Open Access
ARTICLE
Benzyl Amino Purine and Gibberellic Acid Coupled to Nitrogen-Limited Stress Induce Fatty Acids, Biomass Accumulation, and Gene Expression in Scenedesmus Obliquus
1 Departamento de Química, Centro de Ciencias Básicas, Universidad Autónoma de Aguascalientes, Aguascalientes, 20131, México
2 Facultad de Ciencias Químicas, Universidad Autónoma de San Luis Potosí, San Luis Potosí, 78210, México
3 Instituto Politécnico Nacional, Unidad Profesional Interdisciplinaria de Ingeniería Campus Zacatecas, Blvd. del Bote 202, Cerro del
Gato Ejido La Escondida, Col. Ciudad Administrativa, Zacatecas, ZAC 98160, Mexico
* Corresponding Author: José Francisco Morales-Domínguez. Email:
Phyton-International Journal of Experimental Botany 2021, 90(2), 515-531. https://doi.org/10.32604/phyton.2021.013619
Received 13 August 2020; Accepted 15 October 2020; Issue published 07 February 2021
Abstract
The need for renewable energy sources makes microalgae an essential feedstock for biofuels production. The molecular aspects and the response to nitrogen (N)-limited conditions with a phytohormone stimulus in microalgae have been slightly explored. In this work, Scenedesmus obliquus was used as a study model to analyze the effect of benzyl amino purine (BAP) and gibberellic acid (GA) coupled to nitrogen limitation on cell growth, biomass and fatty acids. The selected 10-5 M BAP increased the biomass by 1.44-fold, and 10-6 M GA by 1.35-fold. The total lipids also increased by 2.8 and 1.11-fold, respectively. The 10-5 M BAP and10-6 M GA addition to S. obliquus cultures at different initial nitrogen percentages (N-0, N-25, and N-50) showed a significant increase in cell growth and biomass productivity compared to the unstimulated cultures. BAP N-0 and GA N-0 produced the highest lipid yields with 55% and 50%, respectively. The lipid profile analysis revealed an increase, particularly in C18:1 and C16:0 fatty acids. Gene expression analysis showed an over-expression of acyl carrying protein (ACP), stearoyl-ACP desaturase (SAD), fatty acid acyl-ACP thioesterase (FATA), and diacylglycerol acyltransferase (DGAT) genes, which were mainly induced by nitrogen limitation. Furthermore, BAP and GA produced a significant over-expression on these genes in the N-replete cultures. This study shows that BAP and GA, coupled to N limitation stress, can be used to increase the biomass and lipid production in S. obliquus for sustainable biofuels.
Keywords
In recent decades, microalgae have attracted attention due to the wide variety of metabolites that they synthesize, since they are of interest for several industrial sectors, for example: in functional foods, nutraceuticals, pharmaceuticals, cosmetics, aquaculture and biofuels [1,2]. For these purposes, the selection of the appropriate strain and growth conditions are critical.
Scenedesmus obliquus is a microalgae with great potential as a feedstock for biodiesel production; it proliferates fast, has high biomass productivity, and contains the most suitable fatty acid profile for biodiesel production [3]. In several species of microalgae, the nutrient limitation, mainly nitrogen (N), has shown to be a resource capable of modifying the pattern of carbon storage favoring the triacylglycerol (TAG) formation [4]. However, N deficiency slows down cell division and growth; therefore, biodiesel production’s cost-benefit is affected [5]. It has been reported that the supplementation of exogenous phytohormones such as abscisic acid (ABA), 6-benzyl amino purine (BAP), gibberellic acid (GA) and indole-acetic acid (IAA), among others, increase the microalgae reproduction, and stimulate the production and accumulation of lipids [6].
Phytohormones are chemical messengers that stimulate and regulate cell growth and division in plants and microalgae [7]. Phytohormone supplementation in microalgae cultures under nitrogen limitation has been used as a strategy to increase biomass and lipid biosynthesis in Scenedesmus quadricauda [8] and Acutodesmus dimorphus [9]. However, gene expression analyses in lipid biosynthesis and accumulation have been poorly evaluated. For example, some genes involved in fatty acid and TAG syntheses, such as fatty acid acyl-ACP thioesterase (FATA) and diacylglycerol acyltransferase (DGAT), were over-expressed in Neochloris oleabundans under N-limiting conditions leading to an over-production of fatty acids [10]. In Haematococcus pluvialis, the expression for acyl carrying protein (ACP), FATA, and stearoyl-ACP desaturase (SAD) genes positively correlated with increases in monounsaturated fatty acids (MUFAs), polyunsaturated fatty acids (PUFAs), and oleic acid (C18:1), respectively [11]. In another study, over-expression of ACP, DGAT, and FATA genes, induced by N limitation or high salinity conditions, showed a positive correlation with lipid accumulation in Scenedesmus spp. [12].
In this work, we selected 10-5 M BAP and 10-6 M GA, and studied their effect on growth, biomass productivity, lipid production, and fatty acid profile, in S. obliquus N-limited cultures. Also, the gene expression of four genes involved in the lipid biosynthetic pathway ACP, FATA, SAD, and DGAT was assessed.
S. obliquus (UTEX 3031) was obtained from the algae collection of the University of Texas, USA. Bold’s basal medium (BBM) [13] was used to grow the microalgae. The medium was autoclaved at 121°C for 20 min at 15 psi pressure. S. obliquus was cultured in 1000 mL flasks with 800 mL of sterile BBM at 22 ± 2°C with a 16:8 h light/dark photoperiod, with white light at 76 μmol m-2 s-1. The cultures were aerated (1500 cc/min) using an aquarium air pump to supply CO2 (0.04%). The flasks were shaken twice a day to avoid cell sedimentation. On day 20, this culture was used to inoculate the batch experiments.
2.1 Screening of BAP and GA Concentrations for Improving Growth, Biomass, and Lipid Production in S. obliquus
Both BAP and GA concentrations of 10-5, 10-6, 10-7, 10-8 M were used to obtain the highest cell growth, biomass productivity, and lipid values in S. obliquus. BBM standard was used to grow the microalgae. The cultures cell density was adjusted to an absorbance of 0.2 ± 0.01 measured at an optical density (OD) of 750 nm using a UV-vis spectrophotometer (Thermo ScientificTM GENESYS 10S). Cell growth, biomass, and lipids were measured every 48 h during 15 days in biological triplicate. The flasks were incubated, as mentioned above.
2.2 Effect of BAP and GA on Growth, Biomass, and Lipid Content under N-Reduced Conditions
The concentrations of BAP and GA with the best results were 10-5 M and 10-6 M, respectively. These concentrations were used for the following series of experiments along with different N concentrations: 125 mg L-1, 62.5 mg L-1, and 0 mg L-1 (50%, 25%, and 0% of the total N concentration, to the BBM standard). The incubation conditions were applied identically, as above mentioned.
2.3 Growth and Dry Biomass Determination
Cell density (cells mL-1) was determined by counting with a Bright-Line Hemocytometer (Hausser Scientific) using an Olympus CH30 microscope (Nikon Inc.). The biomass in dry weight (DW) was estimated based on a linear relationship between OD750 and biomass g L-1 DW, which was obtained after a multiple data analysis and was calculated using Eq. (1) [14]:
Biomass (g L−1)=1.6281 (OD750)−0.006 (R2=0.9982) (1)
Biomass productivity (P, g L-1 d-1) were calculated from the Eq. (2):
P=Xt2−Xt1/t2−t1 (2)
where Xt2 and Xt1 are the dry cell weight concentration (g L-1) at time t2 and t1, respectively [15].
2.4 Lipid Quantification and Fatty Acids Analysis
The lipid quantification was performed by the sulpho-phospho-vanillin (SPV) method, according to Park et al. [16] protocol with slight modifications. An aliquot of 0.5 mL of microalgae culture was taken every 48 h. Cells were recovered by centrifugation at 4000 rpm for 5 min, then washed with deionized water and dried at 100°C until constant weight. The dry biomass was used for the SPV reaction and lipid estimation using a GENESYS 10S UV-Vis Spectrophotometer (Thermo Fisher Scientific, USA). For fatty acids analysis, total lipids were extracted by the Blight-Dyer method [17] from 50 mg of biomass harvested on the 15th day of incubation. The fatty acids were transmethylated to fatty acid methyl esters (FAMEs) and analyzed using a Varian CP-3800 gas chromatograph (Varian Inc., Walnut Creek, CA) equipped with a flame ionization detector (FID) using a Stabilwax capillary column (60 m × 0.25 mm internal diameter, 0.25-μm film, Catalog Number 10626, Restek Corp, USA) as previously described by Salas-Montes et al. [18].
2.5 Expression Analysis by qRT-PCR
The S. obliquus cells were recovered after 15 days and freeze-dried. Total RNA extraction was done using the commercial E.Z.N.A.® Kit (Omega Bio-Tek, USA). For cDNA synthesis, the SuperScript III kit and oligo (dT)20 were used (Invitrogen, USA). The reaction mixture was: 1 μL of oligo (dT)20 (50 μM), 10 mM dNTP’s Mix, 1 μg of RNA, 1 μL of SuperScript III, 4 μL of 5 × First-Stand Buffer, and 1 μL DTT (0.1 M). The mixture was incubated at 50°C for 1 h. The cDNA was quantified and diluted to 100 ng cDNA μL-1.
The S. obliquus 18S rRNA gene was used as an internal standard. Details of the oligonucleotides and product size of the DGAT, ACP, FATA, SAD, and ribosomal genes are described in the Appendix A. Real-time PCR (qRT-PCR) was performed on a 96-well optical grade plate using a real-time PCR system from Applied Biosystems StepOneTM (Applied Biosystems, Australia) using RadiantTM Green Lo-ROX qPCR Kit (Alkali Scientific Inc., USA). The program parameters for amplification were 50°C 2 min, 95°C 2.0 min, 40 cycles of 95°C for 5 s and at 60°C for 1.0 min. Melting curves were generated by a cycle of 95°C for 15 s, 60°C for 1 min and 95°C for 30 s. The samples were evaluated in triplicate, and the data were analyzed using Applied Biosystems Step One real-time PCR system software (Applied Biosystems, Mulgrave, AUS). Reference data were obtained from cycles 1 to 40. To normalize the number of transcripts in each sample, the relative abundance of 18S rRNA was also used as the internal standard. Gene expression data were analyzed using the 2-ΔΔCt method [19].
The measurements of growth kinetics, biomass productivity, and gene expression analysis were conducted in triplicate. The analysis of total lipids and fatty acid profile were performed in duplicate. Data was analyzed using Prism 7 for Windows software, version 7.00 (GraphPad Software, Inc.). Significant differences between treatment means for each variable were compared using an analysis of Tukey at a 95% confidence level (p < 0.05) comparing mean values for a data set using the analysis of variance (ANOVA). All SD (standard deviation) values in the graphs were represented as error bars.
3.1 Effect of BAP and GA Concentrations on Cell density, Biomass, and Lipid Production in S. obliquus
During the first five days, the cell number in BAP cultures were similar in all four concentrations (10-5, 10-6, 10-7, and 10-8 M). From the seventh to the fifteenth day, the 10-5 M BAP had the highest cell number with 20.08 × 106 cells mL-1, equivalent to 1.44-fold compared to control (Fig. 1A).
Figure 1: Algal growth rates (A), percentage of lipids (DW) (B), and biomass productivity (mg L-1 d-1) (C), in BAP-stimulated S. obliquus cultures at different concentrations: 10-5 M, 10-6 M, 10-7 M, and 10-8 M. The error bars indicate the standard deviation of the mean. * indicate the significant differences ANOVA (p < 0.05). Tukey’s multiple comparison test was used when the ANOVA detected significant differences (p < 0.05) between each condition. Data with different letters are significantly different. NS = non-significant data
Total lipids were measured as a percent of biomass in DW. In Fig. 1B, 10-5 M BAP increased the lipid production on the fifth day by 2.8-fold, and on the eleventh day, 10-5 M BAP induced the highest lipid percent with 27% (DW), while in control it was 16% (DW). Furthermore, biomass productivity was significantly higher (73.52 mg L-1 d-1) with 10-5 M BAP stimulus compared to the other concentrations (Fig. 1C).
GA (10-6 M) induced the highest cell growth with 16.44 × 106 mL-1 cells, improving the cell number by 1.35-fold more than the control (12.15 × 106 cells mL-1) (Fig. 2A). On the last day of the experiment (day 15), 10-6 M GA produced the highest lipid yield and biomass productivity, with 17.8% (DW) and 59.88 mg L-1 d-1, respectively (Figs. 2B and 2C).
Figure 2: Algal growth rates (A), percentage of lipids (DW) (B), and biomass productivity (mg L-1 d-1) (C), in GA-stimulated S. obliquus cultures at different concentrations: 10-5 M, 10-6 M, 10-7 M, and 10-8 M. The error bars indicate the standard deviation of the mean. * indicate the significant differences ANOVA (p < 0.05). Tukey’s multiple comparison test was used when the ANOVA detected significant differences (p < 0.05) between each condition. Data with different letters are significantly different. NS = non-significant data
These results were used for the following N-limited analyses.
3.2 Effect of BAP and GA on Cell Density, Biomass and Lipid Production in S. obliquus under N-Limited Conditions
In all N-limited and N-replete (controls) assays, 10-5 M BAP and 10-6 M GA increased cell density compared to the non-stimulated cultures. BAP N-100 and GA N-100 had the highest cell number. In N-limited experiments, the most relevant results were in the BAP N-0 and GA N-0 cultures, where cell numbers increased to 6.91 × 106 cells mL-1 and 5.19 × 106 cells mL-1, respectively (Figs. 3A and 4A), two-fold as much as the N-0 cultures without stimulus. Biomass productivity increased to 1.80-fold higher than the N-100 control, with 76.16 mg L-1 d-1 (DW) in BAP N-100 and 73.53 mg L-1 d-1 (DW) in BAP N-50 (Fig. 3B). For GA treatments, all biomass productivities were higher than non-stimulated ones. GA N-0 had the major difference in biomass productivity, which was 32.00 mg L-1 d-1 (DW), 1.48-fold more than the control (Fig. 4B).
Figure 3: Algal growth rates (A), biomass productivity (mg L-1 d-1) (B), and lipid percentage (DW) (C) of S. obliquus. Each chart shows the 10-5 M BAP stimulus in S. obliquus at different N percentages: 0% (BAP N-0), 25% (BAP N-25), 50% (BAP N-50), 100% (BAP N-100), and without 10-5 M BAP stimulus at different N-percentages: 0% (N-0), 25% (N-25), 50% (N-50), 100% (N-100). In growth kinetics chart, *, **, °, and °°, indicate the significant differences in each pair from the third to the fifteenth day. The error bars indicate the standard deviation of the mean. Tukey’s multiple comparison test was used when the ANOVA detected significant differences (p < 0.05) between each condition. NS= non-significant data
Figure 4: Algal growth rates (A), biomass productivity (mg L-1 d-1) (B), and lipid percentage (DW) (C) of S. obliquus. Each chart shows the 10-6 M GA stimulus in S. obliquus at different N percentages: 0% (GA N-0), 25% (GA N-25), 50% (GA N-50), 100% (GA N-100), and without 10-6 M GA stimulus at different N-percentages: 0% (N-0), 25% (N-25), 50% (N-50), 100% (N-100). In growth kinetics chart, *, **, °, and °°, indicate the significant differences in each pair from the third to the fifteenth day. The error bars indicate the standard deviation of the mean. Tukey’s multiple comparison test was used when the ANOVA detected significant differences (p < 0.05) between each condition. NS= non-significant data
BAP and GA, coupled to N-limitation, also altered lipid production. BAP N-0 produced the highest lipid yield with 55% (DW) and for GA N-0, it was 50%. At day three, BAP N-0 culture had a significant increase in lipid production from 1.4-1.8-fold compared to the other BAP N-limited experiments, and on day seven, a 6.0-fold increase (53.9% lipid yield DW) compared to N-100 (Fig. 3C).
GA N-100 did not show a significant lipid production difference compared to N-100 without stimulus (Fig. 4C). At the experimental endpoint (day 15), the controls (N-100 without phytohormones) produced a 16% maximum lipid in S. obliquus DW. BAP N-0 and GA N-0 exceeded these results by 3.4 and 3.1-fold, respectively (Figs. 3C and 4C).
3.3 Effect of BAP and GA on the Fatty Acid Profile of S. obliquus under N-limited Conditions
The methylated fatty acids profiles induced by 10-5 M BAP and 10-6 M GA under N-limited conditions are shown in Tab. 1. BAP N-0 had the most significant increase in C18:1 (41.12%), and BAP N-100, the highest palmitic acid C16:0 (22.58%) accumulation. The saturated fatty acid (SFA) composition decreased to 21.41% with BAP N-0 treatment. In GA N-0 experiments, C18:1 was the predominant fatty acid with 39.21%, and at GA N-100, the highest increase was for palmitic acid (C16:0) with 37.31%. The BAP stimulus and higher N deficiency stress degree increased MUFAs and PUFAs percentages. The GA stimulus, along with increased N limitation in S. obliquus, led to a decrease in SFAs compared to the GA N-100 control. Also, MUFAs values increased in a manner dependent on N limitation. Treatment with GA N-0 produced the maximum accumulation of MUFAs with 43.07%, 1.68-fold more than the control. In GA N-100, the SFAs composition was the highest at 53.70%, while it was 29.35% in control N-100 without stimulus.
3.4 Effect of BAP and GA in S. obliquus under N-Limited Conditions on the Expression of ACP, FATA, SAD, and DGAT
All N-limited treatments, with and without 10-5 M BAP, induced an over-expression in the four genes analyzed (Figs. 5A–5D). Over-expression was N-concentration dependent, with significant differences (p ≤ 0.05) in ACP, DGAT, and FATA genes. The BAP N-0 treatment showed a higher expression of ACP with a 1.26-fold increase compared with the N-0 control, and a 3.5-fold increment compared to the non-stimulated N-100 control (Fig. 5A). FATA expression was the most pronounced with a 4.0-fold increase in the BAP N-0 and N-0 BAP-less treatments. FATA expression levels were 2.0-fold higher in BAP N-100 compared to the N-100 experiment (Fig. 5B). In SAD, the BAP N-limited treatments induced a gene expression increment of up 2.3-fold compared to N-100 control (Fig. 5C). DGAT expression in BAP N-limited cultures was not significantly different from the N-limited controls; nonetheless, N-100 BAP increased 1.7-fold and N-0 BAP 2.2-fold DGAT expression with respect to the N-replete control (Fig. 5D).
Figure 5: Relative expression of (A) ACP, (B) FATA, (C) SAD, and (D) DGAT in S. obliquus. The relative expression levels of S. obliquus genes are represented; the black bars represent the expression at different N-percentages: 0%, 25%, 50%, and 100%, without BAP supplementation and the grey bars represent the gene expression at the same N-percentages: 0%, 25%, 50%, and 100%, with 10-5 M BAP supplementation. The analysis by qRT-PCR was normalized with the constitutive gene 18S-ribosomal. The error bars represent one standard deviation. Data with * are significantly different, by the Tukey’s test p < 0.05
The ACP, DGAT, and FATA expressions in the GA N-100 cultures increased by 3.3, 2.0, and 2.1-folds, respectively, compared to N-100 treatment. The ACP and DGAT over-expression in N-limited (N-0, N-25, and N-50) treatments were slightly higher than the GA N-limited experiments (Figs. 6A and 6B). SAD expression levels in GA-induced cultures were not significantly higher (p ≤ 0.05) than N-limited tests without stimulus. Although, there was a 2.0-fold SAD expression increase in the total N-absence compared to the N-100 trial (Fig. 6C). FATA expression in N-limited and unstimulated GA treatments were higher than those with GA-added, increased expression by 4.1-fold with N-0, 2.9 with N-25, and 2.65-fold with N-50, compared with N-100 (Fig. 6D).
Figure 6: Relative expression of (A) ACP, (B) FATA, (C) SAD, and (D) DGAT in S. obliquus. The relative expression levels of S. obliquus genes are represented; the black bars represent the expression at different N-percentages: 0%, 25%, 50%, and 100%, without GA supplementation and the grey bars represent the gene expression at the same N-percentages: 0%, 25%, 50%, and 100%, with 10-6 M GA supplementation. The analysis by qRT-PCR was normalized with the constitutive gene 18S-ribosomal. The error bars represent one standard deviation. Data with * are significantly different, by the Tukey’s test p < 0.05
Recently, phytohormone supplementation has been used to improve microalgae growth, division, and metabolite production [20]. However, the biochemical effects of phytohormones on various microalgae species are not entirely established. There is little knowledge about the functional role of BAP and GA in S. obliquus physiology under N-limited stress conditions. Therefore, our research aims to determine the usefulness of these chemical regulators for their potential use in the biotechnology field.
4.1 Cell Density, Biomass Productivity and Lipids Induced by BAP and GA
The physiological effects of cytokinins and gibberellins in microalgae are similar to those exerted on plants. When these hormones were applied exogenously to some microalgae strains, cell growth, biomass, and photosynthetic pigments increased [21].
S. obliquus lipid yield increased significantly on the fifth day of the experiment by 2.85-fold (19.70% DW) (Fig. 1B). Similarly, Czerpak et al. [22], observed a biomass increase and in the α- and β-carotene production in Chlorella pyreinosa as BAP concentration increased. In C. pyreinosa, BAP increased biomass, and the production of α-linoleic acid had an increment by 3.03-fold [23]. S. obliquus contains 12%–14% of lipids DW [24]; compared to these results, 10-5 M BAP application increased lipid yield to 27% DW. According to Yu et al. [25], BAP could change the carbon metabolic flow towards fatty acid synthesis. On the other hand, 10-6 M GA generated the highest biomass productivity with 59.88 mg L-1 d-1, while the cell number was 1.35-fold more elevated than the control (Figs. 2A and 2C). GA has several applications in the agro-industrial sector because of its multifunctional activity as it induces plant growth, stimulates seed germination, intervenes in cell elongation, and responds to abiotic stress [26]. Du et al. [27] observed an increase in biomass concentration in C. pyreinosa cultures stimulated with 10 and 20 mg L-1 GA. In another report, the exogenous addition of 10-7–10-6 M GA increased cell number and photosynthetic pigments under stress conditions by heavy metals such as cadmium and lead [28]. Unlike BAP, GA did not show a significant increase in lipid synthesis (Fig. 2B). This behavior was described in Chlamydomona reinhardtii [29] and Chlorella vulgaris [30].
4.2 Cell Density, Biomass Productivity and Total Lipids Induced with BAP and GA under N-Limited Conditions
N limitation is the most widely used strategy to induce higher accumulation of neutral lipids in microalgae, since it stimulates the hydrolysis of phospholipids, increases the intracellular fatty acid fraction of acyl-Coenzyme-A (acyl-CoA) and activates diacylglycerol acyltransferase (DGAT), which in turn catalyzes the conversion of acyl-CoA to triacylglycerol (TAG) [31]. However, it simultaneously induces rapid protein biodegradation and a dramatic decrease in cell growth and biomass productivity [32]. The phytohormone application in N-limited microalgae cultures has resulted in high lipid and biomass productivity in Acutodesmus obliquus [33] and Graesiella emersonii NC-M1, and Chlorophyta sp. NC-M5 [34]. To our knowledge, this is the first report where BAP and GA are used with nitrogen limitation to stimulate lipid production and prevent a drastic drop in cell density. We found that N limitation in S. obliquus supplemented with 10-5 M BAP significantly improved the biomass productivity 1.65-1.92-fold compared with the N-limited trials without BAP. It also significantly increased lipid yield (up to 1.8-fold) compared to N-reduced assays without BAP (Figs. 3A–3C). There is evidence that cytokinins confer increased resistance to abiotic stress in plants, promote photosynthesis, stimulate protein synthesis in chloroplasts, and regulate carbon and nitrogen metabolism [35]. The increase in lipids content in the N absence/limitation is attributed to a redistribution of carbon from carbohydrate biosynthesis to lipid synthesis [36], and an increase in the activity of ATP synthetase, calmodulin, and cyclic electron flow in photosystem I [37].
On the other hand, when 10-6 M GA was added to the N-limited S. obliquus cultures, cell density was significantly higher from 1.14 to 2.17-fold than the untreated cultures (Fig. 4A). The effect of GA on lipid production was not as pronounced as in the case of BAP, probably because of the carbon flow directed towards carbohydrate synthesis to maintain cell growth in the face of N deficiency [38]. However, the N limitation stress induced a significant lipid increase compared to the N-replete controls (Fig. 4B). There are few known about the role of the phytohormones in microalgae metabolism under abiotic stress; however, an expected mechanism for stress regulation and lipid biosynthesis involves cellular responses such as over-expression of ribulose 1,5 bisphosphate carboxylase/oxygenase gene (RBCL), improved photosynthetic and chlorophyll efficiency, and increased production of antioxidases and antioxidants [39].
4.3 S. obliquus Fatty Acid Profile Stimulated with BAP and GA under N-Limited Conditions
The S. obliquus fatty acids profile was modified under the BAP N-limited conditions (Tab. 1). N-0 BAP had a considerable impact on the relative abundance of MUFAs, particularly C18:1, with a 19% increase, a 7.97% decrease in SFA, and 12.85% decrease in PUFAs, compared to the N-100 treatment without BAP stimulus. These results are relevant because high oleic acid percentages and lower amounts of PUFAs increase the oxidative biodiesel stability and significantly reduce the nitrogen oxides (NOx) generation [40]. In agreement with our results, S. obliquus also had an increase in C18:1 by subjecting to N-depleted conditions [41]. BAP supplementation in Scenedesmus sp. LX1 caused an increase in MUFAs, and as the BAP dose increased, PUFAs decreased [42]. For the GA effect in S. obliquus, we found that the most abundant fatty acids were C16:0, C18:0, and C18:1. Similar results were obtained by Jusoh et al. [30] in C. vulgaris.
The relative abundance of SFAs in S. obliquus N-limited, decreased with increasing nutritional stress, while the MUFAs composition became more abundant with increasing N deficiency. These data are consistent with those of Zhao et al. [36] in Monoraphidium sp. and Renuka et al. [33] in A. obliquus. An oil with a high proportion of MUFAs and SFAs is critical for improving biodiesel quality since properties such as viscosity, cetane number, and iodine value are optimized [43]. The results suggest that it is possible to use BAP or GA to induce S. obliquus oil enrichment selectively with SFAs or MUFAs.
N limitation stress in microalgae or exogenous phytohormone application increase the gene-expression in the fatty acid biosynthetic pathway [44,45]. However, few studies have evaluated the expression of genes involved in lipid synthesis under phytohormone stimulus and N limitation.
In this study, three genes from the fatty acid biosynthesis in microalgae were selected: the ACP, SAD and FATA, and DGAT, which are involved in TAG synthesis. Acyl-carrying protein (ACP) is essential for the de novo synthesis of fatty acids, it is responsible for binding and transporting the acyl chains to enzymes involved in the condensation, reduction and dehydration cycles in fatty acid (FA) biosynthesis [46]. ACP over-expression was obtained in all BAP and GA treatments N-limited. ACP expression level increased 3.5-fold with BAP N-0 and 3.8-fold with GA N-50, both compared to their N-100 controls without stimulus (Figs. 5A and 6A). Similarly, Sharma et al. [12], showed in Scenedesmus dimorphus and S. quadricauda that ACP expression increases by 3.0 and 9.0-fold, respectively, under N-limited (2.5 mM) conditions. In contrast, BAP did not affect ACP regulation in Botryococcus braunii; however, with GA (15 mg L-1), ACP was over-expressed by up to 6.5-fold [47].
Of all the genes analyzed, FATA expression was the most pronounced (increased up to 4.0-fold). FATA primary function is to produce free fatty acids by hydrolyzing oleoyl-ACP substrates, mainly C18:1-ACP [48]. The over-expression of some thioesterase genes under N deficiency conditions increased fatty acid production by regulating the feedback rate of fatty acid biosynthesis [49]. In this study, we found that the higher the N deficiency, the higher the FATA over-expression. BAP and GA induced FATA over-expression; however, N limitation was the main factor that triggered gene-expression (Fig. 5B). This behavior is related to S. dimorphus and S. quadricauda results, where FATA was over-expressed under N-limited conditions by 1.5 and 7.0-folds, respectively [12].
The FATA expression with GA N-100 was 2.0- fold higher than its N-100 control (Fig. 6B). It was reported that GA stimulates esterase activity in C. pyrenoidosa [50]. The results are consistent with those of Sivaramakrishnan and Incharoensakdi [51], where FATA expression increased by 1.8-fold in Chorella sp. In the case of BAP N-100 treatment, it also produced a 2.0-fold increase in FATA expression. Similarly, in C. pyrenoidosa, BAP increased by 2.0-fold FATA expression, while in B. braunii, the addition of BAP increased FATA expression by 63.50-fold [27].
The oleic chains synthesis are primarily due to the action of SAD. After stearoyl-ACP (C18:0-ACP) is formed, SAD converts it to C18:1-ACP by introducing a double bond at the ∆9 position, then being transported out of the chloroplast [52]. The N limitation coupled to BAP or GA stimulus resulted in over-expression of SAD by 1.33-2.35-fold (Figs. 5C and 6C). Similarly, in S. dimorphus SD12 and SD16, the N-deficit stress increased the SAD expression by 1.0 and 1.5, respectively [12]. Although SAD expression increases were not as pronounced, S. obliquus oil had a considerable enrichment in C18:1 (Tab. 1). The same behavior was obtained in B. braunii [53]. The BAP and GA effect on SAD overexpression was similar to that of N-limited and non-stimulated controls. Like our results, GA also did not increase SAD expression in C. pyreinosa [27]. The SAD expression had a significant increase with BAP N-0 treatment (Fig. 5C). This result suggests that oleic acid accumulation can be induced since SAD has shown a positive correlation with C18:1 [11]. DGTA exhibited an over-expression in BAP N-100 and GA N-100 trials, compared to the non-supplemented N-100 controls (Figs. 5D and 6D). BAP N-0 increased the DGAT expression by 2.0-fold, although, there were no significant differences with its N-0 control (Fig. 6D). DGTA has been positively correlated with lipid accumulation since it is the enzyme that catalyzes the last step for TAG biosynthesis [54]. In this work, as the N limitation incremented, the fatty acids C16:0 and C18:1 increased (Tab. 1). It has been seen that DGAT favors the addition of the C16:0 or C18:1 chains to the sn-3 position of diacylglycerols (DAG) [55]. In comparison with our results, other microalgae species have shown a similar increase in N-deficit induced DGAT expression, for example, in S. dimorphus SD12, and S. quadricauda SQ19, DGAT increased 1.5, and 2.0-fold, respectively [12]. In Graesiella emersonii, DGAT expression increased 3.17-fold when a mixture of IAA and kinetin were applied under N-limited conditions [34].
Currently, the mechanism of action by which phytohormones alter or regulate the expression levels of genes involved in microalgae lipid metabolism is not entirely elucidated. However, BAP and GA can control the metabolic flow towards fatty acid synthesis, promote glycolysis, interferes in the mevalonate pathway, and direct the carbon flow to pyruvate and acetyl-Co synthesis [25,56].
Optimal concentrations of 10-5 M BAP and 10-6 M GA increased cell growth and biomass productivity in S. obliquus. GA did not affect the total lipid production, and BAP increased the lipids to 27%. Exogenous 10-5 M BAP and 10-6 M GA supplementation along with nitrogen-starvation produced a lipid yield of 55% and 50% yield, respectively, and maintained the microalgal growth. The proportions of fatty acid compositions were also affected, leading to high values of C16:0 and C18:1, which are indispensable for biodiesel’s good quality. The ACP, SAD, FATA, and DGAT expressions were up-regulated mainly by the increment of the N-limited conditions.
This study generated relevant information to increase cell growth, biomass, and lipid yield in S. obliquus, which has excellent potential as a raw material for biodiesel production due to the quality of its oil and the capacity to accumulate high quantities of oil. It also provides data on the regulation of some key genes in lipid synthesis, which can be considered in metabolic engineering strategies to optimize lipid productivity in microalgae for sustainable biofuels.
Acknowledgement: We thank Dr. Roberto Rico for reviewing the English in the manuscript. JFMD and HCCA gratefully acknowledge to Universidad Autónoma de Aguascalientes (UAA) for support. HCCA specially thanks to Comité Técnico para el Otorgamiento de Becas, Estudio, Apoyos Económicos y Licencias con Goce de Sueldo of Instituto Politécnico Nacional (COTEBAL-IPN) for financial support.
Funding Statement: The authors received no specific funding for this study.
Conflicts of Interest: The authors declare that they have no conflicts of interest to report regarding the present study.
References
1. Jha, D., Jain, V., Sharma, B., Kant, A., Garlapati, V. K. (2017). Microalgae-based pharmaceuticals and nutraceuticals: An emerging field with immense market potential. ChemBioEng Reviews, 4(4), 257–272. DOI 10.1002/cben.201600023. [Google Scholar] [CrossRef]
2. Faried, M., Samer, M., Abdelsalam, E., Yousef, R. S., Attia, Y. A. et al. (2017). Biodiesel production from microalgae: Processes, technologies and recent advancements. Renewable and Sustainable Energy Reviews, 79, 893–913. DOI 10.1016/j.rser.2017.05.199. [Google Scholar] [CrossRef]
3. Patnaik, R., Mallick, N. (2015). Utilization of Scenedesmus obliquus biomass as feedstock for biodiesel and other industrially important co-products: An integrated paradigm for microalgal biorefinery. Algal Research, 12, 328–336. DOI 10.1016/j.algal.2015.09.009. [Google Scholar] [CrossRef]
4. Fakhry, E. M., El Maghraby, D. M. (2015). Lipid accumulation in response to nitrogen limitation and variation of temperature in Nannochloropsis salina. Botanical Studies, 56(1), 111. DOI 10.1186/s40529-015-0085-7. [Google Scholar] [PubMed] [CrossRef]
5. Dickinson, S., Mientus, M., Frey, D., Amini-Hajibashi, A., Ozturk, S. et al. (2017). A review of biodiesel production from microalgae. Clean Technologies and Environmental Policy, 19(3), 637–668. DOI 10.1007/s10098-016-1309-6. [Google Scholar] [CrossRef]
6. Han, X., Zeng, H., Bartocci, P., Fantozzi, F., Yan, Y. (2018). Phytohormones and effects on growth and metabolites of microalgae: A review. Fermentation, 4(2), 25. DOI 10.3390/fermentation4020025. [Google Scholar] [CrossRef]
7. Lu, Y., Xu, J. (2015). Phytohormones in microalgae: A new opportunity for microalgal biotechnology? Trends in Plant Science, 20(5), 273–282. DOI 10.1016/j.tplants.2015.01.006. [Google Scholar] [PubMed] [CrossRef]
8. Sulochana, S. B., Arumugam, M. (2016). Influence of abscisic acid on growth, biomass and lipid yield of Scenedesmus quadricauda under nitrogen starved condition. Bioresource Technology, 213, 198–203. DOI 10.1016/j.biortech.2016.02.078. [Google Scholar] [PubMed] [CrossRef]
9. Chokshi, K., Pancha, I., Ghosh, A., Mishra, S. (2017). Nitrogen starvation-induced cellular crosstalk of ROS-scavenging antioxidants and phytohormone enhanced the biofuel potential of green microalga Acutodesmus dimorphus. Biotechnology for Biofuels, 10(1), 294. DOI 10.1186/s13068-017-0747-7. [Google Scholar] [PubMed] [CrossRef]
10. Rismani-Yazdi, H., Haznedaroglu, B. Z., Hsin, C., Peccia, J. (2012). Transcriptomic analysis of the oleaginous microalga Neochloris oleoabundans reveals metabolic insights into triacylglyceride accumulation. Biotechnology for Biofuels, 5(1), 74. DOI 10.1186/1754-6834-5-74. [Google Scholar] [PubMed] [CrossRef]
11. Lei, A., Chen, H., Shen, G., Hu, Z., Chen, L. et al. (2012). Expression of fatty acid synthesis genes and fatty acid accumulation in Haematococcus pluvialis under different stressors. Biotechnology for Biofuels, 5(1), 18. DOI 10.1186/1754-6834-5-18. [Google Scholar] [PubMed] [CrossRef]
12. Sharma, T., Gour, R. S., Kant, A., Chauhan, R. S. (2015). Lipid content in Scenedesmus species correlates with multiple genes of fatty acid and triacylglycerol biosynthetic pathways. Algal Research, 12, 341–349. DOI 10.1016/j.algal.2015.09.006. [Google Scholar] [CrossRef]
13. Andersen, R. A. (2005). Algal culturing techniques. London: Elsevier Academic Press. [Google Scholar]
14. George, B., Pancha, I., Desai, C., Chokshi, K., Paliwal, C. et al. (2014). Effects of different media composition, light intensity and photoperiod on morphology and physiology of freshwater microalgae Ankistrodesmus falcatus–A potential strain for bio-fuel production. Bioresource Technology, 171, 367–374. DOI 10.1016/j.biortech.2014.08.086. [Google Scholar] [PubMed] [CrossRef]
15. Babu, A. G., Wu, X., Kabra, A. N., Kim, D. P. (2017). Cultivation of an indigenous Chlorella sorokiniana with phytohormones for biomass and lipid production under N-limitation. Algal Research, 23, 178–185. DOI 10.1016/j.algal.2017.02.004. [Google Scholar] [CrossRef]
16. Park, J., Jeong, H. J., Yoon, E. Y., Moon, S. J. (2016). Easy and rapid quantification of lipid contents of marine dinoflagellates using the sulpho-phospho-vanillin method. ALGAE, 31(4), 391–401. DOI 10.4490/algae.2016.31.12.7. [Google Scholar] [CrossRef]
17. Bligh, E. G., Dyer, W. J. (1959). A rapid method of total lipid extraction and purification. Canadian Journal of Biochemistry and Physiology, 37(8), 911–917. DOI 10.1139/o59-099. [Google Scholar] [PubMed] [CrossRef]
18. Salas-Montantes, C. J., González-Ortega, O., Ochoa-Alfaro, A. E., Camarena-Rangel, R., Paz-Maldonado, L. M. T. et al. (2018). Lipid accumulation during nitrogen and sulfur starvation in Chlamydomonas reinhardtii overexpressing a transcription factor. Journal of Applied Phycology, 30(3), 1721–1733. DOI 10.1007/s10811-018-1393-6. [Google Scholar] [CrossRef]
19. Livak, K. J., Schmittgen, T. D. (2001). Analysis of relative gene expression data using real-time quantitative PCR and the 2-ΔΔCT method. Methods, 25(4), 402–408. DOI 10.1006/meth.2001.1262. [Google Scholar] [PubMed] [CrossRef]
20. Yu, X., Chen, L., Zhang, W. (2015). Chemicals to enhance microalgal growth and accumulation of high-value bioproducts. Frontiers in Microbiology, 6, 56. [Google Scholar] [PubMed]
21. Romanenko, K. O., Kosakovskaya, I. V., Romanenko, P. O. (2016). Phytohormones of microalgae: Biological role and involvement in the regulation of physiological processes. International Journal on Algae, 18(2), 179–201. DOI 10.1615/InterJAlgae.v18.i2.70. [Google Scholar] [CrossRef]
22. Czerpak, R., Bajguz, A. (2014). Stimulatory effect of auxins and cytokinins on carotenes, with differential effects on xanthophylls in the green alga Chlorella pyrenoidosa Chick. Acta Societatis Botanicorum Poloniae, 66(1), 41–46. DOI 10.5586/asbp.1997.006. [Google Scholar] [CrossRef]
23. Kokkiligadda, S., Pandey, B., Ronda, S. R. (2017). Effect of plant growth regulators on production of alpha-linolenic acid from microalgae Chlorella pyrenoidosa. Sādhanā, 42(10), 1821–1824. [Google Scholar]
24. Wu, X., Ruan, R., Du, Z., Liu, Y. (2012). Current status and prospects of biodiesel production from microalgae. Energies, 5(8), 2667–2682. DOI 10.3390/en5082667. [Google Scholar] [CrossRef]
25. Yu, X. J., Sun, J., Zheng, J. Y., Sun, Y. Q., Wang, Z. (2016). Metabolomics analysis reveals 6-benzylaminopurine as a stimulator for improving lipid and DHA accumulation of Aurantiochytrium sp. Journal of Chemical Technology & Biotechnology, 91(4), 1199–1207. DOI 10.1002/jctb.4869. [Google Scholar] [PubMed] [CrossRef]
26. Camara, M. C., Vandenberghe, L. P., Rodrigues, C., de Oliveira, J., Faulds, C. et al. (2018). Current advances in gibberellic acid (GA3) production, patented technologies and potential applications. Planta, 248(5), 1049–1062. DOI 10.1007/s00425-018-2959-x. [Google Scholar] [PubMed] [CrossRef]
27. Du, H., Ahmed, F., Lin, B., Li, Z., Huang, Y. et al. (2017). The effects of plant growth regulators on cell growth, protein, carotenoid, PUFAs and lipid production of Chlorella pyrenoidosa ZF strain. Energies, 10(11), 1696. DOI 10.3390/en10111696. [Google Scholar] [CrossRef]
28. Falkowska, M., Pietryczuk, A., Piotrowska, A., Bajguz, A., Grygoruk, A. et al. (2011). The effect of gibberellic acid (GA3) on growth, metal biosorption and metabolism of the green algae Chlorella vulgaris (Chlorophyceae) Beijerinck exposed to cadmium and lead stress. Polish Journal of Environmental Studies, 20(1), 53–59. [Google Scholar]
29. Park, W. K., Yoo, G., Moon, M., Kim, C. W., Choi, Y. E. et al. (2013). Phytohormone supplementation significantly increases growth of Chlamydomonas reinhardtii cultivated for biodiesel production. Applied Biochemistry and Biotechnology, 171(5), 1128–1142. DOI 10.1007/s12010-013-0386-9. [Google Scholar] [PubMed] [CrossRef]
30. Jusoh, M., Loh, S. H., Aziz, A., San Cha, T. (2019). Gibberellin promotes cell growth and induces changes in fatty acid biosynthesis and upregulates fatty acid biosynthetic genes in Chlorella vulgaris UMT-M1. Applied Biochemistry and Biotechnology, 188(2), 450–459. DOI 10.1007/s12010-018-02937-4. [Google Scholar] [PubMed] [CrossRef]
31. Xin, L., Hong-Ying, H., Ke, G., Ying-Xue, S. (2010). Effects of different nitrogen and phosphorus concentrations on the growth, nutrient uptake, and lipid accumulation of a freshwater microalga Scenedesmus sp. Bioresource Technology, 101(14), 5494–5500. DOI 10.1016/j.biortech.2010.02.016. [Google Scholar] [PubMed] [CrossRef]
32. Juneja, A., Ceballos, R. M., Murthy, G. S. (2013). Effects of environmental factors and nutrient availability on the biochemical composition of algae for biofuels production: A review. Energies, 6(9), 4607–4638. DOI 10.3390/en6094607. [Google Scholar] [CrossRef]
33. Renuka, N., Guldhe, A., Singh, P., Bux, F. (2018). Combined effect of exogenous phytohormones on biomass and lipid production in Acutodesmus obliquus under nitrogen limitation. Energy Conversion and Management, 168, 522–528. DOI 10.1016/j.enconman.2018.05.029. [Google Scholar] [CrossRef]
34. Mandal, M. K., Chanu, N. K., Chaurasia, N. (2020). Exogenous addition of indole acetic acid and kinetin under nitrogen-limited medium enhances lipid yield and expression of glycerol-3-phosphate acyltransferase and diacylglycerol acyltransferase genes in indigenous microalgae: A potential approach for biodiesel production. Bioresource Technology, 297, 122439. DOI 10.1016/j.biortech.2019.122439. [Google Scholar] [PubMed] [CrossRef]
35. Wu, X., He, J., Chen, J., Yang, S., Zha, D. (2014). Alleviation of exogenous 6-benzyladenine on two genotypes of eggplant (Solanum melongena Mill.) growth under salt stress. Protoplasma, 251(1), 169–176. DOI 10.1007/s00709-013-0535-6. [Google Scholar] [PubMed] [CrossRef]
36. Zhao, Y., Li, D., Xu, J. W., Zhao, P., Li, T. et al. (2018). Melatonin enhances lipid production in Monoraphidium sp. QLY-1 under nitrogen deficiency conditions via a multi-level mechanism. Bioresource Technology, 259, 46–53. DOI 10.1016/j.biortech.2018.03.014. [Google Scholar] [PubMed] [CrossRef]
37. Chen, H., Hu, J., Qiao, Y., Chen, W., Rong, J. et al. (2015). Ca2+-regulated cyclic electron flow supplies ATP for nitrogen starvation-induced lipid biosynthesis in green alga. Scientific Reports, 5(1), 294. DOI 10.1038/srep15117. [Google Scholar] [PubMed] [CrossRef]
38. Ikaran, Z., Suárez-Alvarez, S., Urreta, I., Castañón, S. (2015). The effect of nitrogen limitation on the physiology and metabolism of Chlorella vulgaris var L3. Algal Research, 10, 134–144. DOI 10.1016/j.algal.2015.04.023. [Google Scholar] [CrossRef]
39. Zhao, Y., Wang, H. P., Han, B., Yu, X. (2019). Coupling of abiotic stresses and phytohormones for the production of lipids and high-value by-products by microalgae: A review. Bioresource Technology, 274, 549–556. DOI 10.1016/j.biortech.2018.12.030. [Google Scholar] [PubMed] [CrossRef]
40. Tat, M. E., Wang, P. S., Van Gerpen, J. H., Clemente, T. E. (2007). Exhaust emissions from an engine fueled with biodiesel from high-oleic soybeans. Journal of the American Oil Chemists’ Society, 84(9), 865–869. DOI 10.1007/s11746-007-1109-6. [Google Scholar] [CrossRef]
41. Breuer, G., Lamers, P. P., Martens, D. E., Draaisma, R. B., Wijffels, R. H. (2013). Effect of light intensity, pH, and temperature on triacylglycerol (TAG) accumulation induced by nitrogen starvation in Scenedesmus obliquus. Bioresource Technology, 143, 1–9. DOI 10.1016/j.biortech.2013.05.105. [Google Scholar] [PubMed] [CrossRef]
42. Dao, G. H., Wang, X. X., Zhang, T. Y., Wu, G. X., Zhan, X. M. et al. (2019). Enhanced biomass production and fatty acid accumulation in Scenedesmus sp. LX1 treated with 6-benzylaminopurine. Algal Research, 44, 101714. DOI 10.1016/j.algal.2019.101714. [Google Scholar] [CrossRef]
43. Hoekman, S. K., Broch, A., Robbins, C., Ceniceros, E., Natarajan, M. (2012). Review of biodiesel composition, properties, and specifications. Renewable and Sustainable Energy Reviews, 16(1), 143–169. DOI 10.1016/j.rser.2011.07.143. [Google Scholar] [CrossRef]
44. Banerjee, A., Maiti, S. K., Guria, C., Banerjee, C. (2017). Metabolic pathways for lipid synthesis under nitrogen stress in Chlamydomonas and Nannochloropsis. Biotechnology Letters, 39(1), 1–11. DOI 10.1007/s10529-016-2216-y. [Google Scholar] [PubMed] [CrossRef]
45. Norlina, R., Norashikin, M. N., Loh, S. H., Aziz, A., San Cha, T. (2020). Exogenous abscisic acid supplementation at early stationary growth phase triggers changes in the regulation of fatty acid biosynthesis in Chlorella vulgaris UMT-M1. Applied Biochemistry and Biotechnology, 191(4), 1653–1669. DOI 10.1007/s12010-020-03312-y. [Google Scholar] [PubMed] [CrossRef]
46. Chan, D. I., Vogel, H. J. (2010). Current understanding of fatty acid biosynthesis and the acyl carrier protein. Biochemical Journal, 430(1), 1–19. DOI 10.1042/BJ20100462. [Google Scholar] [PubMed] [CrossRef]
47. Du, H., Ren, J., Li, Z., Zhang, H., Wang, K. et al. (2020). Plant Growth Regulators Affect Biomass, Protein, Carotenoid, and Lipid Production in Botryococcus Braunii. Aquaculture International, 28, 1319–1340. [Google Scholar]
48. Salas, J. J., Ohlrogge, J. B. (2002). Characterization of substrate specificity of plant FatA and FatB acyl-ACP thioesterases. Archives of Biochemistry and Biophysics, 403(1), 25–34. DOI 10.1016/S0003-9861(02)00017-6. [Google Scholar] [PubMed] [CrossRef]
49. Lu, X., Vora, H., Khosla, C. (2008). Overproduction of free fatty acids in E. coli: Implications for biodiesel production. Metabolic Engineering, 10(6), 333–339. DOI 10.1016/j.ymben.2008.08.006. [Google Scholar] [PubMed] [CrossRef]
50. Du, K., Tao, H., Wen, X., Geng, Y., Li, Y. (2015). Enhanced growth and lipid production of Chlorella pyrenoidosa by plant growth regulator GA3. Fresenius Environment Bulletin, 24, 3414–3419. [Google Scholar]
51. Sivaramakrishnan, R., Incharoensakdi, A. (2020). Plant hormone induced enrichment of Chlorella sp. omega-3 fatty acids. Biotechnology for Biofuels, 13(1), 1902. DOI 10.1186/s13068-019-1647-9. [Google Scholar] [PubMed] [CrossRef]
52. Zhao, N., Zhang, Y., Li, Q., Li, R., Xia, X. et al. (2015). Identification and expression of a stearoyl-ACP desaturase gene responsible for oleic acid accumulation in Xanthoceras sorbifolia seeds. Plant Physiology and Biochemistry, 87, 9–16. DOI 10.1016/j.plaphy.2014.12.009. [Google Scholar] [PubMed] [CrossRef]
53. Choi, G. G., Kim, B. H., Ahn, C. Y., Oh, H. M. (2011). Effect of nitrogen limitation on oleic acid biosynthesis in Botryococcus braunii. Journal of Applied Phycology, 23(6), 1031–1037. DOI 10.1007/s10811-010-9636-1. [Google Scholar] [CrossRef]
54. Fan, J., Cui, Y., Wan, M., Wang, W., Li, Y. (2014). Lipid accumulation and biosynthesis genes response of the oleaginous Chlorella pyrenoidosa under three nutrition stressors. Biotechnology for Biofuels, 7(1), 17. DOI 10.1186/1754-6834-7-17. [Google Scholar] [PubMed] [CrossRef]
55. Li, R., Yu, K., Hildebrand, D. F. (2010). DGAT1, DGAT2 and PDAT expression in seeds and other tissues of epoxy and hydroxy fatty acid accumulating plants. Lipids, 45(2), 145–157. DOI 10.1007/s11745-010-3385-4. [Google Scholar] [PubMed] [CrossRef]
56. Yu, X. J., Sun, J., Sun, Y. Q., Zheng, J. Y., Wang, Z. (2016). Metabolomics analysis of phytohormone gibberellin improving lipid and DHA accumulation in Aurantiochytrium sp. Biochemical Engineering Journal, 112, 258–268. DOI 10.1016/j.bej.2016.05.002. [Google Scholar] [CrossRef]
Appendix
Cite This Article
Citations
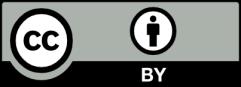
This work is licensed under a Creative Commons Attribution 4.0 International License , which permits unrestricted use, distribution, and reproduction in any medium, provided the original work is properly cited.