rate, germination index and vigor index of wheat seeds, and reduce the plasma membrane permeability of seedling leaves and the damage to cell membrane caused by salt stress [63]. It can improve the activity of SOD, POD (peroxidase) and other protective enzymes in seedling body, alleviate the damage of salt stress to wheat, and improve the adaptability of wheat to salt stress [64,65].
Compared with other plant hormones, relatively little research has been done on the role of SA in plant growth. The Arabidopsis sid2 and SA-depleted nahg transgenic line show increased leaf biomass compared to WT. However, CPR5 (CONSTITUTIVE EXPRESSER of PR 5) and SIZ1 (MIZ1 DOMAIN-CONTAINING LIGASE 1) present the dwarf plant phenotype with a shorter stem and smaller leaves compared to the wild type, mutants showed stunted growth [66]. The mechanism of SA promoting growth may be related to the changes of other growth hormone levels and SA can promote plant growth by changing photosynthesis, transpiration and stomatal conductance under stress.
Under salt stress, plants can induce the production of reactive oxygen species and lipid peroxidation, which are found in tomatoes, wheat and spinach. SA can enhance the antioxidant system of plants, remove excessive reactive oxygen species in cell, reduce lipid oxidation level, improve cell metabolism, and finally alleviate salt stress [67]. Meanwhile, the exogenous application of SA can increase the contents of chlorophyll A and carotenoid in tomato, and also improve the rate of photosynthetic electron transfer.
It was found that under drought stress, the chlorophyll content of wheat increased with the increase of exogenous SA concentration, which further enhanced the photosynthetic rate and stomatal conductance of wheat under drought stress. SA treatment of wheat can enhance the activity of ribulose diphosphate carboxylase and thus enhance the fixation of carbon dioxide [68]. The content of carbon compounds required for plant growth increases, so that plants should not starve to death, and maintain plant growth, dry weight and water content increase.
Flowering in various plant species is regulated by environmental factors, such as night-length in photoperiodic flowering and temperature in vernalization [53]. On the other hand, a SD (short-day) plant such as Pharbitis nil (synonym Ipomoea nil) can be induced to flower under LD (long days) when grew under poor-nutrition [69,70], low temperature or high-intensity light conditions [71]. The flowering induced by these conditions is associated with an increase in PAL activity [72]. Taken together, these facts suggest that the flowering induced by these conditions might be regulated by a common mechanism [73,74]. Poor nutrition, low temperature and high-intensity light can be regarded as stress factors, and PAL activity increases under these stress conditions [75,76]. Timely flowering of plants is the result of the joint regulation of endogenous and exogenous signals, which is of great significance for plant growth and reproductive development [75]. It has been suggested that SA may be related to flower regulation, and Raskin et al. [77] first found that SA exerts a regulatory role as a natural thermogenesis inducer at the flower tip of Alam lily.
In Arabidopsis thaliana, flowering is controlled by complex regulatory networks. 0.5 mM SA enhanced dry weight of root, shoot and nodule, and the number of flower and pods in chickpea, and it also significantly increased photosynthesis and growth in wheat and mung bean [78]. Related studies have shown that under UV-C (ultraviolet-C) light exposure, increased SA level will accelerate flowering, and in short days, NahG transgenic plants lacking SA or eds5/sid1 and sid2 mutants show a significant delayed flowering phenotype, confirming that SA regulate flowering time [39,78].
SA regulation of flowering is important, but its complex regulatory mechanisms have not yet been well studied and key questions such as SA signaling pathways and related hormone regulation of flowering time need to be further answered [79].
Senescence takes place when plants grow to a certain stage or under environmental stress. SA levels increase during plant senescence in Arabidopsis thaliana [80]. Consistent with this observation, mutants with defects in SA synthesis or signal transduction, such as sid2, pad4, npr1, eds5, typically exhibit a pattern of anti-aging that includes delayed aging and decreased necrosis compared to wild-type plants. Buchanan-wollaston et al. [81] analyzed the gene expression profile of senescence in Arabidopsis and found that SA had a general up-regulation process on senescence related genes [82]. In SA synthesis or signal transduction mutants, the expression of these 20% age-related genes was decreased by about two time [83]. These findings suggest that SA might modulate leaf senescence under circumstances such as biotic stress, albeit to a limited extent under normal growth conditions [84].
Most of the genes that show SA-dependent expression during leaf senescence [81] also demonstrate increased expression during senescence in petal and siliques, whereas the other phytohormones cause differential expression profiles in the three plant tissues studied. ET biosynthesis and binding appear to be more important in silique and petal senescence than in leaves (despite the fact that some elements are conserved in the three tissues), while genes linked to auxin (AUX) biosynthesis and response are strongly up regulated in petals but down-regulated in leaves. In contrast, the SA pathway is active in the three tissues during senescence [85].
EIN3 (Ethylene insensitive 3) family protein and its homologous protein EIL1 (EIN3-LIKE1) are key transcription factors in ethylene signaling pathway, which is required for ethylene to promote aging. NPR1 is a core regulatory protein in the SA signaling pathway [86]. As a transcription co-activator, NPR1 interacts with other transcription factors to regulate gene expression. It is found that leaf senescence caused by SA depends on NPR1, EIN3 and EIL1 [87]. Compared with SA or ACC (ethylene precursor 1-aminocyclopropane-1-carboxylic acid), is a non-proteinogenic amino acid produced by ACS (ACC SYNTHASE) treatment alone, SA and ACC treatment dramatically promoted leaf senescence, suggesting that SA and ET can synergistically promote leaf senescence [88].
It was found that under salt stress, 0.1 and 0.2 mM SA could significantly increase germination rate and vigor index of wheat seeds, reduce plasma membrane permeability, and improve the activities of SOD, POD and other cell protective enzymes in seedlings [89]. The two enzymes can be eliminated to some extent, salt stress induced intracellular ROS (reactive oxygen species), prevent membrane lipid peroxide in the cells, maintain the cytoplasm membrane stability and integrity, reduce a product of membrane lipid peroxidation malondialdehyde accumulation, and alleviate the harm of salt stress on wheat, improve its adaptability to salt stress [90]. Exogenous SA may enhance plant resistance by inducing the expression of defense genes and alleviating heavy metal toxicity in plants [91]. Mishra et al. [92] showed that exogenous SA could prevent membrane lipid peroxidation. Under the stress of 10 mM PbCl2 or HgCl2, the plasma membrane disintegration of kidney bean could be alleviated by external application of 100 mM SA. PR2 protein was induced by 10 mM SA. Therefore, the mechanism of SA induced PRP was similar to that of plant resistance. The endogenous SA level of plants subjected to heavy metal stress also increased, and the tolerance of plants to heavy metals was enhanced. However, it is not clear whether plants that are resistant to heavy metal stress also regulate PR2 gene transcription by increasing endogenous SA level. A recent study proved that the levels of endogenous SA and SAG in tobacco were increased under heat stress [93]. Studies have shown that Arabidopsis plants grew in SA culture medium containing 10 μmM experienced heat shock stress under 40°C for 1 h, compared with plants without SA treatment, survival rate increased 5 times, electrolyte leakage rate decreased 50%; The survival rate of nahg was close to zero at 37°C for 1 h, which was much lower than that of wild type [94].
SA is a key plant hormone required for establishing resistance to various pathogens [12]. SA biosynthesis involves two main metabolic pathways with multiple steps: the isochorismate and the phenylalanine ammonia-lyase pathways. However, more in-depth studies of both pathways in different species are still required to address the question of how SA bio-synthesis evolved in plants. As one of the most important enzymes in SA biosynthesis, ICS1 seems to be resilient to a broad range of pH values and temperatures for its function. Thus, it is of great significance to study the mechanism of the ICS1 and the optimum conditions of its catalysis for the synthesis of SA and the function of SA signal in plants. Transcriptional regulations of SA biosynthesis are essential for fine tuning of SA level in plants [95]. The complexity of SA-mediated signaling in growth, development and stress management has attracted increasing attention from scientists under diverse environmental conditions and with the dramatic changes in the environment observed with climate change [96]. Although much work has been done on the immunity of plants to pathogens, and the synthesis and signal transduction of SA are well understood, the specific mechanism of SA in regulating crop growth and development and its response to the natural environment are rarely studied [55]. Therefore, further attention and research should be paid to the following areas: (1) The function of SA in each node of plant growth, especially its influence on energy crops, is of great importance to improve crop yield. (2) SA plays a significant role in plants’ response to pathogen stress, which is often called the aspirin of the plant world. Then what function and specific mechanism does SA play in plants’ response to abiotic stress? (3) The hormone network in plants is complex, and the interaction between hormones may influence the whole life process of plants. Therefore, what kind of relationship between SA and other hormones can jointly regulate the growth and development of plants is a very meaningful topic. (4) It must be noted the higher accumulation of endogenous SA enhances plant immunity but generally suppresses growth. Therefore, under the condition of ensuring the normal growth of plants, how to maximize the promotion of plant resistance to adverse environment? The balance between plant growth and stress defense is of great significance for promoting the development of agricultural production.
Author Contributions: The authors confirm contribution to the paper as follows: Ping Li and Xiangyang Hu designed the research and editing the manuscript together. Yulan Hu and Lulu Zhi obtained the related references and wrote the manuscript together. John T. Hancock contributed to refining the ideas and finalizing this paper. All authors reviewed the results and approved the final version of the manuscript.
Funding Statement: This work was funded by the National Natural Science Foundation of China (Grant No. 31970289).
Conflicts of Interest: The authors declare that they have no conflicts of interest to report regarding the present study.
References
1. Arif, Y., Sami, F., Siddiqui, H., Bajguz, A., Hayat, S. (2020). Salicylic acid in relation to other phytohormones in plant: A study towards physiology and signal transduction under challenging environment. Environmental and Experimental Botany, 175, 104040. DOI 10.1016/j.envexpbot.2020.104040. [Google Scholar] [CrossRef]
2. Han, S. K., Wu, M. F., Cui, S., Wagner, D. (2015). Roles and activities of chromatin remodeling ATPases in plants. The Plant Journal, 83(1), 62–77. DOI 10.1111/tpj.12877. [Google Scholar] [CrossRef]
3. Achard, P., Cheng, H., de Grauwe, L., Decat, J., Schoutteten, H. et al. (2006). Integration of plant responses to environmentally activated phytohormonal signals. Science, 311(5757), 91–94. DOI 10.1126/science.1118642. [Google Scholar] [CrossRef]
4. Acharya, B. R., Assmann, S. M. (2009). Hormone interactions in stomatal function. Plant Molecular Biology, 69(4), 451–462. DOI 10.1007/s11103-008-9427-0. [Google Scholar] [CrossRef]
5. Davies, P. J. (2010). The plant hormones: Their nature, occurrence, and functions. In: Davies, P. J. (Ed.Plant hormones, pp. 1–15. Netherland, Dordrecht: Springer. [Google Scholar]
6. Rocher, F., Chollet, J. F., Legros, S., Jousse, C., Lemoine, R. et al. (2009). Salicylic acid transport in Ricinus communis involves a pH-dependent carrier system in addition to diffusion. Plant Physiology, 150(4), 2081–2091. DOI 10.1104/pp.109.140095. [Google Scholar] [CrossRef]
7. Rivas-San Vicente, M., Plasencia, J. (2011). Salicylic acid beyond defence: Its role in plant growth and development. Journal of Experimental Botany, 62(10), 3321–3338. DOI 10.1093/jxb/err031. [Google Scholar] [CrossRef]
8. An, C., Mou, Z. (2011). Salicylic acid and its function in plant immunity. Journal of Integrative Plant Biology, 53(6), 412–428. DOI 10.1111/j.1744-7909.2011.01043.x. [Google Scholar] [CrossRef]
9. Anjum, N. A., Gill, S. S., Khan, I., Gill, R. (2014). Environmental change, and plant amino acids and their derivatives—An introduction. In: Plant adaptation to environmental change: Significance of amino acids and their derivatives, pp. 1–17. UK, Wallingford: CABI. [Google Scholar]
10. Lefevere, H., Bauters, L., Gheysen, G. (2020). Salicylic acid biosynthesis in plants. Frontiers in Plant Science, 11, 338. DOI 10.3389/fpls.2020.00338. [Google Scholar] [CrossRef]
11. Gautam, J. K., Giri, M. K., Singh, D., Chattopadhyay, S., Nandi, A. K. (2021). MYC2 influences salicylic acid biosynthesis and defense against bacterial pathogens in Arabidopsis thaliana. Physiologia Plantarum, 173(4), 2248–2261. DOI 10.1111/ppl.13575. [Google Scholar] [CrossRef]
12. Ding, P., Ding, Y. (2020). Stories of salicylic acid: A plant defense hormone. Trends in Plant Science, 25(6), 549–565. DOI 10.1016/j.tplants.2020.01.004. [Google Scholar] [CrossRef]
13. Rekhter, D., Lüdke, D., Ding, Y., Feussner, K., Zienkiewicz, K. et al. (2019). Isochorismate-derived biosynthesis of the plant stress hormone salicylic acid. Science, 365(6452), 498–502. DOI 10.1126/science.aaw1720. [Google Scholar] [CrossRef]
14. Torrens-Spence, M. P., Bobokalonova, A., Carballo, V., Glinkerman, C. M., Pluskal, T. et al. (2019). PBS3 and EPS1 complete salicylic acid biosynthesis from isochorismate in Arabidopsis. Molecular Plant, 12(12), 1577–1586. DOI 10.1016/j.molp.2019.11.005. [Google Scholar] [CrossRef]
15. Klämbt, H. (1962). Conversion in plants of benzoic acid to salicylic acid and its βd-glucoside. Nature, 196(4853), 491–491. DOI 10.1038/196491a0. [Google Scholar] [CrossRef]
16. Zheng, Z., Qualley, A., Fan, B., Dudareva, N., Chen, Z. (2009). An important role of a BAHD acyl transferase-like protein in plant innate immunity. The Plant Journal, 57(6), 1040–1053. DOI 10.1111/j.1365-313X.2008.03747.x. [Google Scholar] [CrossRef]
17. Klessig, D. F., Choi, H. W., Dempsey, D. M. A. (2018). Systemic acquired resistance and salicylic acid: Past, present, and future. Molecular Plant-Microbe Interactions, 31(9), 871–888. DOI 10.1094/MPMI-03-18-0067-CR. [Google Scholar] [CrossRef]
18. Huang, J., Gu, M., Lai, Z., Fan, B., Shi, K. et al. (2010). Functional analysis of the Arabidopsis PAL gene family in plant growth, development, and response to environmental stress. Plant Physiology, 153(4), 1526–1538. DOI 10.1104/pp.110.157370. [Google Scholar] [CrossRef]
19. Akasha, A., Ashraf, M., Shereen, A., Mahboob, W., Faisal, S. (2019). Heat tolerance screening studies and evaluating salicylic acid efficacy against high temperature in rice (Oryza sativa L.) genotypes. Journal of Plant Biochem & Physiology, 7(2). DOI 10.35248/2329-9029.19.7.235. [Google Scholar] [CrossRef]
20. Dean, J. V., Delaney, S. P. (2008). Metabolism of salicylic acid in wild-type, UGT74F1 and UGT74F2 glucosyltransferase mutants of Arabidopsis thaliana. Physiologia Plantarum, 132(4), 417–425. DOI 10.1111/j.1399-3054.2007.01041.x. [Google Scholar] [CrossRef]
21. George Thompson, A. M., Iancu, C. V., Neet, K. E., Dean, J. V., Choe, J. (2017). Differences in salicylic acid glucose conjugations by UGT74F1 and UGT74F2 from Arabidopsis thaliana. Scientific Reports, 7(1), 1–11. DOI 10.1038/srep46629. [Google Scholar] [CrossRef]
22. Chen, F., D’Auria, J. C., Tholl, D., Ross, J. R., Gershenzon, J. et al. (2003). An Arabidopsis thaliana gene for methylsalicylate biosynthesis, identified by a biochemical genomics approach, has a role in defense. The Plant Journal, 36(5), 577–588. DOI 10.1046/j.1365-313X.2003.01902.x. [Google Scholar] [CrossRef]
23. Wang, B., Wang, S., Wang, Z. (2017). Genome-wide comprehensive analysis the molecular phylogenetic evaluation and tissue-specific expression of SABATH gene family in Salvia miltiorrhiza. Genes, 8(12), 365. DOI 10.3390/genes8120365. [Google Scholar] [CrossRef]
24. Vlot, A. C., Liu, P. P., Cameron, R. K., Park, S. W., Yang, Y. et al. (2008). Identification of likely orthologs of tobacco salicylic acid-binding protein 2 and their role in systemic acquired resistance in Arabidopsis thaliana. The Plant Journal, 56(3), 445–456. DOI 10.1111/j.1365-313X.2008.03618.x. [Google Scholar] [CrossRef]
25. Park, S. W., Kaimoyo, E., Kumar, D., Mosher, S., Klessig, D. F. (2007). Methyl salicylate is a critical mobile signal for plant systemic acquired resistance. Science, 318(5847), 113–116. DOI 10.1126/science.1147113. [Google Scholar] [CrossRef]
26. Rasmussen, J. B., Hammerschmidt, R., Zook, M. N. (1991). Systemic induction of salicylic acid accumulation in cucumber after inoculation with Pseudomonas syringae pv syringae. Plant Physiology, 97(4), 1342–1347. DOI 10.1104/pp.97.4.1342. [Google Scholar] [CrossRef]
27. Vernooij, B., Friedrich, L., Morse, A., Reist, R., Kolditz-Jawhar, R. et al. (1994). Salicylic acid is not the translocated signal responsible for inducing systemic acquired resistance but is required in signal transduction. The Plant Cell, 6(7), 959–965. DOI 10.2307/3870006. [Google Scholar] [CrossRef]
28. Molders, W., Buchala, A., Metraux, J. P. (1996). Transport of salicylic acid in tobacco necrosis virus-infected cucumber plants. Plant Physiology, 112(2), 787–792. DOI 10.1104/pp.112.2.787. [Google Scholar] [CrossRef]
29. de Vleesschauwer, D., Xu, J., Höfte, M. (2014). Making sense of hormone-mediated defense networking: From rice to Arabidopsis. Frontiers in Plant Science, 5, 611. DOI 10.3389/fpls.2014.00611. [Google Scholar] [CrossRef]
30. Franden, M. A., Pilath, H. M., Mohagheghi, A., Pienkos, P. T., Zhang, M. (2013). Inhibition of growth of Zymomonas mobilis by model compounds found in lignocellulosic hydrolysates. Biotechnology for Biofuels, 6(1), 1–15. DOI 10.1186/1754-6834-6-99. [Google Scholar] [CrossRef]
31. Nawrath, C., Métraux, J. P. (1999). Salicylic acid induction-deficient mutants of Arabidopsis express PR-2 and PR-5 and accumulate high levels of camalexin after pathogen inoculation. The Plant Cell, 11(8), 1393–1404. DOI 10.2307/3870970. [Google Scholar] [CrossRef]
32. Wildermuth, M. C., Dewdney, J., Wu, G., Ausubel, F. M. (2001). Isochorismate synthase is required to synthesize salicylic acid for plant defence. Nature, 414(6863), 562–565. DOI 10.1038/35107108. [Google Scholar] [CrossRef]
33. Xun, H., Yang, X., He, H., Wang, M., Guo, P. et al. (2019). Over-expression of GmKR3, a TIR–NBS–LRR type R gene, confers resistance to multiple viruses in soybean. Plant Molecular Biology, 99(1), 95–111. DOI 10.1007/s11103-018-0804-z. [Google Scholar] [CrossRef]
34. Guo, B., Liang, Y., Zhu, Y., Zhao, F. (2007). Role of salicylic acid in alleviating oxidative damage in rice roots (Oryza sativa) subjected to cadmium stress. Environmental Pollution, 147(3), 743–749. DOI 10.1016/j.envpol.2006.09.007. [Google Scholar] [CrossRef]
35. Hossain, M. A., Bhattacharjee, S., Armin, S. M., Qian, P., Xin, W. et al. (2015). Hydrogen peroxide priming modulates abiotic oxidative stress tolerance: Insights from ROS detoxification and scavenging. Frontiers in Plant Science, 6, 420. DOI 10.3389/fpls.2015.00420. [Google Scholar] [CrossRef]
36. Zschiesche, W., Barth, O., Daniel, K., Böhme, S., Rausche, J. et al. (2015). The zinc-binding nuclear protein HIPP 3 acts as an upstream regulator of the salicylate-dependent plant immunity pathway and of flowering time in Arabidopsis thaliana. New Phytologist, 207(4), 1084–1096. DOI 10.1111/nph.13419. [Google Scholar] [CrossRef]
37. Larkindale, J., Hall, J. D., Knight, M. R., Vierling, E. (2005). Heat stress phenotypes of Arabidopsis mutants implicate multiple signaling pathways in the acquisition of thermotolerance. Plant Physiology, 138(2), 882–897. DOI 10.1104/pp.105.062257. [Google Scholar] [CrossRef]
38. Backer, R., Mahomed, W., Reeksting, B. J., Engelbrecht, J., Ibarra-Laclette, E. et al. (2015). Phylogenetic and expression analysis of the NPR1-like gene family from Persea americana (Mill.). Frontiers in Plant Science, 6, 300. DOI 10.3389/fpls.2015.00300. [Google Scholar] [CrossRef]
39. Pokotylo, I., Kravets, V., Ruelland, E. (2019). Salicylic acid binding proteins (SABPsThe hidden forefront of salicylic acid signalling. International Journal of Molecular Sciences, 20(18), 4377. DOI 10.3390/ijms20184377. [Google Scholar] [CrossRef]
40. Martínez, C., Pons, E., Prats, G., León, J. (2004). Salicylic acid regulates flowering time and links defence responses and reproductive development. The Plant Journal, 37(2), 209–217. DOI 10.1046/j.1365-313X.2003.01954.x. [Google Scholar] [CrossRef]
41. Li, N., Han, X., Feng, D., Yuan, D., Huang, L. J. (2019). Signaling crosstalk between salicylic acid and ethylene/jasmonate in plant defense: Do we understand what they are whispering? International Journal of Molecular Sciences, 20(3), 671. DOI 10.3390/ijms20030671. [Google Scholar] [CrossRef]
42. Chen, J., Mohan, R., Zhang, Y., Li, M., Chen, H. et al. (2019). NPR1 promotes its own and target gene expression in plant defense by recruiting CDK8. Plant Physiology, 181(1), 289–304. DOI 10.1104/pp.19.00124. [Google Scholar] [CrossRef]
43. Pathak, M. R., Teixeira da Silva, J. A., Wani, S. H. (2014). Polyamines in response to abiotic stress tolerance through transgenic approaches. GM Crops & Food: Biotechnology in Agriculture and the Food Chain, 5(2), 87–96. DOI 10.4161/gmcr.28774. [Google Scholar] [CrossRef]
44. Ding, Y., Sun, T., Ao, K., Peng, Y., Zhang, Y. et al. (2018). Opposite roles of salicylic acid receptors NPR1 and NPR3/NPR4 in transcriptional regulation of plant immunity. Cell, 173(6), 1454–1467. DOI 10.1016/j.cell.2018.03.044. [Google Scholar] [CrossRef]
45. Castelló, M. J., Medina-Puche, L., Lamilla, J., Tornero, P. (2018). NPR1 paralogs of Arabidopsis and their role in salicylic acid perception. PLoS One, 13(12), e0209835. DOI 10.1371/journal.pone.0209835. [Google Scholar] [CrossRef]
46. Senaratna, T., Touchell, D., Bunn, E., Dixon, K. (2000). Acetyl salicylic acid (Aspirin) and salicylic acid induce multiple stress tolerance in bean and tomato plants. Plant Growth Regulation, 30(2), 157–161. DOI 10.1023/A:1006386800974. [Google Scholar] [CrossRef]
47. Skelly, M. J., Furniss, J. J., Grey, H., Wong, K. W., Spoel, S. H. (2019). Dynamic ubiquitination determines transcriptional activity of the plant immune coactivator NPR1. elife, 8, e47005. DOI 10.7554/eLife.47005. [Google Scholar] [CrossRef]
48. Shan, D., Wang, C., Zheng, X., Hu, Z., Zhu, Y. et al. (2021). MKK4-MPK3-WRKY17-mediated salicylic acid degradation increases susceptibility to Glomerella leaf spot in apple. Plant Physiology, 186(2), 1202–1219. DOI 10.1093/plphys/kiab108. [Google Scholar] [CrossRef]
49. Deng, B., Wang, W., Ruan, C., Deng, L., Yao, S. et al. (2020). Involvement of CsWRKY70 in salicylic acid-induced citrus fruit resistance against Penicillium digitatum. Horticulture Research, 7, 157. DOI 10.1038/s41438-020-00377-y. [Google Scholar] [CrossRef]
50. van Butselaar, T., van den Ackerveken, G. (2020). Salicylic acid steers the growth-immunity tradeoff. Trends in Plant Science, 25(6), 566–576. DOI 10.1016/j.tplants.2020.02.002. [Google Scholar] [CrossRef]
51. Wei, W., Hu, Y., Yang, W., Li, X., Wei, J. et al. (2021). S-nitrosoglutathion reductase activity modulates the thermotolerance of seeds germination by controlling ABI5 stability under high temperature. Phyton-International Journal of Experimental Botany, 90(4), 1075–1087. DOI 10.32604/phyton.2021.016134. [Google Scholar] [CrossRef]
52. Seo, D. H., Jeong, H., Choi, Y. D., Jang, G. (2021). Auxin controls the division of root endodermal cells. Plant Physiology, 187(3), 1577–1586. DOI 10.1093/plphys/kiab341. [Google Scholar] [CrossRef]
53. Shinozaki, M., Takimoto, A. (1982). The role of cotyledons in flower initiation of Pharbitis nil at low temperatures. Plant and Cell Physiology, 23(3), 403–408. DOI 10.1093/oxfordjournals.pcp.a076362. [Google Scholar] [CrossRef]
54. Szalai, G., Páldi, E., Janda, T. (2005). Effect of salt stress on the endogenous salicylic acid content in maize (Zea mays L.) plants. Acta Biologica Szegediensis, 49(1–2), 47–48. [Google Scholar]
55. Lee, S., Kim, S. G., Park, C. M. (2010). Salicylic acid promotes seed germination under high salinity by modulating antioxidant activity in Arabidopsis. New Phytologist, 188(2), 626–637. DOI 10.1111/j.1469-8137.2010.03378.x. [Google Scholar] [CrossRef]
56. Li, L., Yan, X., Li, J., Tian, Y., Pan, R. (2021). Advances in cotton tolerance to heavy metal stress and applications to remediate heavy metal-contaminated farmland soil. Phyton-International Journal of Experimental Botany, 90(1), 35–50. DOI 10.32604/phyton.2021.012276. [Google Scholar] [CrossRef]
57. Yang, Y., Qi, M., Mei, C. (2004). Endogenous salicylic acid protects rice plants from oxidative damage caused by aging as well as biotic and abiotic stress. The Plant Journal, 40(6), 909–919. DOI 10.1111/j.1365-313X.2004.02267.x. [Google Scholar] [CrossRef]
58. Borsani, O., Valpuesta, V., Botella, M. A. (2001). Evidence for a role of salicylic acid in the oxidative damage generated by NaCl and osmotic stress in Arabidopsis seedlings. Plant Physiology, 126(3), 1024–1030. DOI 10.1104/pp.126.3.1024. [Google Scholar] [CrossRef]
59. Rajjou, L., Belghazi, M., Huguet, R., Robin, C., Moreau, A. et al. (2006). Proteomic investigation of the effect of salicylic acid on Arabidopsis seed germination and establishment of early defense mechanisms. Plant Physiology, 141(3), 910–923. DOI 10.1104/pp.106.082057. [Google Scholar] [CrossRef]
60. Sarath, G., Hou, G., Baird, L. M., Mitchell, R. B. (2007). Reactive oxygen species, ABA and nitric oxide interactions on the germination of warm-season C4-grasses. Planta, 226(3), 697–708. DOI 10.1007/s00425-007-0517-z. [Google Scholar] [CrossRef]
61. Preston, J., Tatematsu, K., Kanno, Y., Hobo, T., Kimura, M. et al. (2009). Temporal expression patterns of hormone metabolism genes during imbibition of Arabidopsis thaliana seeds: A comparative study on dormant and non-dormant accessions. Plant and Cell Physiology, 50(10), 1786–1800. DOI 10.1093/pcp/pcp121. [Google Scholar] [CrossRef]
62. Hamada, A. (1998). Effects of exogenously added ascorbic acid, thiamin or aspirin on photosynthesis and some related activities of drought-stressed wheat plants. In: Garab, G. (Ed.Photosynthesis: Mechanisms and effects, pp. 2581–2584. Netherland, Dordrecht: Springer. [Google Scholar]
63. Sui, N., Tian, S., Wang, W., Wang, M., Fan, H. (2017). Overexpression of glycerol-3-phosphate acyltransferase from suaeda salsa improves salt tolerance in Arabidopsis. Frontiers in Plant Science, 8, 1337. DOI 10.3389/fpls.2017.01337. [Google Scholar] [CrossRef]
64. Pouramir-Dashtmian, F., Khajeh-Hosseini, M., Esfahani, M. (2014). Improving chilling tolerance of rice seedling by seed priming with salicylic acid. Archives of Agronomy and Soil Science, 60(9), 1291–1302. DOI 10.1080/03650340.2014.892584. [Google Scholar] [CrossRef]
65. Su, D., Chen, N. L., Gao, T. P., Wang, C. Y., Sheng, H. M. et al. (2012). Effects of Si+, K+ and Ca2+ on antioxidant enzyme activities and osmolytes in halocnemum strobilaceum under salt stress. Advanced Materials Research, 356–360, 2542–2550. DOI 10.4028/www.scientific.net/AMR.356-360.2542. [Google Scholar] [CrossRef]
66. Kim, J. Y., Song, J. T., Seo, H. S. (2021). Ammonium-mediated reduction in salicylic acid content and recovery of plant growth in Arabidopsis siz1 mutants is modulated by NDR1 and NPR1. Plant Signaling & Behavior, 16(9), 1928819. DOI 10.1080/15592324.2021.1928819. [Google Scholar] [CrossRef]
67. Santner, A., Calderon-Villalobos, L. I. A., Estelle, M. (2009). Plant hormones are versatile chemical regulators of plant growth. Nature Chemical Biology, 5(5), 301–307. DOI 10.1038/nchembio.165. [Google Scholar] [CrossRef]
68. Tan, S., Abas, M., Verstraeten, I., Glanc, M., Molnár, G. et al. (2020). Salicylic acid targets protein phosphatase 2A to attenuate growth in plants. Current Biology, 30(3), 381–395. e388. DOI 10.1016/j.cub.2019.11.058. [Google Scholar] [CrossRef]
69. Shinozaki, M., Hikichi, M., Yoshida, K., Watanabe, K., Takimoto, A. (1982). Effect of high-intensity light given prior to low-temperature treatment on the long-day flowering of Pharbitis nil. Plant and Cell Physiology, 23(3), 473–477. DOI 10.1093/oxfordjournals.pcp.a076371. [Google Scholar] [CrossRef]
70. Wada, K. C., Yamada, M., Shiraya, T., Takeno, K. (2010). Salicylic acid and the flowering gene FLOWERING LOCUS T homolog are involved in poor-nutrition stress-induced flowering of Pharbitis nil. Journal of Plant Physiology, 167(6), 447–452. DOI 10.1016/j.jplph.2009.10.006. [Google Scholar] [CrossRef]
71. Shinozaki, M., Asada, K., Takimoto, A. (1988). Correlation between chlorogenic acid content in cotyledons and flowering in Pharbitis seedlings under poor nutrition. Plant and Cell Physiology, 29(4), 605–609. [Google Scholar]
72. Dixon, R. A., Paiva, N. L. (1995). Stress-induced phenylpropanoid metabolism. The Plant Cell, 7(7), 1085–1097. DOI 10.2307/3870059. [Google Scholar] [CrossRef]
73. Hirai, N., Kuwano, Y., Kojima, Y., Koshimizu, K., Shinozaki, M. et al. (1995). Increase in the activity of phenylalanine ammonia-lyase during the non-photoperiodic induction of flowering in seedlings of morning glory (Pharbitis nil). Plant and Cell Physiology, 36(2), 291–297. DOI 10.1093/oxfordjournals.pcp.a078761. [Google Scholar] [CrossRef]
74. Xu, M. Y., Zhang, L., Li, W. W., Hu, X. L., Wang, M. B. et al. (2014). Stress-induced early flowering is mediated by miR169 in Arabidopsis thaliana. Journal of Experimental Botany, 65(1), 89–101. DOI 10.1093/jxb/ert353. [Google Scholar] [CrossRef]
75. Hirai, N., Yamamuro, M., Koshimizu, K., Shinozaki, M., Takimoto, A. (1994). Accumulation of phenylpropanoids in the cotyledons of morning glory (Pharbitis nil) seedlings during the induction of flowering by low temperature treatment, and the effect of precedent exposure to high-intensity light. Plant and Cell Physiology, 35(4), 691–695. DOI 10.1093/oxfordjournals.pcp.a078644. [Google Scholar] [CrossRef]
76. Wada, K., Takeno, K. (2013). Salicylic acid-mediated stress-induced flowering. In: Hayat, S., Ahmad, A., Alyemeni, M. (Eds.Salicylic acid, pp. 163–182. Netherland, Dordrecht: Springer. [Google Scholar]
77. Leon, J., Shulaev, V., Yalpani, N., Lawton, M. A., Raskin, I. (1995). Benzoic acid 2-hydroxylase, a soluble oxygenase from tobacco, catalyzes salicylic acid biosynthesis. PNAS, 92(22), 10413–10417. DOI 10.1073/pnas.92.22.10413. [Google Scholar] [CrossRef]
78. Kaur, H., Hussain, S. J., Kaur, G., Poor, P., Alamri, S. et al. (2022). Salicylic acid improves nitrogen fixation, growth, yield and antioxidant defence mechanisms in chickpea genotypes under salt stress. Journal of Plant Growth Regulation, 41, 2043–2047. DOI 10.1007/s00344-022-10592-7. [Google Scholar] [CrossRef]
79. Lu, P., Magwanga, R. O., Lu, H., Kirungu, J. N., Wei, Y. et al. (2018). A novel G-protein-coupled receptors gene from upland cotton enhances salt stress tolerance in transgenic Arabidopsis. Genes, 9(4), 209. DOI 10.3390/genes9040209. [Google Scholar] [CrossRef]
80. Seguel, A., Jelenska, J., Herrera-Vásquez, A., Marr, S. K., Joyce, M. B. et al. (2018). PROHIBITIN3 forms complexes with ISOCHORISMATE SYNTHASE1 to regulate stress-induced salicylic acid biosynthesis in Arabidopsis. Plant Physiology, 176(3), 2515–2531. DOI 10.1104/pp.17.00941. [Google Scholar] [CrossRef]
81. Buchanan-Wollaston, V., Page, T., Harrison, E., Breeze, E., Lim, P. O. et al. (2005). Comparative transcriptome analysis reveals significant differences in gene expression and signalling pathways between developmental and dark/starvation-induced senescence in Arabidopsis. The Plant Journal, 42(4), 567–585. DOI 10.1111/j.1365-313X.2005.02399.x. [Google Scholar] [CrossRef]
82. Zhang, Y., Li, X. (2019). Salicylic acid: Biosynthesis, perception, and contributions to plant immunity. Current Opinion in Plant Biology, 50, 29–36. DOI 10.1016/j.pbi.2019.02.004. [Google Scholar] [CrossRef]
83. Wang, Y. H., Irving, H. R. (2011). Developing a model of plant hormone interactions. Plant Signaling & Behavior, 6(4), 494–500. DOI 10.4161/psb.6.4.14558. [Google Scholar] [CrossRef]
84. Zhang, D., Zhu, Z., Gao, J., Zhou, X., Zhu, S. et al. (2021). The NPR1-WRKY46-WRKY6 signaling cascade mediates probenazole/salicylic acid-elicited leaf senescence in Arabidopsis thaliana. Journal of Integrative Plant Biology, 63(5), 924–936. DOI 10.1111/jipb.13044. [Google Scholar] [CrossRef]
85. Lundberg, D. S., Lebeis, S. L., Paredes, S. H., Yourstone, S., Gehring, J. et al. (2012). Defining the core Arabidopsis thaliana root microbiome. Nature, 488(7409), 86–90. DOI 10.1038/nature11237. [Google Scholar] [CrossRef]
86. Zheng, X. Y., Zhou, M., Yoo, H., Pruneda Paz, J. L., Spivey, N. W. et al. (2015). Spatial and temporal regulation of biosynthesis of the plant immune signal salicylic acid. PNAS, 112(30), 9166–9173. DOI 10.1073/pnas.1511182112. [Google Scholar] [CrossRef]
87. Backer, R., Naidoo, S., Van den Berg, N. (2019). The NONEXPRESSOR OF PATHOGENESIS-RELATED GENES 1 (NPR1) and related family: Mechanistic insights in plant disease resistance. Frontiers in Plant Science, 10, 102. DOI 10.3389/fpls.2019.00102. [Google Scholar] [CrossRef]
88. Zhou, Y. C., Zheng, R. L. (1991). Phenolic compounds and an analog as superoxide anion scavengers and anti oxidants. Biochemical Pharmacology, 42(6), 1177–1179. DOI 10.1016/0006-2952(91)90251-Y. [Google Scholar] [CrossRef]
89. Zhang, H., Yang, Z., You, X., Heng, Y., Wang, Y. (2021). The potassium transporter AtKUP12 enhances tolerance to salt stress through the maintenance of the K+/Na+ ratio in Arabidopsis. Phyton-International Journal of Experimental Botany, 90(2), 389–402. DOI 10.32604/phyton.2021.014156. [Google Scholar] [CrossRef]
90. Alshoaibi, A. (2021). Improved tolerance of three Saudi pearl millet cultivars (Pennisetum spicatum) to salt stress by mycorrhiza. Phyton-International Journal of Experimental Botany, 90(3), 731–745. DOI 10.32604/phyton.2021.015476. [Google Scholar] [CrossRef]
91. Alharby, H. F., Hasanuzzaman, M., Al Zahrani, H. S., Hakeem, K. R. (2021). Exogenous selenium mitigates salt stress in soybean by improving growth, physiology, glutathione homeostasis and antioxidant defense. Phyton-International Journal of Experimental Botany, 90(2), 373–388. DOI 10.32604/phyton.2021.013657. [Google Scholar] [CrossRef]
92. Mishra, A., Choudhuri, M. (1999). Effects of salicylic acid on heavy metal-induced membrane deterioration mediated by lipoxygenase in rice. Biologia Plantarum, 42(3), 409–415. DOI 10.1023/A:1002469303670. [Google Scholar] [CrossRef]
93. Li, X., Liao, F., Ma, Q., Jiang, B., Pan, Y. et al. (2021). Proteomic analysis of high temperature stress-responsive proteins in Chrysanthemum leaves. Phyton-International Journal of Experimental Botany, 90(5), 1415–1423. DOI 10.32604/phyton.2021.016143. [Google Scholar] [CrossRef]
94. Zahoor, A., Waraich, E. A., Rehman, M. Z. U., Ayub, M. A., Usman, M. et al. (2021). Foliar application of phosphorus enhances photosynthesis and biochemical characteristics of maize under drought stress. Phyton-International Journal of Experimental Botany, 90(2), 503–514. DOI 10.32604/phyton.2021.013588. [Google Scholar] [CrossRef]
95. Raskin, I. (1992). Salicylate, a new plant hormone. Plant Physiology, 99(3), 799–803. DOI 10.1104/pp.99.3.799. [Google Scholar] [CrossRef]
96. Sugano, S., Jiang, C. J., Miyazawa, S. I., Masumoto, C., Yazawa, K. et al. (2010). Role of OsNPR1 in rice defense program as revealed by genome-wide expression analysis. Plant Molecular Biology, 74(6), 549–562. DOI 10.1007/s11103-010-9695-3. [Google Scholar] [CrossRef]
Cite This Article
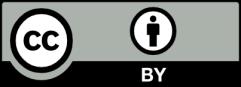
This work is licensed under a Creative Commons Attribution 4.0 International License , which permits unrestricted use, distribution, and reproduction in any medium, provided the original work is properly cited.