Open Access
REVIEW
Metal-based molecules in the treatment of cancer: From bench to bedside
Laboratory of Molecular and Cellular Biology of Cancer, Department of Biomedical Sciences, Faculty of Medicine, Universidad Católica del Norte, Coquimbo, 1781421, Chile
* Corresponding Author: GIULIANO BERNAL. Email:
Oncology Research 2025, 33(4), 759-779. https://doi.org/10.32604/or.2024.057019
Received 06 August 2024; Accepted 15 November 2024; Issue published 19 March 2025
Abstract
Cancer remains one of the leading causes of death in the world, with more than 9 million deaths in 2022, a number that continues to rise. This highlights the urgent need for the development of new drugs, with enhanced antitumor capabilities and fewer side effects. Metal-based drugs have been used in clinical practice since the late 1970s, beginning with the introduction of cisplatin. Later, two additional platinum-based molecules, carboplatin, and oxaliplatin, were introduced, and all three continue to be widely used in the treatment of various cancers. However, despite their significant anticancer activity, the undesirable side effects of these drugs have motivated the scientific community to explore other metal-based complexes with greater anticancer potential and fewer adverse effects. In this context, metals such as ruthenium, copper, gold, zinc, palladium, or iridium, present promising alternatives for the development of new anticancer agents. Unfortunately, although thousands of metal-based drugs have been synthesized and tested both in vitro and in animal models, only a few ruthenium-based drugs have entered clinical trials in recent years. Meanwhile, many other molecules with comparable or even greater anticancer potential have not advanced beyond the laboratory stage. In this review, we will revisit the mechanisms of action and anticancer activities of established platinum-based drugs and explore their use in recent clinical trials. Additionally, we will examine the development of potential new metal-based drugs that could one day contribute to cancer treatment worldwide.Graphic Abstract
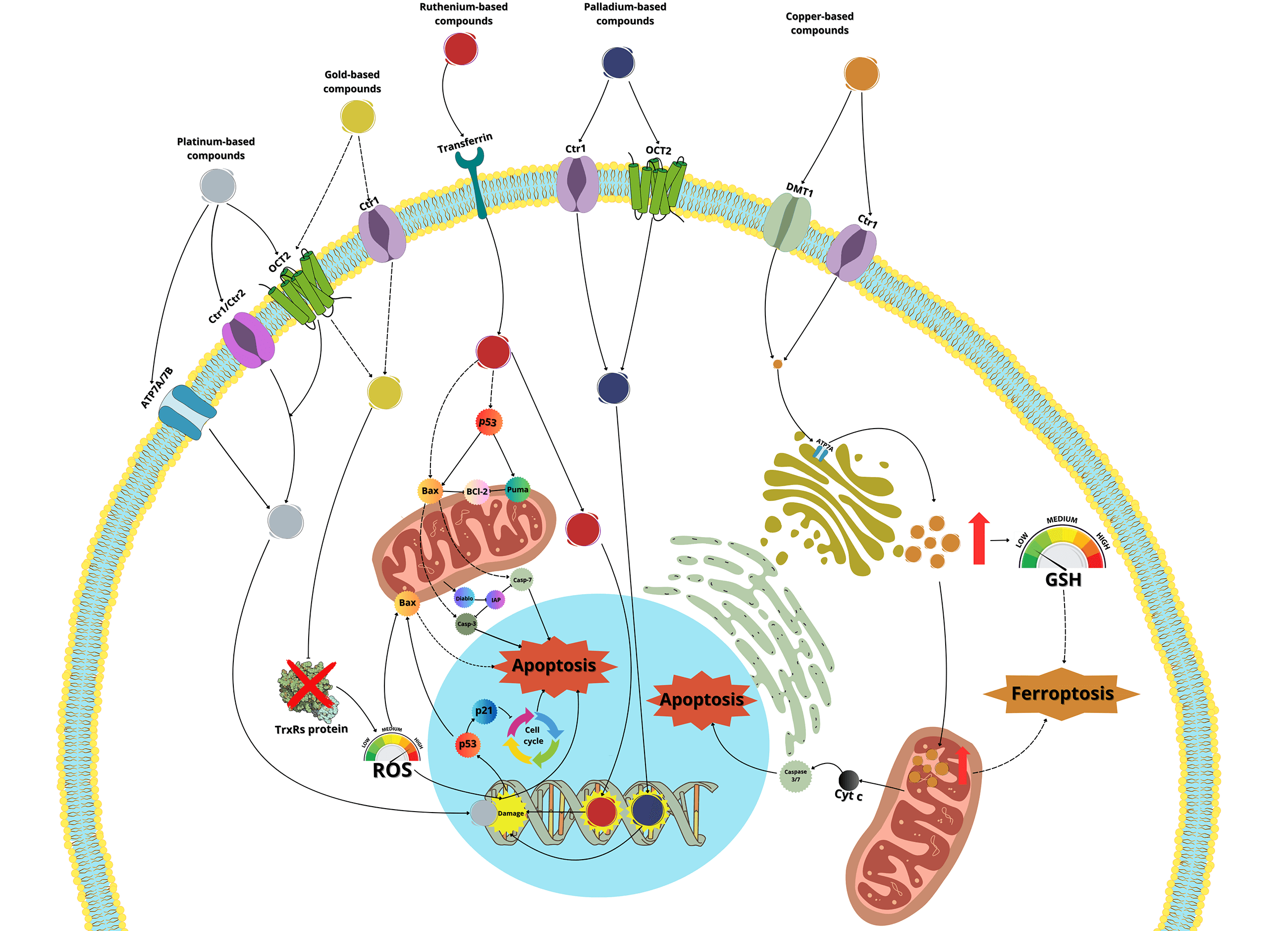
Keywords
Cancer is one of the most significant non-communicable diseases (NCDs) globally, with nearly 20 million new cases and 9.7 million deaths in 2022. By 2050, a projected increase of 77% is expected, leading to over 35 million new cancer cases annually [1]. In light of this alarming trend, there is a critical need for the development of new drugs that can effectively combat the rising incidence of cancer, offering enhanced antitumor capabilities and fewer side effects.
The development of metal-based drugs in cancer therapy dates back to the mid-1960s when Barnett Rosenberg discovered the antiproliferative activity of cisplatin [2,3]. Although the molecule was discovered in 1845 by Michele Peyrone, it was not until 1978 that the Food and Drug Administration (FDA) approved cisplatin for use in cancer therapy [4]. Later, additional platinum drugs, such as carboplatin and oxaliplatin, were introduced [2,4]. These platinum drugs have been extensively used to treat different types of cancer worldwide. However, resistance to these drugs has been observed in many cases, necessitating to combine it with other drugs, such as tyrosine kinase inhibitors (TKIs) (imatinib, nilotinib) [5], taxanes (paclitaxel) [6], ribonucleotide reductase inhibitors (Gemcitabine) [7], phosphatidylinositol 3-kinase (PI3K) inhibitors (Alpelisib) [8], among others. These combinations not only improve the anticancer activity of platinum drugs but also reduce the side effects experienced by patients.
Studies have also demonstrated that synergistic use of platinum drugs with natural compounds, such as oxyresveratrol [9], isovitexin [10], limonene [11], or extracts from fermented ginger [12], Vernonia calvoana [13], Platycodon grandiflorus [14] phenylethanol glycosides from Herba Cistanche [15], or the essential oil of Ylang ylang (Cananga odorata) [16], can enhance efficacy and reduce toxicity.
In a recent article, genes potentially linked to resistance to platin drugs in ovarian cancer were investigated in 90 chemoresistant and 197 chemosensitive tissues. The findings, correlated with data of 1347 patients, revealed that a high expression of PARD6B and STAT5A or a low expression of SOS1, MSH6, and STAT5A could serve as reliable markers for predicting resistance to platinum-based drugs [17].
Since the development of cisplatin, carboplatin, and oxaliplatin, a wide array of new platinum-based drugs has been designed using “structure-activity relationship” models. However, only a few have advanced to clinical trials [2,18]. With the global rise in cancer incidence, other metal-based complexes, incorporating ions such as ruthenium, copper, gold, zinc, palladium, and iridium, have attracted significant interest as potential anticancer agents [18–22].
In this review, we will explore the structures and potential mechanism of action of metal-based drugs, including those containing platinum, ruthenium, copper, gold, palladium, zinc, and iridium, as well as their potential use in cancer treatment.
Biochemical and Clinical Properties of Metal-Based Drugs
Metal-based complexes exhibit remarkable chemical diversity and versatility, which depend on factors such as the coordinated metal, its oxidation state, the number and type of ligands, and specific magnetic and/or optical properties [3]. Metals like platinum, ruthenium, copper, gold, palladium, zinc, or iridium possess notable characteristics, including redox activity, variable coordination modes, and reactivity towards organic substrates, principally proteins or DNA. These properties grant metal complexes significant therapeutic potential in cancer treatment [18].
Cisplatin (Table 1), the first metal-based drug used in the treatment of cancer, exerts its antiproliferative effects primarily by binding to DNA, blocking DNA replication and transcription, and triggering caspase activation and apoptosis [2,4,23]. In the bloodstream, cisplatin is primarily bound to plasma proteins, such as albumin, and is subsequently transported across the plasma membrane by transporters such as copper transporter-1 and -2 (Ctr1, Ctr2), the P-type copper-transporting ATPases ATP7A and ATP7B, and the organic cation transporter-2 (OCT2) [24–27]. Once inside the cell, cisplatin undergoes monoaquation, where one of the chloride ligands is replaced by a water molecule due to the lower chloride concentration in the cytoplasm (4–20 mM) compared to the blood (approximately 100 mM) [2,28]. The monoaquated platinum acts as a potent electrophile, reacting with nitrogen donor atoms in DNA, forming intra- and inter-strand crosslinks that induce DNA adducts and damage [29,30]. This damage activates repair mechanisms, including mismatch repair proteins and high-mobility group box (HMGB) proteins [24,25]. When the damage surpasses the cell’s repair capacity, apoptotic pathways are activated, involving the expression of p53 and Bcl2 Associated X Protein (BAX, part of the Bcl-2 family proteins), the cytochrome c release, and the activation of caspase-3, -7 and -9, leading to apoptosis [2,19,24,30,31].
Cisplatin is used to treat various cancers, including breast, ovarian, testicular, head and neck, esophageal, lung, bladder, and brain cancers [2]. However, cisplatin is associated with severe adverse effects, particularly nephrotoxicity and ototoxicity [32]. Cisplatin-induced nephrotoxicity occurs in approximately 28%–36% of patients due to drug accumulation in proximal tubular cells, which impairs the expression of glucose, amino acid, magnesium, and water transporters, reduces renal blood flow, and induces acute kidney injury (AKI) [33,34]. Nephrotoxicity is more frequent in children than in adults. A recent study involving 159 children treated with cisplatin revealed that 46% developed AKI [35]. Nonetheless, Several natural compounds have demonstrated cytoprotective effects against AKI, including curcumin (a Curcuma derivative) [36]; Gastrodin (the main active ingredient of Gastrodia elata Blume) [37]; ginsenosides (the main active components of ginseng roots) [38]; asiatic acid (triterpenoid extracted from Centella asiatica) [39]; betulinic acid (a triterpene isolated from Silene succulenta Forssk) [40]; Oleanolic acid acetate (a triterpene isolated from Vigna angularis) [41], isorhamnetin (flavanol derived from Hippophae rhamnoides L. and Ginkgo biloba L.) [42]; Kaempferide (flavanol found in the rhizome of Kaempferia galanga, Chromolaena odorata, Alpinia conchigera, and Hippophae rhamnoides L.) [43]; and isoliquiritigenin (flavonoid extracted from Glycyrrhiza glabra, Allium ascalonicum, and Glycine max) [44]. Similar nephroprotective effects have been observed with extracts from Achyranthes aspera root [45]; Olea europaea [46]; Clinacanthus nutans [47]; and Primula vulgaris leaves [48].
In these cases, the protective mechanisms involve a reduction in serum levels of blood urea nitrogen (BUN) and creatinine, along with an increase in glutathione (GSH) levels and activation of Nuclear factor erythroid 2-related factor 2 (Nrf2), a key regulator of cellular redox homeostasis. Furthermore, these compounds reduce serum proinflammatory cytokines, including Tumor Necrosis Factor-alpha (TNF-α), Interleukin-1 beta (IL-1β), and IL-6, while modulating apoptosis by downregulating BAX and upregulating Bcl-2, preventing caspase-3 activation.
Other therapeutics have also demonstrated nephroprotective effects when used concomitantly with cisplatin, including ammonium tetrathiomolybdate [49]; β-hydroxybutyrate [50]; diallyl trisulfide [51]; dihydroartemisinin [52]; omega-3-6-9 [53]; piracetam [54]; sodium thiosulfate [55]; and thrombomodulin [56].
Regarding ototoxicity, cisplatin induces hearing loss in 40%–60% of adults, including 18% who experience severe hearing loss, while in children, more than 70% are affected [57]. A recent study found that the cumulative incidence of cisplatin-induced hearing loss three years after treatment initiation was 75% in children under five years old, compared to 48% in adult patients [58].
Due to these severe side effects, other platinum drugs have been developed and approved for clinical use, including carboplatin and oxaliplatin (Table 1). Thousands of additional platinum-based molecules have been studied globally, some of which have been approved for clinical use in specific countries. These compounds generally act similarly to cisplatin by interacting with DNA [59].
Carboplatin, developed after cisplatin, is used to treat a range of cancers including ovarian, lung, certain head-and-neck cancers, and some metastatic breast cancer. It enters cells via transporters such as Ctr1, ATP7A, and ATP7B, as well as by simple diffusion. Once inside, carboplatin induces the expression of miR-145 and p53, and binds to DNA, causing deformation and initiating apoptosis [60].
A 2019 study comparing the genotoxic effects of cisplatin and carboplatin in cultured human lymphocytes found that both drugs significantly increased chromosomal aberrations, but cisplatin caused a higher frequency of sister-chromatid exchange, likely due to its higher reactivity and faster DNA binding kinetics compared to carboplatin [61]. However, a recent meta-analysis of 20 studies involving 4468 participants demonstrated that carboplatin is superior to cisplatin in treating early triple-negative breast cancer (TNBC), showing better disease-free survival (DFS), overall survival (OS), and pathological complete response (pCR), supporting the use of carboplatin for early TNBC [62]. Another meta-analysis, which included 11,049 patients, demonstrated that carboplatin, combined with dual HER2 blockade (pertuzumab + trastuzumab) plus docetaxel, was more effective than the same regimen without carboplatin in neoadjuvant treatment for HER2-positive breast cancer [63]. A third meta-analysis, conducted on 2111 patients with advanced or metastatic urothelial carcinoma (AMUC), demonstrated that cisplatin and carboplatin were similar to immune checkpoint inhibitor (ICI) monotherapy in terms of OS and pCR, but both platinum-based drugs had better objective response rates (ORR) than ICI therapy [64].
Nephrotoxicity observed with cisplatin occurs less frequently with carboplatin, with rates of 20%–30% for cisplatin vs. 10%–15% for carboplatin. This difference is likely due to structural changes in carboplatin, which result in less accumulation and thus reduced nephrotoxicity [65]. However, two recent studies evaluating a total of 362 patients found no significant difference in nephrotoxicity between cisplatin and carboplatin treatments [66,67]. Another study suggest that the risk of nephrotoxicity increased with age, particularly in patients treated with cisplatin compared to carboplatin, due to the natural decline in nephron number and size with age, which makes older individuals more susceptibility to nephrotoxicity [65].
Oxaliplatin was approved for clinical use by the FDA in 2002 and is indicated for cancers where resistance to cisplatin or carboplatin has developed. It is primarily used in the treatment of colorectal cancer, often in combination with other drugs such as 5-fluorouracil and leucovorin (FOLFOX regimen), FOLFOX plus irinotecan (FOLFOXIRI regimen); or oxaliplatin combined with capecitabine (CAPEOX regimen); among others [60].
The mechanism of oxaliplatin’s entry into the cell is similar to cisplatin, primarily through passive diffusion or via the CTR1 transporter [60]. Once inside, unlike cisplatin or carboplatin, oxaliplatin inhibits RNA Pol I, leading to rRNA silencing and nucleolar disruption, likely mediated by the DNA damage response kinases Ataxia Telangiectasia Mutated (ATM) and ATR serine/threonine kinase (ATR) [68].
Although oxaliplatin has fewer side effects than cisplatin, it can cause a neuropathy known as oxaliplatin-induced peripheral neurotoxicity (OIPN), which may lead to treatment interruption, though the condition can be reversible upon cessation of treatment. OIPN is thought to arise from DNA damage in sensory neurons, dysfunction of voltage-gated ion channels, increased pro-inflammatory response in certain neurons and peripheral nerves, oxidative stress from DNA adducts in neurons, and eventual neuronal apoptosis [69].
As noted, oxaliplatin is the treatment of election for colorectal cancer, often in combination with other drugs. A recent meta-analysis involving 795 patients demonstrated that the FOLFOX regimen was superior to fluorouracil plus leucovorin (IFL) or even oxaliplatin combined with irinotecan (IROX), in terms of median time to progression, response rate, and median survival time for the treatment of metastatic colorectal cancer [70]. Another meta-analysis, which included 4571 patients, compared regimens containing oxaliplatin and irinotecan for treating metastatic colorectal cancer, finding no statistically significant differences between the two treatment groups in terms of OS, progression-free survival (PFS), or ORR [71].
A recent study from the National Surgical Adjuvant Breast and Bowel Project (NSABP), known as the R-04 trial (NCT00058474), evaluated oxaliplatin’s toxicity in 1132 rectal cancer patients. These patients were randomized into four groups: 5-FU alone (n = 277), 5-FU + oxaliplatin (n = 286), capecitabine alone (n = 283), and capecitabine plus oxaliplatin (n = 286). The study found that oxaliplatin in combination with either 5-FU or capecitabine was less tolerable than either chemotherapy alone [72], suggesting that a careful balance between tumor control and side effects must be considered.
Next to the development of cisplatin (first generation), carboplatin, and oxaliplatin (second generation), a third generation of platinum-based drugs was developed. Among them, is nedaplatin (Table 1), which retains chemical similarities to cisplatin and carboplatin but offers improved anticancer and fewer side effects. Developed to enhance the water solubility of cisplatin and reduce its adverse effects while maintaining efficacy, nedaplatin is currently used clinically in Japan. It has demonstrated notable anticancer activity against cervical, small- and non-small cell lung, breast, ovarian, and testicular cancers, although it has not yet been widely accepted globally [73]. A study evaluating hypersensitivity to nedaplatin in 31 patients with carboplatin hypersensitivity showed a response rate of 71.4% in the nedaplatin-treated group, compared to 30.0% in the no-treated group. Only one patient in the nedaplatin group experienced a hypersensitivity reaction, demonstrating that nedaplatin is both safe and effective in this context [74].
Lobaplatin (Table 1) is another third-generation platinum-based drug, which appears to have lower nephro- and ototoxicity than its predecessors. Currently, it is only available in China. Lobaplatin was tested in bladder cancer cell lines T24 and 5637, showing IC50 values of 11.62 µg/mL and 9.61 µg/mL at 24 h, respectively. Flow cytometry revealed that lobaplatin induced apoptosis in 31.25% of T24 cells, compared to 6.25% in the control group, and 14.3% in 5637 cells, compared to 2.5% in the control. The study indicated that apoptosis was mediated by the regulation of Bcl-2 and BAX expression and inhibition of the PI3K/Akt signaling pathway [75].
Heptaplatin (Table 1), another third-generation platinum-based drug, was developed in South Korea and approved by the Korean FDA in 1999. Heptaplatin forms DNA adducts, altering transcription and replication processes, thereby inducing apoptosis [76]. A recent article explored supramolecular chemotherapy using heptaplatin and cucurbit-7uril (heptaplatin-CB7) to treat colorectal cancer cells. The results showed that heptaplatin-CB7 induced a notable percentage of early apoptosis in HCT116 and HT29 cells, and induced an inhibitory response in the G1 phase of the cell cycle in these cells [77].
The last third-generation platinum-based drug discussed here is miriplatin (Table 1), designed and synthesized in Japan for the treatment of unresectable hepatocellular carcinoma. Due to its poor solubility in water and organic solvents, miriplatin is unsuitable for intravenous administration [78]. In a recent study, miriplatin was encapsulated in a liposomal formulation called lipomiriplatin (LMPt) and tested in human pancreatic cancer cell lines (AsPC-1, BxPC-3, MIA-PaCa-2, PANC-1, and SU.86.86). The inhibitory effect of LMPt on these cancer cells was 2.70 to 16.74 times greater than that of miriplatin alone, with IC50 values ranging from 0.28 to 19.81 μmol/L. In comparison, oxaliplatin, used as a control, showed IC50 values greater than 75 μmol/L, indicating that LMPt is more effective than oxaliplatin. Additionally, EdU (a thymidine analog) was used to evaluate LMPt’s ability to inhibit DNA replication and cell proliferation, demonstrating superior activity compared to oxaliplatin in pancreatic cancer cells. In vivo, LMPt was tested in an AsPC-1 mouse xenograft model, where it exhibited a 31.36% inhibitory effect, approximately eight times greater than of miriplatin alone [78].
Despite their promising results, including chemical stability, low toxicity, and potent anticancer activity, even in cisplatin-resistant cancer cells, nedaplatin, lobaplatin, heptaplatin, and miriplatin have not been used or evaluated in Western countries. Economic factors may play a role in limiting their entry into Western healthcare markets, though the exact reasons remain unclear.
Ruthenium-based molecules represent a large family of compounds with unique properties that make them promising candidates for cancer treatment. These properties include the ability to slowly exchange ligands with biologically important molecules like glutathione and certain proteins, and the capacity to exist in multiple oxidation states—Ru(II), Ru(III), and Ru(IV)—under physiological conditions. This allows some ruthenium complexes to be administered as a prodrug in the Ru(III) or Ru(IV) states and then reduced to the more reactive Ru(II) state in the tumor environment, thereby enhancing their cancer effectiveness [79,80]. Additionally, ruthenium can be transported throughout the body by plasma proteins such as albumin and transferrin, facilitating its distribution [81]. Once in circulation, ruthenium complexes can enter cells through transferrin receptors or death receptors, triggering apoptosis [82]. Some ruthenium complexes are internalized via endocytosis of transferrin receptors, while others may enter cells through passive diffusion [83].
Regarding the mechanism of action of ruthenium complexes, they can bind to DNA, disrupting DNA replication and RNA transcription, and can also stably bind to the G-quadruplex structure of telomeric DNA, interfering with telomerase activity. Additionally, ruthenium complexes can inhibit topoisomerases, which play key roles in DNA metabolism, leading to the initiation of apoptosis [80,84]. Recent studies have demonstrated that certain ruthenium complexes, such as [Ru(dip)2(PPβC)]PF6 (Table 2) and [Ru(phen)2(PPβC)]PF6, accumulate in mitochondria and inhibit mitochondrial DNA topoisomerase I, thereby inducing caspase-mediated apoptosis [85]. Another study highlighted a novel Ru(II) complex, [Ru(U)2(H2O)2]Cl3 (U = 5,6-Diamino-1,3-dimethylpyrimidine-2,4(1H,3H)-dione), which induces apoptosis by downregulating nuclear topoisomerase I expression [86]. Other studies have reinforced the observation that ruthenium complexes preferentially inhibit DNA topoisomerase I in cancer cells [87,88]. Similar results were shown with a thiomaltol-based [Ru(II)]PF6 complex, which has been shown to induce apoptosis in breast cancer cells by inhibiting DNA topoisomerase IIα, with a particular affinity for the DNA-binding pocket of the enzyme [89].
One of the most remarkable characteristics of ruthenium compounds is their ability to be photoactivated by light of specific wavelengths. This opens up possibilities for the development of ruthenium-based drugs for photodynamic therapy or photoactivated chemotherapy. In these therapies, the molecules can be selectively activated in the tumor area, thereby minimizing damage to healthy tissues. Over the past two years, several photoactivable ruthenium compounds have been developed for cancer treatment. For example, [Ru(phen-PPh3)2(1-Py-βC)](PF6)4 and [Ru(phen)2(1-Py-βC)](PF6)2, were shown to increase cytotoxicity by 50-100 times upon photoactivation in breast cancer MDA-MB-231 cells [90]. Other ruthenium complexes, such as cis-[Ru(dcbpyH)2(PTAH)2]Cl2, cis-[Ru(bpy)2(PTA)2]Cl2 and trans-[Ru(bpy)2(PTA)2](CF3SO3)2, exhibited 4- to 10-fold increase in cytotoxicity upon photoactivation in lung cancer A549 and prostate adenocarcinoma PC-3 cells [91]. Complexes Ru1−Ru5 of general formula [Ru(phen)2(N∧N′)]2+, being CH3 (Ru1), F (Ru2), CF3 (Ru3), NO2 (Ru4), and N(CH3)2 (Ru5) substituents in the phenyl ring, showed a 7- to 15-fold increase in cytotoxicity in cervical cancer HeLa cells and a 7- to 29-fold increase in cytotoxicity in A375 melanoma cells upon photoactivation [92]. Furthermore, the molecule Ru-Cyn-1 (Table 2) demonstrated a 106-fold increase in cytotoxicity when photoactivated in colon cancer CT26 cells [93].
At the molecular level, several genes are affected by ruthenium complexes. For example, the ruthenium complex RXC has been shown to downregulate the expression of genes encoding the chaperone Hsp90 in HCT116 colorectal cancer cells, along with downstream effectors of Hsp90, including Akt1, Akt (pS473), mTOR (pS2448), 4EBP1 (pT36/pT45), GSK-3β (pS9) and NF-κB p65 (pS529). This suggests that RXC induces cell death through inhibition of the AKT/mTOR pathway [94]. Similar results were observed with a ruthenium complex bound to 5-fluorouracil (Ru/5-FU), which inhibited the expression of Akt1 and Akt (pS473), mTOR (pS2448), S6 (pS235/pS236), 4EBP1 (pT36/pT45), GSK-3β (pS9) and NF-κB p65 (pS529) in HCT116 cells, further indicating inhibition of the Akt/mTOR pathway as a mechanism of action [95]. Another compound, RuZ2, demonstrated an IC50 of 4.05 μM in ovarian carcinoma SKO3CR cells. RuZ2 upregulated beclin-1, PINK1, Parkin, cleaved-caspase-3, caspase-9, and cytochrome c, while downregulating FNUDC1 and p62, suggesting a mechanism of cell death via mitophagy related-apoptosis [96]. Similarly, the ruthenium complex Ru-UCN1 was found to induce apoptosis in AGS cells by overexpressing p53, PUMA, and caspase-3 [97]. Another ruthenium compound, [Ru(η6-anethole)(en)X]PF6I, also induced significant overexpression of pro-apoptotic genes as caspase-3, PUMA, and DIABLO in AGS cells, supporting a mechanism of apoptosis similar to that seen with other ruthenium compounds [98]. Continuing this trend, another ruthenium compound, named complex-6, was shown to activate caspase-9 in dose- and time-dependent manner, along with up-regulation of BAX and cleaved-caspase-3, and downregulation of Bcl-2 in NCI-H460 lung cancer cells. This suggests that complex-6 induces caspase-mediated apoptosis through the intrinsic mitochondrial pathway in these cells [99]. A ruthenium biochanin-A compound has also been shown to induce apoptosis and cell cycle arrest in A549 lung cancer cells by activating caspase-3, while downregulating PI3K, TNF-α, TGF-β, and PPARγ in a dose-dependent manner [100]. Interestingly, this same compound was tested in vivo in Balb/c mice and was found to upregulate p53 and caspase-3 expression, while downregulating Bcl-2 in lung tissue [94]. Additionally, another ruthenium compound, a dendrimer named CRD13, was tested in Balb/c mice injected with 4T1 breast cancer cells. After 28 days of treatment with CRD13, tumor size decreased by nearly 50% compared to untreated control animals, and the ruthenium dendrimer did not affect the weight of mice [101].
An interesting ruthenium compound, NKP-1339, has undergone both preclinical and clinical testing. The biodistribution of this molecule was evaluated at different times in Balb/c mice bearing CT26 allograft (a murine colon cancer cell line). The study revealed that NKP-1339 initially accumulated in the serum within the first 4 h, after which its concentration gradually decreased. The kidneys and liver also accumulated the compound, likely due to their role in excretion and clearance. Only trace amounts of ruthenium were found in the brain, bones, and muscles up to 72 h post-administration. While ruthenium concentrations decreased in all organs over time, the concentration remained elevated in the kidneys. Notably, the tumor tissue exhibited higher ruthenium concentration at 24, 48, and 72 h compared to the initial 4 h, indicating preferential accumulation in the tumor over time [102].
To date, only a few ruthenium compounds have progressed to clinical trials as antitumor agents, including NAMI-A, KP1019, KP1339, and TLD1433 (all four in Table 2) [103]. These trials, which are primarily in Phases I or II, aim to determine maximal tolerable dose, dose-limiting toxicities, antitumor efficacy, as well as pharmacokinetics and pharmacodynamics of these ruthenium compounds. Details of these clinical studies will be covered in a separate section.
Copper is an essential metal involved in various redox processes and has emerged as a promising candidate for the development of new anticancer drugs. Copper can damage DNA, arrest the cell cycle, and induce apoptosis in tumor cells. Additionally, copper depletion has been shown to suppress angiogenesis, thereby inhibiting the neovascularization tumor of tumors [104,105].
Copper is primarily ingested in the form of Cu(II), which must be processed by intestinal cells for use by the body. These cells contain reductases on their surface, that reduce Cu(II) to Cu(I), facilitated by divalent metal transporters 1 (DMT1), while the copper transporter protein (CTR1) helps Cu(I) enter the cell. Once inside, it binds to the antioxidant chaperone protein 1 (ATOX1), which directs copper to the transporter proteins ATP7A and ATP7B. Copper is then bound to ceruloplasmin, which distributes it to various organs and tissues [106].
Intracellular copper concentrations must be tightly regulated, as elevated levels can lead to cytotoxicity and apoptosis. The FDX1 protein plays a crucial role in copper-induced cell death by acting as a reducing factor that converts Cu(II) to the more toxic Cu(I) form, increasing intracellular copper concentrations. This leads to the rapid generation of reactive oxygen species (ROS), which causes oxidative stress and ultimately triggers apoptosis [107,108].
Elesclomol (Table 3), a copper ionophere, has demonstrated high efficiency in inhibiting colorectal cancer cells (SW480) by inducing copper overload. This molecule degrades ATP7A, preventing copper from being transported and eliminated from the cells. The resulting accumulation of ROS diminishes the transporter responsible for GSH production, ultimately leading to ferroptosis in the cells [109]. Ghasemi et al. [110] studied the effect of copper nanoparticles (CuNPs) on the same SW480 colon cancer cells, showing that CuNPs induce cytotoxicity and ROS-mediated apoptosis, this was accompanied by increased expression of proapoptotic proteins BAX and P53 and decreased expression of Bcl-2. Similarly, in the breast cancer cell line (MCF-7), CuNPs were found to increase the expression of P53 and BAX, and the activation of caspase-8 and -9, suggesting that CuNPs trigger apoptosis through both intrinsic and extrinsic pathways [111].
In addition to inducing oxidative stress, copper, like other metals, can interact with DNA and cause genetic damage due to its reactive and redox-active nature, which allows it to bond with various functional groups. Researchers have explored these characteristics by binding copper with ligands such as 5-fluoracyl and phenanthrolines to enhance their anticancer properties [112,113]. For example, two Cu(II) complexes [Cu (bpy)2L1] BF4·CH3OH and [Cu(phen)2L1] BF4·H2O, with L1 = 5-fluorouracil-1-yl acetic acid (Table 3), were studied as anticancer agents against colon cancer cells (HCT116) and triple-negative breast cancer cells (TNBC) (MDA-MB-231), demonstrating cytotoxic effects on both cell lines. This study also showed that DNA binding by Cu(II) is enhanced when conjugated to 5-fluorouracil [113]. In an effort to reduce the side effects of copper-based molecules, researchers have formulated ligands that are selective for tumor cells. One example is the development of curcumin-derived ligands, as curcumin itself has demonstrated anticancer properties. Curcumin-copper complexes have shown cytotoxic effects in breast cancer cells, with IC50 ranging between 2.3–7.1 μM, significantly lower than carboplatin (IC50 = 359.3 μM). Moreover, these compounds were tested in human umbilical vein endothelial cells (HUVECs), showing a higher IC50, which indicates lower cytotoxicity compared to carboplatin, making them promising alternatives with selective toxicity for cancer cells [114].
Recent research has explored the effects of copper compounds on other types of cancer cells. Machado et al. [115] tested the cytotoxic activity of copper in OVCAR3 ovarian and PC3 prostate cancer cells, finding high anticancer activity in both cell lines. In PC3 cells, these copper compounds were 7 to 22 times more active than cisplatin. When investigating the mechanism of cell death in OVCAR3 cells, the induction of apoptosis was indicated as the main mechanism, ruling out oxidative stress as a contributing factor.
Additionally, two copper complexes, [Cu(Trop)Sac] and [Cu(Trop)Cl] (Table 3), which contain a tropolone structure along with saccharin or chlorine, have been studied for their anticancer effects. These compounds were tested in colon cancer cells, where the chlorine-containing molecule [Cu(Trop)Cl] exhibited antiproliferative effects, a property not observed with the saccharin-containing molecule [Cu(Trop)Sac]. However, in migration assays, both molecules inhibited cancer cell movement, with [Cu(Trop)Sac] demonstrating a stronger effect. This suggests that both [Cu(Trop)Sac] and [Cu(Trop)Cl], hold potential as therapeutic agents for reducing invasiveness and metastasis in colon cancer [116].
Since the FDA approved the gold compound auranofin (Table 4) for the treatment of rheumatoid arthritis in the 1980s, interest in gold compounds for therapeutic applications has significantly increased [117]. Gold(I) and gold(III) ions can be conjugated to various atoms such as nitrogen, phosphorus, selenium, carbon, and others, resulting in complexes with diverse geometric configurations. The multiple oxidation states, high electronegativity, and electron affinity of gold make its complexes promising candidates for cancer therapy. These complexes can modulate immune responses, inhibit key enzymes involved in cell proliferation, and induce apoptosis [118–120].
Gold(I) compounds have a lower affinity for DNA but show a strong preference for sulfhydryl, thiol, and selenocysteine groups in important protein targets [121,122]. For instance, gold(I) interacts with the thiol and selenol groups of thioredoxin reductases (TrxRs), inhibiting their normal function. Since TrxRs are critical for maintaining cellular redox homeostasis, their inhibition leads to elevated intracellular ROS levels, oxidative stress, and ultimately, apoptosis [123]. Gold(I) compounds also bind to the sulfhydryl groups of DNA polymerases, impeding their action and reducing the acuracy of DNA replication, triggering programmed cell death. While gold(III) compounds also induce apoptosis, they do so through different mechanisms. In physiological conditions, ligands such as diphosphines, terpyridines, dithiolates, and diamines stabilize gold(III). Notably, terpyridine-based gold(III) complexes form strong, irreversible bonds with DNA, a key mechanism underlying their potent antiproliferative effects in cancer cells [124].
Studies have shown that [C^N]Au(III) cyclometalated gold compounds with a norbornane scaffold and secondary diamines exhibit antiproliferative effects on ovarian cancer cells. These compounds demonstrate high toxicity toward glycolysis-dependent cells compared to cells reliant on oxidative phosphorylation, suggesting that, unlike other metal-based drugs, they do not cause damage to mitochondria [125]. Other research has explored gold compounds as a means of overcoming cisplatin resistance in ovarian cancer cells. In a recent study, the cytotoxicity of four molecules [Au(mnt)2] −, [Au(i-mnt)2] −, [Au(cdc)2] − and [Au(qdt)2] − was evaluated in ovarian cancer cell lines A2780 and A2780cisR (cisplatin resistant). After 48 h of exposure, the IC50 values ranged from 0.9 to 5.5 μM in both cancer cell lines, demonstrating strong cytotoxic effects [126].
Another study conducted on MDA-MB-231 cells, a model of TNBC, revealed that the gold compound Au4BC (Table 4) exhibited cytotoxic effects, causing a dose-dependent reduction in cell viability. This was accompanied by a significant increase in γ-H2AX levels, a marker of DNA damage that is recruited to double-strand break sites to facilitate DNA repair, as well as an increase in ROS levels. These findings suggest that Au4BC may induce cell death by modulating ROS levels within the cells [127]. Similarly, AuPhos-89 (Table 4), a gold(III)-bisphosphine complex, demonstrated alterations in biological pathways related to inflammation and mitochondrial function in MDA-MB-231 cells. AuPhos-89 appears to induce apoptosis by modulating oxidative phosphorylation and redox pathways, impacting mitochondrial metabolism. In vivo studies using a murine model of TNBC showed that AuPhos-89 significantly inhibits tumor growth, positioning it as a promissory compound for cancer treatment [128]. Other molecules, such as alkylgold(III) compounds, have also shown cytotoxic effects in MDA-MB-231 and MCF-7 breast cancer cell lines, particularly when irradiated with UV light. The cytotoxicity of these photoreactive alkylgold(III) compounds is significantly enhanced in the presence of light, suggesting a light-dependent activation mechanism. This ability to selectively activate compounds using UV light offers greater precision in drug delivery, potentially reducing systemic toxicity and improving therapeutic efficacy [129].
Giuso et al. [130] evaluated the anticancer potential of four binuclear biphenyl organogold(III) complexes, of general formula [(C^C)Au(Cl)(L^L)(Cl)Au(C^C)] (Table 4), across various cellular models. Notably, one of these complexes demonstrated potent antiproliferative effects in A549 lung cancer, MDA-MB-231 breast cancer, and A2780 ovarian cancer cells, with IC50 values of 0.13, 0.20 and 0.07 μM, respectively. The most surprising finding was that these compounds significantly lower cytotoxicity against non-cancer cells compared to cisplatin, which is a highly desirable feature in anticancer compounds.
Despite the growing body of research on gold complexes, further studies are needed, particularly in preclinical models, followed by clinical trials to fully assess their therapeutic potential.
Some examples of the anti-cancer activity of gold-based compounds can be seen in Table 4.
Palladium(II) and platinum(II) species show certain structural and thermodynamic similarities, making palladium(II) complexes an interesting alternative to platinum-based drugs. Recent studies have shown that several palladium(II) complexes exhibit greater bioactivity compared to platinum(II)-based drugs. Moreover, these palladium(II) complexes have demonstrated low toxicity and high specificity [131]. Palladium complexes could bind strongly with DNA via the minor groove and show good binding affinity for bovine serum albumin (BSA), which may facilitate their targeted delivery to tumors [132–134].
Palladium-based nanomaterials also exhibit strong absorption in the near-infrared (NIR) region, with high photothermal conversion efficiency, photothermal stability, and biocompatibility. These properties make palladium-based compounds plausible candidates for photothermal therapy, where the drugs are activated by light to selectively target cancer cells [135].
Palladium(II) complexes with thioamide ligands have shown potential as anticancer agents. The presence of aromatic N-donor motifs enhances their activity, while sulfur offers diverse coordination possibilities for metal centers, contributing to their effectiveness [136,137]. Palladium complexes are thought to be less toxic than cisplatin. For example, the median lethal dose (LD50) of cisplatin administered orally to rats is approximately 270 mg/kg, whereas the LD50 for palladium is over 2700 mg/kg, indicating tenfold lower toxicity [136].
Palladium is capable of inducing mitochondrial dysfunction through the increase of mitochondrial ROS, alteration of membrane potential, and release of cytochrome c. This mitochondrial disturbance can alter oxidative phosphorylation in cells treated with palladium complexes, thereby affecting cell viability and increasing the likelihood of apoptosis [138].
One study evaluated the cytotoxicity of various palladium(II) triphenylphosphine complexes of thioamides against the human prostate cancer cell line, PC3. In particular, the complexes [Pd(Tu)2(PPh3)2]Cl2, [Pd(Dmtu)2(PPh3)2]Cl2, and [Pd(Mpm)2(PPh3)2]Cl2 exhibited notable cytotoxic activity, with IC50 values of 18.30, 5.80, and 8.17 µM, respectively, which are desirable for antitumor agents [139].
Another study on breast cancer cell lines, MCF7, MC4L2, and 4T1, demonstrated that the palladium compound L2PdCl, containing thioamide ligands, was capable of inhibiting cell proliferation with an IC50 value of 20 µM across all cell lines after 48 h of treatment. Two other palladium compounds were also evaluated, but their IC50 values ranged from 40 to 100 µM [136].
A recent article evaluated a photoactivable palladium compound, PdL (Table 5), in tumor xenografts of human skin melanoma (A375) in nude mice. PdL showed minimal inhibition of tumor growth in the dark; however, when the animals were irradiated with green light 12 h after drug injection, tumor growth was strongly inhibited. Additionally, PdL exhibited low cytotoxicity in healthy organs, indicating its potential for selective cancer treatment [140].
Another recent study evaluated the anticancer potential of several palladium-based drugs in vitro and in vivo in lung adenocarcinoma models. Palladium compounds were tested in A549 and Spc-A1 lung cancer cell lines, with IC50 values ranging from 2 to 26 µM, while in the normal cell line 293T, the IC50 values ranged from 119 to 172 µM. Notably, a compound called 9a (Table 5) showed IC50 values of 2.4 and 7.28 µM in A549 and Spc-A1 cell lines, respectively, compared to 130.56 µM in 293T cells, demonstrating selective toxicity towards cancer cells. Flow cytometry analysis confirmed that compound 9a induced apoptosis in lung cancer cells. Furthermore, 9a activated caspase-3 and -9, and downregulated the antiapoptotic gene Bcl-2. In in vivo studies, animals treated with compound 9a showed a six-fold decrease in tumor weight, highlighting its potential as a promising anticancer agent [141].
A coumarin-palladium(II) complex, designated as C1, was evaluated against the pancreatic carcinoma cell line PANC-1. The IC50 value for C1 in these cells was 3.39 µM. Flow cytometry showed that treatment with 5 μM of the C1 complex induces 10% necrosis and 20% late apoptosis in the cells. In comparison, the control drug doxorubicin caused four times the level of cell death due to necrosis. Additionally, C1 significantly upregulated BAK and downregulated Bcl-2 in PANC-1 cells, markers of apoptosis. Finally, in an in vivo zebrafish-PANC-1 xenograft model, treatment with 0.5 μM of C1 resulted in a tumor mass reduction of approximately 60%, demonstrating the compound’s potent anticancer activity [142].
Some examples of the anti-cancer activity of palladium-based compounds can be seen in Table 5.
Zinc plays an essential role in nutrition and in human health, with a recommended daily intake of 6.7 to 15 mg per day [143]. The FDA has approved zinc oxide (ZnO) as a safe metal oxide, which is less toxic than many other metals and exhibits low reactivity with active pharmaceutical ingredients. The pharmacokinetic properties and biodistribution of ZnO nanoparticles in vivo can be enhanced using nanotechnology; studies have shown that orally administered ZnO tends to accumulate in the liver, kidneys, and lungs. The primary route of ZnO excretion is through feces, while smaller particles are excreted via urine [144].
In cancer patients, serum zinc levels often decrease significantly, disrupting zinc metabolism. This reduction has been observed in a variety of cancers, including breast, ovarian, gastrointestinal, lung, thyroid, and esophageal cancers [145].
ZnO nanoparticles have been associated with anticancer activity, likely through the generation of ROS, which activates the release of apoptotic factors from mitochondria, ultimately inducing cell death. Additionally, ZnO nanoparticles can interact with the negatively charged environment of tumor cells, a characteristic of the Warburg effect. ZnO nanoparticles release Zn(II) ions into the cell nucleus, where they can interact with DNA and RNA, disrupting processes such as replication and transcription [146].
The first evidence of the antiproliferative effects of zinc in cancer was reported in the late 1990s when Liang and colleagues found that physiological levels of zinc inhibited cell growth in dose-dependent prostate cancer cell lines LNCaP and PC-3. Their study demonstrated that zinc induces G2/M phase cell cycle arrest through the overexpression of p21 [147].
Since then, several in vivo and in vitro studies have confirmed the effectiveness of zinc compounds as anticancer agents and tumor suppressors, primarily through the modulation of growth-related genes and signaling pathways [148].
Previous studies using MTT assays, cell clone formation, Hoechst staining, and flow cytometry have demonstrated that exposure of PC-3 prostate cancer cells to zinc can enhance the antitumor activity of paclitaxel (an anti-microtubule taxane) by nearly 50%. This effect is believed to be mediated through the mitochondria-dependent apoptosis pathway. Additionally, an increase in the activation of caspase-3 and caspase-9 was observed, along with a reduction in the Bcl-2/BAX expression ratio, all hallmark characteristics of apoptosis [149]. Subsequent studies further confirmed that the combination of paclitaxel and zinc affects cell proliferation in a dose- and time-dependent manner in PC3 and DU145 prostate cancer cells. This drug combination has also been shown to inhibit prostate cancer cell invasion and migration by downregulating the expression of TWIST1, a transcription factor involved in embryonic development and cancer progression [150].
In more recent research, ZnO nanoparticles were synthesized in combination with Rubus fairholmianus root extract (named RFZnO) to investigate their synergistic cytotoxic effects on breast cancer MCF-7 cells. Rubus species have been used in traditional medicine due to their diverse pharmacological properties, and previous studies have reported the in vitro cytotoxic effects of R. fairholmianus on colorectal, breast, and lung cancers. The results demonstrated that RFZnO nanoparticles significantly increased cytotoxicity in MCF-7 cells (5.22-fold) compared to untreated controls. Additionally, there was a marked increase (4.57-fold) in cytochrome c release in the treated cells. RFZnO nanoparticles also induced the overexpression of BAX and p53, while downregulating Bcl-2 at both the mRNA and protein levels. These findings suggest that RFZnO nanoparticles induce apoptosis in breast cancer cells through a mitochondria-mediated, caspase-dependent apoptotic pathway [151].
As mentioned earlier, various natural extracts can reduce the adverse effects of metal-based drugs while also enhancing their anticancer activities. In one study, the cytotoxic and antitumor effects of zinc oxide nanoparticles (ZnO NPs), prepared with pure curcumin (Green-ZnO-NPs), were evaluated on the breast cancer cell line MCF-7. The results showed a significant reduction in MCF-7 cell viability, with IC50 values of 23.54 and 20.53 μg/mL at 24 and 48 h, respectively, demonstrating an increase in activity over time. Curcumin itself did not show anticancer activity before 24 h of treatment, indicating that it enhanced the anticancer activity of the zinc nanoparticles.
A recent study also assessed the effect of ZnO, among other compounds, on the efficacy of cisplatin in MCF-7 cells. The IC50 value for cisplatin alone was 9.1 μg/mL, whereas the IC50 for the ZnO/cisplatin complex was 2.12 μg/mL at 24 h, more than 4 times lower than cisplatin alone. Furthermore, flow cytometry analysis showed that the ZnO/cisplatin complex induced higher early apoptosis (67.02%) compared to the cisplatin control (53.59%). Additionally, a chitosan-ZnO/cisplatin complex exhibited a higher level of late apoptosis (57.75%) compared to the cisplatin control (35.96%), demonstrating that ZnO can significantly enhance the anticancer activity of cisplatin [152].
Although the precise mechanism of ZnO nanoparticle cytotoxicity remains unclear, the most widely accepted theory involves the intracellular release of Zn(II) ions, along with generation of ROS [153]. This mechanism, together with changes in the expression of pro- and anti-apoptotic genes, likely contributes to the observed cytotoxic effects.
Recently, iridium(III) compounds have shown a great potential as anticancer drugs, since they can interact with DNA and induce alteration of mitochondrial function, in addition to enhancing the generation of reactive ROS, inducing endoplasmic reticulum stress and apoptosis [154,155]. Another interesting feature of iridium(III) is its ability to absorb and emit light, making it a good candidate for targeted anticancer photodynamic therapy [156]. In this regard, a recent article evaluated the photoactivity of two iridium(III) compounds, [Ir(ppy)2(BDIP)](PF6) (Table 5) and [Ir(ppy)2(MDIP)](PF6), which were tested on A549 lung cancer cells. When cells were treated for 48 h, the IC50 values were 4 and 26.8 μM, respectively. However, when cells were treated with these iridium compounds and exposed to white light for 48 h, the IC50 values were 0.7 and 1.8 μM, respectively, which represent a considerable improvement in the activity of these compounds. The IC50 of cisplatin in these cells was 6.6 μM, indicating that these photoactivable iridium compounds could be excellent drugs for targeted therapy [154].
Another recent study performed in A549 lung cell cancer, B16 melanoma, and HCT116 colorectal cancer cells, showed that [Ir(piq)2Cl]2 (Table 5) has potent antiproliferative activity with IC50 values of 2.2, 2.5, and 2.5 μM, respectively, while the IC50 for cisplatin in these cells was 6.1, 28.8, and 15.3 μM, respectively. Again, these iridium compounds show significantly greater antitumor activity compared to cisplatin in the treated cells. Furthermore, it was determined that this iridium compound is capable of affecting mitochondrial function opening the mitochondrial permeability transition pore, elevating ROS, and inducing a release of cytochrome c, which causes ferroptosis and pyroptosis. Finally, this iridium compound was tested in vivo in Balb/c nude mice xenotransplanted with A549 cells, demonstrating that it was able to induce a tumor inhibition rate of 34.04% with the treatment of 3.2 mg/kg and of 58.58% with 5.0 mg/kg of drug [157].
In other studies, the A549 cells were treated with the iridium compound [Ir(bzq)2PPA]PF6 (Table 5) showing an IC50 of 1.6 μM, while the IC50 values for cisplatin and oxaliplatin were 18.6 and 13.5 μM, respectively. This indicates that the iridium compound increases cytotoxicity in A549 cells more than 10-fold compared to traditional platinum-based chemotherapy [158]. This increased activity opens up an interesting opportunity to develop more drugs of this type that allow the use of low doses with greater potential.
Some examples of the anti-cancer activity of iridium-based compounds can be seen in Table 5.
Clinical trials with platinum drugs
Despite the increasing exploration of alternative therapies, hundreds of studies published last year alone highlight ongoing clinical trials using platinum-based drugs, typically in combination with other treatments, for cancer therapy.
In particular, cisplatin is widely used as a chemotherapy drug due to its good results and low cost, however, many cancer patients show partial or no response when treated with cisplatin for a long time and develop resistance. This cisplatin resistance is partly due to the hypoxic tumor microenvironment (TME), which results in elevated expression of HIF-1α, which is involved in the induction of angiogenesis, changes in the glucose metabolism, cell proliferation, inhibition of apoptosis, invasion and metastasis, finally leading to an adaptation to the hypoxic TME [159].
Platinum drugs combined with etoposide and anti–PD-L1 therapies currently serve as the standard first-line treatment for extensive-stage small cell lung cancer (ES-SCLC). In a study involving 457 patients, 227 received tislelizumab (a human anti–PD–1 antibody) alongside chemotherapy (180 patients with etoposide plus carboplatin and 47 with etoposide plus cisplatin), while 230 patients received placebo plus chemotherapy (181 with etoposide plus carboplatin and 49 with etoposide plus cisplatin). The results showed that tislelizumab, when combined with platinum-etoposide chemotherapy, significantly reduced the risk of death and improved PFS, ORR, and duration of response (DoR). These findings suggest that tislelizumab plus chemotherapy could become the new first-line treatment for ES-SCLC patients [160].
In another recent study involving 1214 patients with non-small cell lung cancer (NSCLC), the efficacy of avelumab (a human anti-PD-L1 IgG1 antibody) was evaluated in 688 patients, compared with 526 patients receiving platinum-based doublet chemotherapy. The differences in OS and PFS between the two groups were not statistically significant, indicating that platinum-based therapy remains the best option for patients with high-expression PD-L1+ NSCLC [161].
Additionally, for patients with EGFR-mutated metastatic NSCLC, the combination of nivolumab (another human anti-PD-1 antibody) with platinum-based doublet chemotherapy (cisplatin or carboplatin) was evaluated in 141 patients, compared with platinum chemotherapy alone in 143 patients. No significant differences were found in PFS, ORR, or DoR between the groups, further confirming that platinum-based chemotherapy is a viable and effective option for treating metastatic NSCLC [162].
In an interesting study, selenium yeast, a feed additive, was evaluated for its potential to prevent adverse effects related to platinum-based combination therapy in patients with malignant tumors. Selenium yeast, a selenium supplement, is primarily used to promote the well-being of patients with tumors, and cardiovascular, or cerebrovascular diseases. In this study involving 86 patients, 43 received platinum treatment (cisplatin or carboplatin) combined with selenium yeast at a dose of 200 μg daily, while the other 43 received platinum treatment without selenium yeast. The results indicated that patients supplemented with selenium yeast experienced a significant reduction in adverse reactions associated with platinum therapy. Benefits included improved appetite, prevention of weight loss, and significant pain relief, making selenium yeast a promising adjunct for managing the side effects of platinum-based chemotherapy without compromising its efficacy [163].
In Phases I/II study, the efficacy of dendritic cell (DC) vaccination combined with carboplatin/paclitaxel was evaluated in 5 patients with metastatic endometrial cancer (mEC). The results demonstrated that DC vaccination can be safely combined with carboplatin/paclitaxel chemotherapy in these patients, suggesting a potential new synergistic treatment option with promising outcomes [164].
In a retrospective study involving 198 patients with ovarian cancer, different chemotherapy regimens were evaluated. Of these, 92 patients (46.5%) received gemcitabine plus carboplatin, 76 patients (38.4%) received paclitaxel plus carboplatin, and 30 patients (15.2%) received gemcitabine plus cisplatin combined with bevacizumab. The group that received gemcitabine, cisplatin, and bevacizumab had the highest overall survival (OS) compared to the other groups, suggesting a potential benefit of this combination therapy [165].
Clinical trials with ruthenium drugs
In a Phase I study involving 31 patients with NSCLC, NAMI-A combined with gemcitabine was evaluated. Many patients experienced common adverse effects, including fatigue, nausea, vomiting, and diarrhea. Out of 15 evaluable patients, only 1 experienced partial remission, suggesting that NAMI-A’s efficacy may not surpass that of gemcitabine alone [166]. In 2009, the ruthenium-based drug KP1019 was administered to 7 patients with various solid tumors to assess its pharmacokinetics. No dose-limiting toxicity was observed, likely due to the low solubility of the drug. KP1019 showed low clearance and renal excretion was responsible for eliminating only a small fraction of ruthenium. Biliary excretion was suggested as the primary route of elimination for KP1019. However, the study did not evaluate the drug’s effect on the tumors [167]. A more recent study evaluated the safety and tolerability of the photoactivable ruthenium compound TLD-1433 in 6 patients with non–muscle-invasive bladder cancer (NMIBC). The study showed that TLD-1433 was well tolerated, with no serious adverse events. Three patients were treated with a dose of 0.70 mg/cm2 of TLD-1433. Drug concentrations in the urine were measured at 60.1 ng/mL and 0.6 ng/mL at 24 and 72 h, respectively, and the drug was undetectable after 72 h. Of these three patients, two achieved complete responses at 180 days, although one patient developed metastatic disease at 138 days. Overall, TLD-1433 demonstrated good tolerability and safety, with potential efficacy [168].
Over the past ten years, no clinical trials have been conducted with KP1019, KP1339, or other non-platinum metal-based drugs in cancer patients.
Given the increase in cancer worldwide, it has become necessary to find new drugs capable of inhibiting tumor growth and inducing apoptosis in cancer cells specifically, without affecting normal cells, thereby avoiding undesirable effects on patients. Metal-based drugs therefore appear to have considerable potential as novel therapeutic agents against cancer because they display wide chemical diversity and versatility, exist in different oxidation states, coordinate diverse types of ligands, exhibit redox activity, and react with important biomolecules in cells, such as proteins or DNA. Metallodrugs based on platinum, ruthenium, copper, gold, palladium, zinc, and iridium exhibit different mechanisms of action, target different components, and show varying toxicities, making them a promising avenue for overcoming drug resistance in cancer treatment.
Platinum-based drugs were the first metal-based molecules whose antiproliferative activity was described in the mid-1960s, particularly cisplatin, which was approved by the FDA in the late 1970s. Since then, the development of new drugs has progressed rapidly, with drugs such as carboplatin, oxaliplatin, nedaplatin, lobaplatin, heptaplatin, and miriplatin, now in clinical use in various countries. Unfortunately, other metal-based drugs have not been as successful, with only a few ruthenium compounds—such as NAMI-A, KP1019, KP1339, and TLD1433—reaching clinical studies, but only in Phases I or II. No recent clinical trials have been conducted with other metal-based drugs.
Various copper-based compounds have been tested on colon, breast, prostate, and ovarian cancer cells, demonstrating their ability to induce the activity of pro-apoptotic molecules such as p53, BAX, caspase-8 and -9, while inhibiting the expression of Bcl-2. Moreover, copper compounds have been shown to be more effective than platinum-based molecules in the studies reviewed.
Gold compounds appear to induce apoptosis through different mechanisms, either by binding to proteins involved in cell cycle control or directly interacting with DNA. Interestingly, some gold compounds, unlike other metal-based compounds, do not cause mitochondrial damage, while others are photoreactive, suggesting a light-dependent activation mechanism. These compounds can be activated by UV light, allowing for greater precision in drug efficacy.
This photoactivatable activity has also been observed in palladium and iridium compounds, which were tested on cancer cells, showing greater anticancer activity when irradiated with light. Similar results were observed in palladium compounds, which were tested on tumor xenografts in nude mice, showing higher inhibition of tumor growth when the animals were irradiated with light. Other palladium and iridium compounds have also been evaluated in vivo, showing a significant decrease in tumor weight in treated animals.
Zinc-based drugs have been combined with other drugs, such as paclitaxel and cisplatin, showing significantly higher activity compared to the drugs used alone.
Additionally, interesting studies combining natural compounds with metal-based drugs were reviewed, indicating that these not only improve antitumor activity but also reduce cytotoxicity in normal cells, which could potentially reduce side effects for patients in future applications of metal-based compounds.
The limitation of this review is the lack of clinical studies, so we only analyzed results on some platinum compounds. However, we did not find clinical studies with other metal-based compounds, which restricted the discussion on the topic. We hope that more metal-based complexes, beyond the well-known platinum-based drugs, can advance to clinical trials. The in vitro and in vivo results for these compounds are very promising, but there is a notable lack of pharmacokinetic and pharmacodynamic studies for many of these metallodrugs, which are highlighted in this review.
Advancements in these types of studies could provide new opportunities for millions of cancer patients worldwide.
Acknowledgement: Not applicable.
Funding Statement: The authors received no specific funding for this study.
Author Contributions: Giuliano Bernal: Conceptualization, Writing—original draft, Supervision, Writing—review & editing. Gisela Aquea: Writing—original draft, Visualization, Writing—review & editing. Sebastian Ramírez-Rivera: Writing—original draft, Visualization, Writing–review & editing. All authors reviewed the results and approved the final version of the manuscript.
Availability of Data and Materials: Not applicable.
Ethics Approval: Not applicable.
Conflicts of Interest: The authors declare no conflicts of interest to report regarding the present study.
References
1. Bray F, Laversanne M, Sung H, Ferlay J, Siegel RL, Soerjomataram I, et al. Global cancer statistics 2022: GLOBOCAN estimates of incidence and mortality worldwide for 36 cancers in 185 countries. CA Cancer J Clin. 2024;74(3):229–63. doi:10.3322/caac.v74.3. [Google Scholar] [CrossRef]
2. Ghosh S. Cisplatin: the first metal based anticancer drug. Bioorg Chem. 2019;88:102925. doi:10.1016/j.bioorg.2019.102925. [Google Scholar] [PubMed] [CrossRef]
3. Riccardi C, Piccolo M. Metal-based complexes in cancer. Int J Mol Sci. 2023;24(8):7289. doi:10.3390/ijms24087289. [Google Scholar] [PubMed] [CrossRef]
4. Romani AMP. Cisplatin in cancer treatment. Biochem Pharmacol. 2022;206:115323. doi:10.1016/j.bcp.2022.115323. [Google Scholar] [PubMed] [CrossRef]
5. Beirne DF, Farkaš B, Donati C, Gandin V, Rozas I, Velasco-Torrijos T, et al. Novel design of dual-action Pt (IV) anticancer pro-drugs based on cisplatin and derivatives of the tyrosine kinase inhibitors imatinib and nilotinib. Dalton Trans. 2023;52(39):14110–22. doi:10.1039/D3DT02030D. [Google Scholar] [PubMed] [CrossRef]
6. Pernar Kovač M, Tadić V, Kralj J, Duran GE, Stefanelli A, Stupin Polančec D, et al. Carboplatin-induced upregulation of pan β-tubulin and class III β-tubulin is implicated in acquired resistance and cross-resistance of ovarian cancer. Cell Mol Life Sci. 2023;80(10):294. doi:10.1007/s00018-023-04943-0. [Google Scholar] [PubMed] [CrossRef]
7. Sanchon-Sanchez P, Briz O, Macias RIR, Abad M, Sanchez-Martin A, Marin JJG, et al. Evaluation of potential targets to enhance the sensitivity of cholangiocarcinoma cells to anticancer drugs. Biomed Pharmacother. 2023;168:115658. doi:10.1016/j.biopha.2023.115658. [Google Scholar] [PubMed] [CrossRef]
8. Tsang ES, Aggarwal RR, Dhawan MS, Bergsland EK, Alvarez EA, Calabrese S, et al. A phase IB trial of the PI3K inhibitor Alpelisib and weekly cisplatin in patients with solid tumor malignancies. Cancer Res Commun. 2022;2(7):570–6. doi:10.1158/2767-9764.CRC-22-0028. [Google Scholar] [PubMed] [CrossRef]
9. Thaklaewphan P, Wikan N, Potikanond S, Nimlamool W. Oxyresveratrol enhances the anti-cancer effect of cisplatin against epithelial ovarian cancer cells through suppressing the activation of protein Kinase B (AKT). Biomolecules. 2024;14(9):1140. doi:10.3390/biom14091140. [Google Scholar] [PubMed] [CrossRef]
10. Chen RL, Wang Z, Huang P, Sun CH, Yu WY, Zhang HH, et al. Isovitexin potentiated the antitumor activity of cisplatin by inhibiting the glucose metabolism of lung cancer cells and reduced cisplatin-induced immunotoxicity in mice. Int Immunopharmacol. 2021;94:107357. doi:10.1016/j.intimp.2020.107357. [Google Scholar] [PubMed] [CrossRef]
11. Motie FM, Soltani Howyzeh M, Ghanbariasad A. Synergic effects of DL-limonene, R-limonene, and cisplatin on AKT, PI3K, and mTOR gene expression in MDA-MB-231 and 5637 cell lines. Int J Biol Macromol. 2024;280:136216. doi:10.1016/j.ijbiomac.2024.136216. [Google Scholar] [PubMed] [CrossRef]
12. Lee KC, Wu KL, Chang SF, Chang HI, Chen CN, Chen YY. Fermented ginger extract in natural deep eutectic solvent enhances cytotoxicity by inhibiting NF-κB mediated CXC chemokine receptor 4 expression in oxaliplatin-resistant human colorectal cancer cells. Antioxid. 2022;11(10):2057. doi:10.3390/antiox11102057. [Google Scholar] [PubMed] [CrossRef]
13. Mbemi AT, Sims JN, Yedjou CG, Noubissi FK, Gomez CR, Tchounwou PB. Vernonia calvoana shows promise towards the treatment of ovarian cancer. Int J Mol Sci. 2020;21(12):4429. doi:10.3390/ijms21124429. [Google Scholar] [PubMed] [CrossRef]
14. Li Y, Wu Y, Xia Q, Zhao Y, Zhao R, Deng S. Platycodon grandiflorus enhances the effect of DDP against lung cancer by down regulating PI3K/Akt signaling pathway. Biomed Pharmacother. 2019;120:109496. doi:10.1016/j.biopha.2019.109496. [Google Scholar] [PubMed] [CrossRef]
15. Wen L, Hu J, Zhang J, Yang J. Phenylethanol glycosides from Herba Cistanche improve the hypoxic tumor microenvironment and enhance the effects of oxaliplatin via the HIF-1α signaling pathway. Mol Med Rep. 2021;24(1):517. doi:10.3892/mmr.2021.12156. [Google Scholar] [PubMed] [CrossRef]
16. Sedky NK, Abdel-Kader NM, Issa MY, Abdelhady MMM, Shamma SN, Bakowsky U, et al. Co-delivery of Ylang Ylang oil of cananga odorata and oxaliplatin using intelligent PH-sensitive lipid-based nanovesicles for the effective treatment of triple-negative breast cancer. Int J Mol Sci. 2023;24(9):8392. doi:10.3390/ijms24098392. [Google Scholar] [PubMed] [CrossRef]
17. Wang C, Chen C, Chen X, Luo J, Su Y, Liu X, et al. Identification of genes predicting chemoresistance and short survival in ovarian cancer. Transl Cancer Res. 2024;13(8):4354–71. doi:10.21037/tcr. [Google Scholar] [CrossRef]
18. Lucaciu RL, Hangan AC, Sevastre B, Oprean LS. Metallo-drugs in cancer therapy: past, present and future. Molecules. 2022;27(19):6485. doi:10.3390/molecules27196485. [Google Scholar] [PubMed] [CrossRef]
19. Czarnomysy R, Radomska D, Szewczyk OK, Roszczenko P, Bielawski K. Platinum and palladium complexes as promising sources for antitumor treatments. Int J Mol Sci. 2021;22(15):8271. doi:10.3390/ijms22158271. [Google Scholar] [PubMed] [CrossRef]
20. Kacsir I, Sipos A, Bényei A, Janka E, Buglyó P, Somsák L, et al. Reactive oxygen species production is responsible for antineoplastic activity of osmium, ruthenium, iridium and rhodium half-sandwich type complexes with bidentate glycosyl heterocyclic ligands in various cancer cell models. Int J Mol Sci. 2022;23(2):813. doi:10.3390/ijms23020813. [Google Scholar] [PubMed] [CrossRef]
21. Geisler H, Harringer S, Wenisch D, Urban R, Jakupec MA, Kandioller W, et al. Systematic study on the cytotoxic potency of commonly used dimeric metal precursors in human cancer cell lines. ChemistryOpen. 2022;11(7):e202200019. doi:10.1002/open.202200019. [Google Scholar] [PubMed] [CrossRef]
22. Łomzik M, Błauż A, Tchoń D, Makal A, Rychlik B, Plażuk D. Development of half-sandwich Ru, Os, Rh, and Ir complexes bearing the Pyridine-2-ylmethanimine bidentate ligand derived from 7-Chloroquinazolin-4(3H)-one with enhanced antiproliferative activity. ACS Omega. 2024;9(16):18224–37. doi:10.1021/acsomega.3c10482. [Google Scholar] [PubMed] [CrossRef]
23. Selim MS, Kassem AB, El-Bassiouny NA, Salahuddin A, Abu El-Ela RY, Hamza MS. Polymorphic renal transporters and cisplatin’s toxicity in urinary bladder cancer patients: current perspectives and future directions. Med Oncol. 2023;40(2):80. doi:10.1007/s12032-022-01928-0. [Google Scholar] [PubMed] [CrossRef]
24. Ciarimboli G. Membrane transporters as mediators of Cisplatin and side effects. Anticancer Res. 2014;34(1):547–50. [Google Scholar] [PubMed]
25. Tan WJT, Vlajkovic SM. Molecular characteristics of cisplatin-induced ototoxicity and therapeutic interventions. Int J Mol Sci. 2023;24(22):16545. doi:10.3390/ijms242216545. [Google Scholar] [PubMed] [CrossRef]
26. Lukanović D, Herzog M, Kobal B, Černe K. The contribution of copper efflux transporters ATP7A and ATP7B to chemoresistance and personalized medicine in ovarian cancer. Biomed Pharmacother. 2020;129:110401. doi:10.1016/j.biopha.2020.110401. [Google Scholar] [PubMed] [CrossRef]
27. Arnesano F, Natile G. Interference between copper transport systems and platinum drugs. Semin Cancer Biol. 2021;76:173–88. doi:10.1016/j.semcancer.2021.05.023. [Google Scholar] [PubMed] [CrossRef]
28. Jeon J, Lee S, Kim H, Kang H, Youn H, Jo S, et al. Revisiting platinum-based anticancer drugs to overcome gliomas. Int J Mol Sci. 2021;22(10):5111. doi:10.3390/ijms22105111. [Google Scholar] [PubMed] [CrossRef]
29. Zhang C, Xu C, Gao X, Yao Q. Platinum-based drugs for cancer therapy and anti-tumor strategies. Theranostics. 2022;12(5):2115–32. doi:10.7150/thno.69424. [Google Scholar] [PubMed] [CrossRef]
30. Dasari S, Njiki S, Mbemi A, Yedjou CG, Tchounwou PB. Pharmacological effects of cisplatin combination with natural products in cancer chemotherapy. Int J Mol Sci. 2022;23(3):1532. doi:10.3390/ijms23031532. [Google Scholar] [PubMed] [CrossRef]
31. Minerva, Bhat A, Verma S, Chander G, Jamwal RS, Sharma B, et al. Cisplatin-based combination therapy for cancer. J Cancer Res Ther. 2023;19(3):530–6. doi:10.4103/jcrt.jcrt_792_22. [Google Scholar] [PubMed] [CrossRef]
32. Fang CY, Lou DY, Zhou LQ, Wang JC, Yang B, He QJ, et al. Natural products: potential treatments for cisplatin-induced nephrotoxicity. Acta Pharmacol Sin. 2021;42(12):1951–69. doi:10.1038/s41401-021-00620-9. [Google Scholar] [PubMed] [CrossRef]
33. Bork T, Hernando-Erhard C, Liang W, Tian Z, Yamahara K, Huber TB. Cisplatin nephrotoxicity is critically mediated by the availability of BECLIN1. Int J Mol Sci. 2024;25(5):2560. doi:10.3390/ijms25052560. [Google Scholar] [PubMed] [CrossRef]
34. Schofield J, Harcus M, Pizer B, Jorgensen A, McWilliam S. Long-term cisplatin nephrotoxicity after childhood cancer: a systematic review and meta-analysis. Pediatr Nephrol. 2024;39(3):699–710. doi:10.1007/s00467-023-06149-9. [Google Scholar] [PubMed] [CrossRef]
35. McMahon KR, Lebel A, Rassekh SR, Schultz KR, Blydt-Hansen TD, Cuvelier GDE, et al. Acute kidney injury during cisplatin therapy and associations with kidney outcomes 2 to 6 months post-cisplatin in children: a multi-centre, prospective observational study. Pediatr Nephrol. 2023;38(5):1667–85. doi:10.1007/s00467-022-05745-5. [Google Scholar] [PubMed] [CrossRef]
36. El-Gohary RM, Ghalwash AA, Awad MM, El-Shaer RAA, Ibrahim S, Eltantawy AF, et al. Novel insights into the augmented effect of curcumin and liraglutide in ameliorating cisplatin-induced nephrotoxicity in rats: effects on oxidative stress, inflammation, apoptosis and pyroptosis via GSK-3β. Arch Biochem Biophys. 2023;749:109801. doi:10.1016/j.abb.2023.109801. [Google Scholar] [PubMed] [CrossRef]
37. Qiu CW, Chen B, Zhu HF, Liang YL, Mao LS. Gastrodin alleviates cisplatin nephrotoxicity by inhibiting ferroptosis via the SIRT1/FOXO3A/GPX4 signaling pathway. J Ethnopharmacol. 2024;319:117282. doi:10.1016/j.jep.2023.117282. [Google Scholar] [PubMed] [CrossRef]
38. Luo X, Xie D, Chen Z, Ji Q. Protective effects of ginsenosides in cisplatin-induced kidney injury: a systematic review, meta-analysis. Indian J Pharmacol. 2023;55(4):243–50. doi:10.4103/ijp.ijp_251_23. [Google Scholar] [PubMed] [CrossRef]
39. Chen XC, Huang LF, Tang JX, Wu D, An N, Ye ZN, et al. Asiatic acid alleviates cisplatin-induced renal fibrosis in tumor-bearing mice by improving the TFEB-mediated autophagy-lysosome pathway. Biomed Pharmacother. 2023;165:115122. doi:10.1016/j.biopha.2023.115122. [Google Scholar] [PubMed] [CrossRef]
40. Alherz FA, Elekhnawy E, Selim HM, El-Masry TA, El-Kadem AH, Hussein IA, et al. Protective role of betulinic acid against cisplatin-induced nephrotoxicity and its antibacterial potential toward uropathogenic bacteria. Pharmaceuticals. 2023;16(8):1180. doi:10.3390/ph16081180. [Google Scholar] [PubMed] [CrossRef]
41. Lee B, Kim YY, Jeong S, Lee SW, Lee SJ, Rho MC, et al. Oleanolic acid acetate alleviates cisplatin-induced nephrotoxicity via inhibition of apoptosis and necroptosis in vitro and in vivo. Toxics. 2024;12(4):301. doi:10.3390/toxics12040301. [Google Scholar] [PubMed] [CrossRef]
42. Wang L, Xie Y, Xiao B, He X, Ying G, Zha H, et al. Isorhamnetin alleviates cisplatin-induced acute kidney injury via enhancing fatty acid oxidation. Free Radic Biol Med. 2024;212:22–33. doi:10.1016/j.freeradbiomed.2023.12.010. [Google Scholar] [PubMed] [CrossRef]
43. Shao YF, Tang BB, Ding YH, Fang CY, Hong L, Shao CX, et al. Kaempferide ameliorates cisplatin-induced nephrotoxicity via inhibiting oxidative stress and inducing autophagy. Acta Pharmacol Sin. 2023;44(7):1442–54. doi:10.1038/s41401-023-01051-4. [Google Scholar] [PubMed] [CrossRef]
44. Gómez-Sierra T, Ortega-Lozano AJ, Rojas-Morales P, Medina-Reyes EI, Barrera-Oviedo D, Pedraza-Chaverri J. Isoliquiritigenin pretreatment regulates ER stress and attenuates cisplatin-induced nephrotoxicity in male Wistar rats. J Biochem Mol Toxicol. 2023;37(12):e23492. doi:10.1002/jbt.v37.12. [Google Scholar] [CrossRef]
45. Lin SY, Chang CL, Liou KT, Kao YK, Wang YH, Chang CC, et al. The protective role of Achyranthes aspera extract against cisplatin-induced nephrotoxicity by alleviating oxidative stress, inflammation, and PANoptosis. J Ethnopharmacol. 2024;319:117097. doi:10.1016/j.jep.2023.117097. [Google Scholar] [PubMed] [CrossRef]
46. Ibrahim D, Halboup A, Al Ashwal M, Shamsher A. Ameliorative effect of olea europaea leaf extract on cisplatin-induced nephrotoxicity in the rat model. Int J Nephrol. 2023;2023:2074498. doi:10.1155/2023/2074498. [Google Scholar] [PubMed] [CrossRef]
47. Mahmod II, Ismail IS, Normi YM, Chong SG. Protective effect of Clinacanthus nutans in cisplatin-induced nephrotoxicity on human kidney cell (PCS-400-010) elucidated by an LCMS-based metabolomics approach. Biomed Chromatogr. 2023;37(12):e5750. doi:10.1002/bmc.5750. [Google Scholar] [PubMed] [CrossRef]
48. Turan I, Canbolat D, Demir S, Kerimoglu G, Colak F, Turkmen Alemdar N, et al. The ameliorative effect of Primula vulgaris on cisplatin-induced nephrotoxicity in rats and quantification of its phenolic components using LC-ESI-MS/MS. Saudi Pharm J. 2023;31(9):101730. doi:10.1016/j.jsps.2023.101730. [Google Scholar] [PubMed] [CrossRef]
49. Qi H, Shi H, Yan M, Zhao L, Yin Y, Tan X, et al. Ammonium tetrathiomolybdate relieves oxidative stress in cisplatin-induced acute kidney injury via NRF2 signaling pathway. Cell Death Discov. 2023;9(1):259. doi:10.1038/s41420-023-01564-1. [Google Scholar] [PubMed] [CrossRef]
50. Tian R, Tang S, Zhao J, Hao Y, Zhao L, Han X, et al. β-hydroxybutyrate protects against cisplatin-induced renal damage via regulating ferroptosis. Ren Fail. 2024;46(1):2354918. doi:10.1080/0886022X.2024.2354918. [Google Scholar] [PubMed] [CrossRef]
51. Jiang X, Zhu X, Liu Y, Zhou N, Zhao Z, Lv H. Diallyl trisulfide and its active metabolite allyl methyl sulfone attenuate cisplatin-induced nephrotoxicity by inhibiting the ROS/MAPK/NF-κB pathway. Int Immunopharmacol. 2024;127:111373. doi:10.1016/j.intimp.2023.111373. [Google Scholar] [PubMed] [CrossRef]
52. Luo Y, Zhang J, Jiao Y, Huang H, Ming L, Song Y, et al. Dihydroartemisinin abolishes cisplatin-induced nephrotoxicity in vivo. J Nat Med. 2024;78(2):439–54. doi:10.1007/s11418-024-01783-5. [Google Scholar] [PubMed] [CrossRef]
53. Abd Alhusen SK, Hasan AF. Evaluating the renoprotective effects of omega-3-6-9 against cisplatin-induced nephrotoxicity in mice. J Med Life. 2023;16(12):1756–9. doi:10.25122/jml-2023-0078. [Google Scholar] [PubMed] [CrossRef]
54. El-Dessouki AM, Alzokaky AA, Raslan NA, Ibrahim S, Salama LA, Yousef EH. Piracetam mitigates nephrotoxicity induced by cisplatin via the AMPK-mediated PI3K/Akt and MAPK/JNK/ERK signaling pathways. Int Immunopharmacol. 2024;137:112511. doi:10.1016/j.intimp.2024.112511. [Google Scholar] [PubMed] [CrossRef]
55. Senguttuvan RN, Santiago NL, Han ES, Lee B, Lee S, Lin WC, et al. Impact of sodium thiosulfate on prevention of nephrotoxicities in HIPEC: an ancillary evaluation of cisplatin-induced toxicities in ovarian cancer. Ann Surg Oncol. 2023;30(13):8144–55. doi:10.1245/s10434-023-14216-6. [Google Scholar] [PubMed] [CrossRef]
56. Yamamoto H, Ishida Y, Zhang S, Osako M, Nosaka M, Kuninaka Y, et al. Protective roles of thrombomodulin in cisplatin-induced nephrotoxicity through the inhibition of oxidative and endoplasmic reticulum stress. Sci Rep. 2024;14(1):14004. doi:10.1038/s41598-024-64619-y. [Google Scholar] [PubMed] [CrossRef]
57. Wang X, Zhou Y, Wang D, Wang Y, Zhou Z, Ma X, et al. Cisplatin-induced ototoxicity: from signaling network to therapeutic targets. Biomed Pharmacother. 2023;157:114045. doi:10.1016/j.biopha.2022.114045. [Google Scholar] [PubMed] [CrossRef]
58. Meijer AJM, Li KH, Brooks B, Clemens E, Ross CJ, Rassekh SR, et al. The cumulative incidence of cisplatin-induced hearing loss in young children is higher and develops at an early stage during therapy compared with older children based on 2052 audiological assessments. Cancer. 2022;128(1):169–79. doi:10.1002/cncr.v128.1. [Google Scholar] [CrossRef]
59. McAdam AD, Batchelor LK, Romano-deGea J, Vasilyev D, Dyson PJ. Thermoresponsive carboplatin-releasing prodrugs. J Inorg Biochem. 2024;254:112505. doi:10.1016/j.jinorgbio.2024.112505. [Google Scholar] [PubMed] [CrossRef]
60. Forgie BN, Prakash R, Telleria CM. Revisiting the anti-cancer toxicity of clinically approved platinating derivatives. Int J Mol Sci. 2022;23(23):15410. doi:10.3390/ijms232315410. [Google Scholar] [PubMed] [CrossRef]
61. Azab B, Alassaf A, Abu-Humdan A, Dardas Z, Almousa H, Alsalem M, et al. Genotoxicity of cisplatin and carboplatin in cultured human lymphocytes: a comparative study. Interdiscip Toxicol. 2019;12(2):93–7. doi:10.2478/intox-2019-0011. [Google Scholar] [PubMed] [CrossRef]
62. Mason SR, Willson ML, Egger SJ, Beith J, Dear RF, Goodwin A. Platinum chemotherapy for early triple-negative breast cancer. Breast. 2024;75:103712. doi:10.1016/j.breast.2024.103712. [Google Scholar] [PubMed] [CrossRef]
63. Villacampa G, Matikas A, Oliveira M, Prat A, Pascual T, Papakonstantinou A. Landscape of neoadjuvant therapy in HER2-positive breast cancer: a systematic review and network meta-analysis. Eur J Cancer. 2023;190:112885. doi:10.1016/j.ejca.2023.03.042. [Google Scholar] [PubMed] [CrossRef]
64. Mori K, Schuettfort VM, Yanagisawa T, Katayama S, Pradere B, Laukhtina E, et al. Reassessment of the efficacy of carboplatin for metastatic urothelial carcinoma in the era of immunotherapy: a systematic review and meta-analysis. Eur Urol Focus. 2022;8(6):1687–95. doi:10.1016/j.euf.2022.02.007. [Google Scholar] [PubMed] [CrossRef]
65. Van der Gaag S, Labots M, Swart EL, Crul M. Reducing renal function assessment prior to platinum-based chemotherapy: a real-world evaluation. Acta Oncol. 2024;63:169–74. doi:10.2340/1651-226X.2024.23960. [Google Scholar] [PubMed] [CrossRef]
66. Fernandes ARDS, de Brito GA, Baptista AL, Andrade LAS, Imanishe MH, Pereira BJ. The influence of acute kidney disease on the clinical outcomes of patients who received cisplatin, carboplatin, and oxaliplatin. Health Sci Rep. 2022;5(1):e479. doi:10.1002/hsr2.479. [Google Scholar] [PubMed] [CrossRef]
67. Choudhary R, Bundela MK, Bharang K. Comparison of renal functions evaluated by measured glomerular filtration rate in patients treated with cisplatin, carboplatin, and oxaliplatin. Cureus. 2023;15(3):e36549. doi:10.7759/cureus.36549. [Google Scholar] [PubMed] [CrossRef]
68. Nechay M, Wang D, Kleiner RE. Inhibition of nucleolar transcription by oxaliplatin involves ATM/ATR kinase signaling. Cell Chem Biol. 2023;30(8):906–919.e4. doi:10.1016/j.chembiol.2023.06.010. [Google Scholar] [PubMed] [CrossRef]
69. Cheng F, Zhang R, Sun C, Ran Q, Zhang C, Shen C, et al. Oxaliplatin-induced peripheral neurotoxicity in colorectal cancer patients: mechanisms, pharmacokinetics and strategies. Front Pharmacol. 2023;14:1231401. doi:10.3389/fphar.2023.1231401. [Google Scholar] [PubMed] [CrossRef]
70. Goldberg RM, Sargent DJ, Morton RF, Fuchs CS, Ramanathan RK, Williamson SK, et al. A randomized controlled trial of fluorouracil plus leucovorin, irinotecan, and oxaliplatin combinations in patients with previously untreated metastatic colorectal cancer. J Clin Oncol. 2023;41(19):3461–8. doi:10.1200/JCO.22.02759. [Google Scholar] [PubMed] [CrossRef]
71. Kawai S, Takeshima N, Hayasaka Y, Notsu A, Yamazaki M, Kawabata T, et al. Comparison of irinotecan and oxaliplatin as the first-line therapies for metastatic colorectal cancer: a meta-analysis. BMC Cancer. 2021;21(1):116. doi:10.1186/s12885-021-07823-7. [Google Scholar] [PubMed] [CrossRef]
72. Peipert JD, Roydhouse J, Tighiouart M, Henry NL, Kim S, Hays RD, et al. Overall side effect assessment of oxaliplatin toxicity in rectal cancer patients in NRG oncology/NSABP R04. Qual Life Res. 2024;33(11):3069–79. doi:10.1007/s11136-024-03746-5. [Google Scholar] [PubMed] [CrossRef]
73. Aboeita NM, Fahmy SA, El-Sayed MMH, Azzazy HME, Shoeib T. Enhanced anticancer activity of nedaplatin loaded onto copper nanoparticles synthesized using red algae. Pharmaceutics. 2022;14(2):418. doi:10.3390/pharmaceutics14020418. [Google Scholar] [PubMed] [CrossRef]
74. Chikazawa K, Netsu S, Imai K, Ishiguro A, Kimura A, Wang L, et al. Nedaplatin use in patients with hypersensitivity reaction episodes to carboplatin. Taiwan J Obstet Gynecol. 2020;59(4):546–50. doi:10.1016/j.tjog.2020.05.013. [Google Scholar] [PubMed] [CrossRef]
75. Yu Q, Lan T, Ma Z, Wang Z, Zhang C, Jiang Y, et al. Lobaplatin induces apoptosis in T24 and 5637 bladder cancer cells by regulating Bcl-2 and Bax expression and inhibiting the PI3K/Akt signaling pathway. Transl Androl Urol. 2023;12(8):1296–307. doi:10.21037/tau-23-376. [Google Scholar] [PubMed] [CrossRef]
76. Tsvetkova D, Ivanova S. Application of approved cisplatin derivatives in combination therapy against different cancer diseases. Molecules. 2022;27(8):2466. doi:10.3390/molecules27082466. [Google Scholar] [PubMed] [CrossRef]
77. Huang X, Zhou H, Jiao R, Liu H, Qin C, Xu L, et al. Supramolecular chemotherapy: host-guest complexes of Heptaplatin-Cucurbit[7]uril toward colorectal normal and tumor cells. Langmuir. 2021;37(18):5475–82. doi:10.1021/acs.langmuir.0c03603. [Google Scholar] [PubMed] [CrossRef]
78. Wang X, Wang M, Cai M, Shao R, Xia G, Zhao W. Miriplatin-loaded liposome, as a novel mitophagy inducer, suppresses pancreatic cancer proliferation through blocking POLG and TFAM-mediated mtDNA replication. Acta Pharm Sin B. 2023;13(11):4477–501. doi:10.1016/j.apsb.2023.07.009. [Google Scholar] [PubMed] [CrossRef]
79. Zhang C, Kang T, Wang X, Song J, Zhang J, Li G. Stimuli-responsive platinum and ruthenium complexes for lung cancer therapy. Front Pharmacol. 2022;13:1035217. doi:10.3389/fphar.2022.1035217. [Google Scholar] [PubMed] [CrossRef]
80. Lin K, Zhao ZZ, Bo HB, Hao XJ, Wang JQ. Applications of ruthenium complex in tumor diagnosis and therapy. Front Pharmacol. 2018;9:1323. doi:10.3389/fphar.2018.01323. [Google Scholar] [PubMed] [CrossRef]
81. Riisom M, Eade L, Tremlett WDJ, Hartinger CG. The aqueous stability and interactions of organoruthenium compounds with serum proteins, cell culture medium, and human serum. Metallomics. 2022;14(7):mfac043. doi:10.1093/mtomcs/mfac043. [Google Scholar] [PubMed] [CrossRef]
82. Deng Z, Gao P, Yu L, Ma B, You Y, Chan L, et al. Ruthenium complexes with phenylterpyridine derivatives target cell membrane and trigger death receptors-mediated apoptosis in cancer cells. Biomaterials. 2017;129:111–26. doi:10.1016/j.biomaterials.2017.03.017. [Google Scholar] [PubMed] [CrossRef]
83. Levina A, Chetcuti ARM, Lay PA. Controversial role of transferrin in the transport of ruthenium anticancer drugs. Biomolecules. 2022;12(9):1319. doi:10.3390/biom12091319. [Google Scholar] [PubMed] [CrossRef]
84. Kanaoujiya R, Meenakshi, Srivastava S, Singh R, Mustafa G. Recent advances and application of ruthenium complexes in tumor malignancy. Mat Today: Proc. 2023;72(6):2822–7. [Google Scholar]
85. Tang H, Guo X, Yu W, Gao J, Zhu X, Huang Z, et al. Ruthenium(II) complexes as mitochondrial inhibitors of topoisomerase induced A549 cell apoptosis. J Inorg Biochem. 2023;246:112295. doi:10.1016/j.jinorgbio.2023.112295. [Google Scholar] [PubMed] [CrossRef]
86. Althobaiti F, Sahyon HA, Shanab MMAH, Aldhahrani A, Helal MA, Khireldin A, et al. A comparative study of novel ruthenium(III) and iron(III) complexes containing uracil; docking and biological studies. J Inorg Biochem. 2023;247:112308. doi:10.1016/j.jinorgbio.2023.112308. [Google Scholar] [PubMed] [CrossRef]
87. Maikoo S, Xulu B, Mambanda A, Mkhwanazi N, Davison C, de la Mare JA, et al. Biomolecular interactions of cytotoxic ruthenium compounds with thiosemicarbazone or benzothiazole schiff base chelates. ChemMedChem. 2022;17(20):e202200444. doi:10.1002/cmdc.202200444. [Google Scholar] [PubMed] [CrossRef]
88. Ceramella J, Troiano R, Iacopetta D, Mariconda A, Pellegrino M, Catalano A, et al. Synthesis of novel N-heterocyclic carbene-ruthenium (II) complexes, “precious” tools with antibacterial, anticancer and antioxidant properties. Antibiotics. 2023;12(4):693. doi:10.3390/antibiotics12040693. [Google Scholar] [PubMed] [CrossRef]
89. Legina MS, Nogueira JJ, Kandioller W, Jakupec MA, González L, Keppler BK. Biological evaluation of novel thiomaltol-based organometallic complexes as topoisomerase IIα inhibitors. J Biol Inorg Chem. 2020;25(3):451–65. doi:10.1007/s00775-020-01775-2. [Google Scholar] [PubMed] [CrossRef]
90. Ling YY, Li ZY, Mu X, Kong YJ, Hao L, Wang WJ, et al. Self-assembly of a ruthenium-based cGAS-STING photoactivator for carrier-free cancer immunotherapy. Eur J Med Chem. 2024;275:116638. doi:10.1016/j.ejmech.2024.116638. [Google Scholar] [PubMed] [CrossRef]
91. Del Pino JMV, Scalambra F, Bermejo-Casadesús C, Massaguer A, García-Maroto F, Romerosa A. Study of the biological activity of photoactive bipyridyl-Ru(II) complexes containing 1,3,5-triaza-7-phosphaadamantane (PTA). J Inorg Biochem. 2023;246:112291. doi:10.1016/j.jinorgbio.2023.112291. [Google Scholar] [PubMed] [CrossRef]
92. Ballester FJ, Hernández-García A, Santana MD, Bautista D, Ashoo P, Ortega-Forte E, et al. Photoactivatable ruthenium complexes containing minimal straining Benzothiazolyl-1,2,3-triazole chelators for cancer treatment. Inorg Chem. 2024;63(14):6202–16. doi:10.1021/acs.inorgchem.3c04432. [Google Scholar] [PubMed] [CrossRef]
93. Gandioso A, Izquierdo-García E, Mesdom P, Arnoux P, Demeubayeva N, Burckel P, et al. Ru(II)-cyanine complexes as promising photodynamic photosensitizers for the treatment of hypoxic tumours with highly penetrating 770 nm near-infrared light. Chemistry. 2023;29(61):e202301742. doi:10.1002/chem.202301742. [Google Scholar] [PubMed] [CrossRef]
94. Santos LS, Silva VR, de Castro MVL, Dias RB, Valverde LF, Rocha CAG, et al. New ruthenium-xanthoxylin complex eliminates colorectal cancer stem cells by targeting the heat shock protein 90 chaperone. Cell Death Dis. 2023;14(12):832. doi:10.1038/s41419-023-06330-w. [Google Scholar] [PubMed] [CrossRef]
95. Silva VR, Santos LS, de Castro MVL, Dias RB, Valverde LF, Rocha CAG, et al. A novel ruthenium complex with 5-fluorouracil suppresses colorectal cancer stem cells by inhibiting Akt/mTOR signaling. Cell Death Discov. 2023;9(1):460. doi:10.1038/s41420-023-01759-6. [Google Scholar] [PubMed] [CrossRef]
96. Wang ZF, Huang XQ, Wu RC, Xiao Y, Zhang SH. Antitumor studies evaluation of triphenylphosphine ruthenium complexes with 5,7-dihalo-substituted-8-quinolinoline targeting mitophagy pathways. J Inorg Biochem. 2023;248:112361. doi:10.1016/j.jinorgbio.2023.112361. [Google Scholar] [PubMed] [CrossRef]
97. Ramírez-Rivera S, Pizarro S, Gallardo M, Gajardo F, Delgadillo A, De La Fuente-Ortega E, et al. Anticancer activity of two novel ruthenium compounds in gastric cancer cells. Life Sci. 2018;213:57–65. doi:10.1016/j.lfs.2018.10.024. [Google Scholar] [PubMed] [CrossRef]
98. Carrillo E, Ramírez-Rivera S, Bernal G, Aquea G, Tessini C, Thomet FA. Water-soluble Ru(II)-anethole compounds with promising cytotoxicity toward the human gastric cancer cell line AGS. Life Sci. 2019;217:193–201. doi:10.1016/j.lfs.2018.12.010. [Google Scholar] [PubMed] [CrossRef]
99. Liu T, Pan C, Shi H, Huang T, Huang YL, Deng YY, et al. Cytotoxic cis-ruthenium(III) bis(amidine) complexes. Dalton Trans. 2023;52(25):8540–8. doi:10.1039/D3DT00328K. [Google Scholar] [PubMed] [CrossRef]
100. Cao M, Fan B, Zhen T, Das A, Wang J. Ruthenium biochanin—a complex ameliorates lung carcinoma through the downregulation of the TGF-β/PPARγ/PI3K/TNF-α pathway in association with caspase-3-mediated apoptosis. Toxicol Res. 2023;39(3):455–75. doi:10.1007/s43188-023-00177-1. [Google Scholar] [PubMed] [CrossRef]
101. Michlewska S, Wójkowska D, Watala C, Skiba E, Ortega P, de la Mata FJ, et al. Ruthenium metallodendrimer against triple-negative breast cancer in mice. Nanomed. 2023;53:102703. doi:10.1016/j.nano.2023.102703. [Google Scholar] [PubMed] [CrossRef]
102. Happl B, Brandt M, Balber T, Benčurová K, Talip Z, Voegele A, et al. Synthesis and preclinical evaluation of radiolabeled [103Ru] BOLD-100. Pharmaceutics. 2023;15(11):2626. doi:10.3390/pharmaceutics15112626. [Google Scholar] [PubMed] [CrossRef]
103. Lu Y, Zhu D, Le Q, Wang Y, Wang W. Ruthenium-based antitumor drugs and delivery systems from monotherapy to combination therapy. Nanoscale. 2022;14(44):16339–75. doi:10.1039/D2NR02994D. [Google Scholar] [PubMed] [CrossRef]
104. Machado PHA, Paixão DA, Lino RC, de Souza TR, de Souza Bontempo NJ, Sousa LM, et al. A selective CuII complex with 4-fluorophenoxyacetic acid hydrazide and phenanthroline displays DNA-cleaving and pro-apoptotic properties in cancer cells. Sci Rep. 2021;11(1):24450. doi:10.1038/s41598-021-03909-1. [Google Scholar] [PubMed] [CrossRef]
105. Ji P, Wang P, Chen H, Xu Y, Ge J, Tian Z, et al. Potential of copper and copper compounds for anticancer applications. Pharmaceuticals. 2023;16(2):234. doi:10.3390/ph16020234. [Google Scholar] [PubMed] [CrossRef]
106. Lelièvre P, Sancey L, Coll JL, Deniaud A, Busser B. The multifaceted roles of copper in cancer: a trace metal element with dysregulated metabolism, but also a target or a bullet for therapy. Cancers. 2020;12(12):3594. doi:10.3390/cancers12123594. [Google Scholar] [PubMed] [CrossRef]
107. Khan AQ, Rashid K, AlAmodi AA, Agha MV, Akhtar S, Hakeem I, et al. Reactive oxygen species (ROS) in cancer pathogenesis and therapy: an update on the role of ROS in anticancer action of benzophenanthridine alkaloids. Biomed Pharmacother. 2021;143:112142. doi:10.1016/j.biopha.2021.112142. [Google Scholar] [PubMed] [CrossRef]
108. Nakamura H, Takada K. Reactive oxygen species in cancer: current findings and future directions. Cancer Sci. 2021;112(10):3945–52. doi:10.1111/cas.v112.10. [Google Scholar] [CrossRef]
109. Gao W, Huang Z, Duan J, Nice EC, Lin J, Huang C. Elesclomol induces copper-dependent ferroptosis in colorectal cancer cells via degradation of ATP7A. Mol Oncol. 2021;15(12):3527–44. doi:10.1002/mol2.v15.12. [Google Scholar] [CrossRef]
110. Ghasemi P, Shafiee G, Ziamajidi N, Abbasalipourkabir R. Copper nanoparticles induce apoptosis and oxidative stress in SW480 human colon cancer cell line. Biol Trace Elem Res. 2023;201(8):3746–54. doi:10.1007/s12011-022-03458-2. [Google Scholar] [PubMed] [CrossRef]
111. Al-Zharani M, Qurtam AA, Daoush WM, Eisa MH, Aljarba NH, Alkahtani S, et al. Antitumor effect of copper nanoparticles on human breast and colon malignancies. Environ Sci Pollut Res. 2021;28(2):1587–95. doi:10.1007/s11356-020-09843-5. [Google Scholar] [PubMed] [CrossRef]
112. Masuri S, Vaňhara P, Cabiddu MG, Moráň L, Havel J, Cadoni E, et al. Copper(II) phenanthroline-based complexes as potential anticancer drugs: a walkthrough on the mechanisms of action. Molecules. 2022;27(1):49. [Google Scholar]
113. Xi YH, Yan X, Bigdeli F, Zhang Q, Esrafili L, Hanifehpour Y, et al. Two new Cu (II) complexes based on 5-fluorouracil-1-yl acetic acid and N-donor ligands: investigation of their interaction with DNA and anticancer activity. Appl Organomet Chem. 2022;36(1):e6458. doi:10.1002/aoc.v36.1. [Google Scholar] [CrossRef]
114. Emami F, Aliomrani M, Tangestaninejad S, Kazemian H, Moradi M, Rostami M. Copper-curcumin-bipyridine dicarboxylate complexes as anticancer candidates. Chem Biodivers. 2022;19(10):e202200202. doi:10.1002/cbdv.v19.10. [Google Scholar] [CrossRef]
115. Machado JF, Marques F, Pinheiro T, Villa de Brito MJ, Scalese G, Pérez-Díaz L, et al. Copper(I)-thiosemicarbazone complexes with dual anticancer and antiparasitic activity. ChemMedChem. 2023;18(14):e202300074. doi:10.1002/cmdc.v18.14. [Google Scholar] [CrossRef]
116. Garufi A, Scarpelli F, Ricciardi L, Aiello I, D’Orazi G, Crispini A. New copper-based metallodrugs with anti-invasive capacity. Biomolecules. 2023;13(10):1489. doi:10.3390/biom13101489. [Google Scholar] [PubMed] [CrossRef]
117. Moreno-Alcántar G, Picchetti P, Casini A. Gold complexes in anticancer therapy: from new design principles to particle-based delivery systems. Angew Chem Int Ed Engl. 2023;62(22):202218000. doi:10.1002/anie.v62.22. [Google Scholar] [CrossRef]
118. Sánchez Delgado GY, Arvellos JFA, Paschoal DFS, Dos Santos HF. Role of the enzymatic environment in the reactivity of the AuIII-C^N^C anticancer complexes. Inorg Chem. 2021;60(5):3181–95. doi:10.1021/acs.inorgchem.0c03521. [Google Scholar] [PubMed] [CrossRef]
119. Khan HA, Al-Hoshani A, Isab AA, Alhomida AS. A Gold(III) complex with potential anticancer properties. ChemistrySelect. 2022;7(45):e202202956. doi:10.1002/slct.v7.45. [Google Scholar] [CrossRef]
120. Yue S, Luo M, Liu H, Wei S. Recent advances of gold compounds in anticancer immunity. Front Chem. 2020;8:543. doi:10.3389/fchem.2020.00543. [Google Scholar] [PubMed] [CrossRef]
121. Zhang J, Li Y, Fang R, Wei W, Wang Y, Jin J, et al. Organometallic gold(I) and gold(III) complexes for lung cancer treatment. Front Pharmacol. 2022;13:979951. doi:10.3389/fphar.2022.979951. [Google Scholar] [PubMed] [CrossRef]
122. Yeo CI, Ooi KK, Tiekink ERT. Gold-based medicine: a paradigm shift in anti-cancer therapy? Molecules. 2018;23(6):1410. doi:10.3390/molecules23061410. [Google Scholar] [PubMed] [CrossRef]
123. Quero J, Ruighi F, Osada J, Gimeno MC, Cerrada E, Rodriguez-Yoldi MJ. Gold(I) complexes bearing alkylated 1,3,5-triaza-7-phosphaadamantane ligands as thermoresponsive anticancer agents in human colon cells. Biomedicines. 2021;9(12):1848. doi:10.3390/biomedicines9121848. [Google Scholar] [PubMed] [CrossRef]
124. Gil-Moles M, Concepción Gimeno M. The therapeutic potential in cancer of terpyridine-based metal complexes featuring group 11 elements. ChemMedChem. 2024;19(10):e202300645. doi:10.1002/cmdc.v19.10. [Google Scholar] [CrossRef]
125. Gukathasan S, Obisesan OA, Saryazdi S, Ratliff L, Parkin S, Grossman RB, et al. A conformationally restricted gold(III) complex elicits antiproliferative activity in cancer cells. Inorg Chem. 2023;62(32):13118–29. doi:10.1021/acs.inorgchem.3c02066. [Google Scholar] [PubMed] [CrossRef]
126. Sousa SA, Leitão JH, Silva RAL, Belo D, Santos IC, Guerreiro JF, et al. On the path to gold: monoanionic Au bisdithiolate complexes with antimicrobial and antitumor activities. J Inorg Biochem. 2020;202:110904. doi:10.1016/j.jinorgbio.2019.110904. [Google Scholar] [PubMed] [CrossRef]
127. Augello G, Azzolina A, Rossi F, Prencipe F, Mangiatordi GF, Saviano M, et al. New insights into the behavior of NHC-gold complexes in cancer cells. Pharmaceutics. 2023;15(2):466. doi:10.3390/pharmaceutics15020466. [Google Scholar] [PubMed] [CrossRef]
128. Kim JH, Ofori S, Parkin S, Vekaria H, Sullivan PG, Awuah SG. Anticancer gold(III)-bisphosphine complex alters the mitochondrial electron transport chain to induce: in vivo tumor inhibition. Chem Sci. 2021;12(21):7467–79. doi:10.1039/D1SC01418H. [Google Scholar] [PubMed] [CrossRef]
129. Jiang J, Cao B, Chen Y, Luo H, Xue J, Xiong X, et al. Alkylgold(III) complexes undergo unprecedented photo-induced β-hydride elimination and reduction for targeted cancer therapy. Angew Chem Int Ed Engl. 2022;61(16):e202201103. doi:10.1002/anie.v61.16. [Google Scholar] [CrossRef]
130. Giuso V, Yang J, Forté J, Dossmann H, Daniel C, Gourlaouen C, et al. Binuclear Biphenyl Organogold(III) complexes: synthesis, photophysical and theoretical investigation, and anticancer activity. Chempluschem. 2023;88(11):e202300303. doi:10.1002/cplu.v88.11. [Google Scholar] [CrossRef]
131. Fahmy HM, Mosleh AM, El-Sayed AA, El-Sherif AA. Novel palladium(II) and Zinc(II) schiff base complexes: synthesis, biophysical studies, and anticancer activity investigation. J Trace Elem Med Biol. 2023;79:127236. doi:10.1016/j.jtemb.2023.127236. [Google Scholar] [PubMed] [CrossRef]
132. Seddik RG, Shoukry AA, Rashidi FB, Salah-Eldin DS. Investigation on CT-DNA and protein interaction of new Pd(II) complexes involving ceftazidime and 3-Amino-1,2,3-triazole: synthesis, characterization, biological impact, anticancer evaluation, and molecular docking approaches. Chem Biodivers. 2023;20(12):e202301170. doi:10.1002/cbdv.v20.12. [Google Scholar] [CrossRef]
133. Feizi-Dehnayebi M, Dehghanian E, Mansouri-Torshizi H. Biological activity of bis-(morpholineacetato) palladium(II) complex: preparation, structural elucidation, cytotoxicity, DNA-/serum albumin-interaction, density functional theory, in-silico prediction and molecular modeling. Spectrochim Acta A Mol Biomol Spectrosc. 2022;281:121543. doi:10.1016/j.saa.2022.121543. [Google Scholar] [PubMed] [CrossRef]
134. Jovičić Milić SS, Jevtić VV, Radisavljević SR, Petrović BV, Radojević ID, Raković IR, et al. Synthesis, characterization, DNA interactions and biological activity of new palladium(II) complexes with some derivatives of 2-aminothiazoles. J Inorg Biochem. 2022;233:111857. doi:10.1016/j.jinorgbio.2022.111857. [Google Scholar] [PubMed] [CrossRef]
135. Liu Y, Li J, Chen M, Chen X, Zheng N. Palladium-based nanomaterials for cancer imaging and therapy. Theranostics. 2020;10(22):10057–74. doi:10.7150/thno.45990. [Google Scholar] [PubMed] [CrossRef]
136. Hosseini-Kharat M, Rahimi R, Alizadeh AM, Zargarian D, Khalighfard S, Mangin LP, et al. Cytotoxicity, anti-tumor effects and structure-activity relationships of nickel and palladium S,C,S pincer complexes against double and triple-positive and triple-negative breast cancer (TNBC) cells. Bioorg Med Chem Lett. 2021;43:128107. doi:10.1016/j.bmcl.2021.128107. [Google Scholar] [PubMed] [CrossRef]
137. Taskinen I, Jääskeläinen S, Koshevoy I, Hirva P. Palladium and platinum complexes of primary 2-pyridinethioamide. Solid State Sci. 2023;146:107374. doi:10.1016/j.solidstatesciences.2023.107374. [Google Scholar] [CrossRef]
138. Hosseini MJ, Jafarian I, Farahani S, Khodadadi R, Tagavi SH, Naserzadeh P, et al. New mechanistic approach of inorganic palladium toxicity: impairment in mitochondrial electron transfer. Metallomics. 2016;8(2):252–9. doi:10.1039/C5MT00249D. [Google Scholar] [PubMed] [CrossRef]
139. Nadeem S, Bolte M, Ahmad S, Fazeelat T, Tirmizi SA, Rauf MK, et al. Synthesis, crystal structures and, antibacterial and antiproliferative activities in vitro of palladium(II) complexes of triphenylphosphine and thioamides. Inorganica Chimica Acta. 2010;363(13):3261–9. doi:10.1016/j.ica.2010.06.015. [Google Scholar] [CrossRef]
140. Zhou XQ, Wang P, Ramu V, Zhang L, Jiang S, Li X, et al. In vivo metallophilic self-assembly of a light-activated anticancer drug. Nat Chem. 2023;15(7):980–7. doi:10.1038/s41557-023-01199-w. [Google Scholar] [PubMed] [CrossRef]
141. Ouyang R, Wang S, Feng K, Liu C, Silva DZ, Chen Y, et al. Potent saccharinate-containing palladium(II) complexes for sensitization to cancer therapy. J Inorg Biochem. 2023;244:112205. doi:10.1016/j.jinorgbio.2023.112205. [Google Scholar] [PubMed] [CrossRef]
142. Krstic A, Pavic A, Avdovic E, Markovic Z, Stevanovic M, Petrovic I. Coumarin-Palladium(II) complex acts as a potent and non-toxic anticancer agent against pancreatic carcinoma cells. Molecules. 2022;27(7):2115. doi:10.3390/molecules27072115. [Google Scholar] [PubMed] [CrossRef]
143. Schoofs H, Schmit J, Rink L. Zinc toxicity: understanding the limits. Molecules. 2024;29(13):3130. doi:10.3390/molecules29133130. [Google Scholar] [PubMed] [CrossRef]
144. Bilensoy E, Varan C. Is there a niche for zinc oxide nanoparticles in future drug discovery? Expert Opin Drug Discov. 2023;18(9):943–5. doi:10.1080/17460441.2023.2230152. [Google Scholar] [PubMed] [CrossRef]
145. Qu Z, Liu Q, Kong X, Wang X, Wang Z, Wang J, et al. A systematic study on zinc-related metabolism in breast cancer. Nutrients. 2023;15(7):1703. doi:10.3390/nu15071703. [Google Scholar] [PubMed] [CrossRef]
146. Wiesmann N, Tremel W, Brieger J. Zinc oxide nanoparticles for therapeutic purposes in cancer medicine. J Mater Chem B. 2020;8(23):4973–89. doi:10.1039/D0TB00739K. [Google Scholar] [PubMed] [CrossRef]
147. Liang JY, Liu YY, Zou J, Franklin RB, Costello LC, Feng P. Inhibitory effect of zinc on human prostatic carcinoma cell growth. Prostate. 1999;40(3):200–7. doi:10.1002/(ISSN)1097-0045. [Google Scholar] [CrossRef]
148. Li D, Stovall DB, Wang W, Sui G. Advances of zinc signaling studies in prostate cancer. Int J Mol Sci. 2020;21(2):667. doi:10.3390/ijms21020667. [Google Scholar] [PubMed] [CrossRef]
149. Zhang P, Li Y, Tang X, Guo R, Li J, Chen Y, et al. Zinc enhances chemosensitivity to paclitaxel in PC-3 prostate cancer cells. Oncol Rep. 2018;40:2269–77. doi:10.3892/or.2018.6622. [Google Scholar] [PubMed] [CrossRef]
150. Xue YN, Yu BB, Liu YN, Guo R, Li JL, Zhang LC, et al. Zinc promotes prostate cancer cell chemosensitivity to paclitaxel by inhibiting epithelial-mesenchymal transition and inducing apoptosis. Prostate. 2019;79(6):647–56. doi:10.1002/pros.23772. [Google Scholar] [PubMed] [CrossRef]
151. George BP, Rajendran NK, Houreld NN, Abrahamse H. Rubus capped zinc oxide nanoparticles induce apoptosis in MCF-7 breast cancer cells. Molecules. 2022;27(20):6862. doi:10.3390/molecules27206862. [Google Scholar] [PubMed] [CrossRef]
152. Mohamed SY, Elshoky HA, El-Sayed NM, Fahmy HM, Ali MA. Ameliorative effect of zinc oxide-chitosan conjugates on the anticancer activity of cisplatin: approach for breast cancer treatment. Int J Biol Macromol. 2024;257:128597. doi:10.1016/j.ijbiomac.2023.128597. [Google Scholar] [PubMed] [CrossRef]
153. Alallam B, Doolaanea AA, Alfatama M, Lim V. Phytofabrication and characterisation of zinc oxide nanoparticles using pure curcumin. Pharmaceuticals. 2023;16(2):269. doi:10.3390/ph16020269. [Google Scholar] [PubMed] [CrossRef]
154. Li G, Chen J, Xie Y, Yang Y, Niu Y, Chen X, et al. White light increases anticancer effectiveness of iridium(III) complexes toward lung cancer A549 cells. J Inorg Biochem. 2024;259:112652. doi:10.1016/j.jinorgbio.2024.112652. [Google Scholar] [PubMed] [CrossRef]
155. Lv A, Li G, Zhang P, Tao R, Li X, Ren X, et al. Design and anticancer behaviour of cationic/neutral half-sandwich iridium (III) imidazole-phenanthroline/phenanthrene complexes. J Inorg Biochem. 2024;257:112612. doi:10.1016/j.jinorgbio.2024.112612. [Google Scholar] [PubMed] [CrossRef]
156. Kasparkova J, Novohradsky V, Ruiz J, Brabec V. Photoactivatable, mitochondria targeting dppz iridium (III) complex selectively interacts and damages mitochondrial DNA in cancer cells. Chem Biol Interact. 2024;392:110921. doi:10.1016/j.cbi.2024.110921. [Google Scholar] [PubMed] [CrossRef]
157. Hu H, Zhang F, Sheng Z, Tian S, Li G, Tang S, et al. Synthesis and mitochondria-localized iridium (III) complexes induce cell death through pyroptosis and ferroptosis pathways. Eur J Med Chem. 2024;268:116295. doi:10.1016/j.ejmech.2024.116295. [Google Scholar] [PubMed] [CrossRef]
158. Chen L, Tang H, Chen W, Wang J, Zhang S, Gao J, et al. Mitochondria-targeted cyclometalated iridium (III) complexes: dual induction of A549 cells apoptosis and autophagy. J Inorg Biochem. 2023;249:112397. doi:10.1016/j.jinorgbio.2023.112397. [Google Scholar] [PubMed] [CrossRef]
159. Yang C, Deng X, Tang Y, Tang H, Xia C. Natural products reverse cisplatin resistance in the hypoxic tumor microenvironment. Cancer Lett. 2024;598:217116. doi:10.1016/j.canlet.2024.217116. [Google Scholar] [PubMed] [CrossRef]
160. Cheng Y, Fan Y, Zhao Y, Huang D, Li X, Zhang P, et al. Tislelizumab plus platinum and etoposide versus placebo plus platinum and etoposide as first-line treatment for extensive-stage SCLC (RATIONALE-312a multicenter, double-blind, placebo-controlled, randomized, phase 3 clinical trial. J Thorac Oncol. 2024;19(7):1073–85. doi:10.1016/j.jtho.2024.03.008. [Google Scholar] [PubMed] [CrossRef]
161. Reck M, Barlesi F, Yang JC, Westeel V, Felip E, Özgüroğlu M, et al. Avelumab versus platinum-based doublet chemotherapy as first-line treatment for patients with high-expression programmed death-Ligand 1-positive metastatic NSCLC: primary analysis from the phase 3 JAVELIN Lung 100 trial. J Thorac Oncol. 2024;19(2):297–313. doi:10.1016/j.jtho.2023.09.1445. [Google Scholar] [PubMed] [CrossRef]
162. Mok T, Nakagawa K, Park K, Ohe Y, Girard N, Kim HR, et al. Nivolumab plus chemotherapy in epidermal growth factor receptor-mutated metastatic non-small-cell lung cancer after disease progression on epidermal growth factor receptor tyrosine kinase inhibitors: final results of CheckMate 722. J Clin Oncol. 2024;42(11):1252–64. doi:10.1200/JCO.23.01017. [Google Scholar] [PubMed] [CrossRef]
163. Chen M, Zhang H, Cui WX, Chen MY, Cheng XP. The effect of selenium yeast in the prevention of adverse reactions related to platinum-based combination therapy in patients with malignant tumors. Eur Rev Med Pharmacol Sci. 2023;27(21):10499–506. [Google Scholar] [PubMed]
164. Koeneman BJ, Schreibelt G, Gorris MAJ, Hins-de Bree S, Westdorp H, Ottevanger PB, et al. Dendritic cell vaccination combined with carboplatin/paclitaxel for metastatic endometrial cancer patients: results of a phase I/II trial. Front Immunol. 2024;15:1368103. doi:10.3389/fimmu.2024.1368103. [Google Scholar] [PubMed] [CrossRef]
165. Khanmammadov NJ, Doğan İ., Okay NS, Azizy A, Saip P, Aydiner A. Comparative analysis of doublet chemotherapy regimens plus bevacizumab in patients with recurrent ovarian cancer. Medicine. 2024;103(1):e36750. doi:10.1097/MD.0000000000036750. [Google Scholar] [PubMed] [CrossRef]
166. Leijen S, Burgers SA, Baas P, Pluim D, Tibben M, Van Werkhoven E, et al. Phase I/II study with ruthenium compound NAMI-A and gemcitabine in patients with non-small cell lung cancer after first line therapy. Invest New Drugs. 2015;33(1):201–14. doi:10.1007/s10637-014-0179-1. [Google Scholar] [PubMed] [CrossRef]
167. Lentz F, Drescher A, Lindauer A, Henke M, Hilger RA, Hartinger CG, et al. Pharmacokinetics of a novel anticancer ruthenium complex (KP1019, FFC14A) in a phase I dose-escalation study. Anticancer Drugs. 2009;20(2):97–103. doi:10.1097/CAD.0b013e328322fbc5. [Google Scholar] [PubMed] [CrossRef]
168. Kulkarni GS, Lilge L, Nesbitt M, Dumoulin-White RJ, Mandel A, Jewett MAS. A phase 1b clinical study of intravesical photodynamic therapy in patients with Bacillus Calmette-Guérin-unresponsive non-muscle-invasive bladder cancer. Eur Urol Open Sci. 2022;41:105–11. doi:10.1016/j.euros.2022.04.015. [Google Scholar] [PubMed] [CrossRef]
Cite This Article
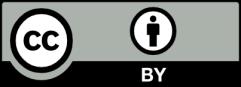
This work is licensed under a Creative Commons Attribution 4.0 International License , which permits unrestricted use, distribution, and reproduction in any medium, provided the original work is properly cited.